Abstract
Plants play an essential role in methane (CH4) production, transport and release processes of constructed wetlands but as yet there has been no consistent and clear consensus of their impacts on CH4 emissions. In this study, we used plant presence, species richness, plant species-specificity, and harvesting activity information obtained by reviewing papers published from 1993 to 2018 to elucidate the key factors that drive CH4 emission from constructed wetlands. Although it was not statistically significant, plant presence increased the CH4 emissions compared to unvegetated conditions and relatively lower values were observed for constructed wetlands planted with Acorus calamus, Cyperus papyrus or Juncus effusus. The use of a single plant species not only changed the production and consumption of CH4 by affecting the functioning of roots but also influenced the process of CH4 entering the atmosphere under different transport capacities. The CH4 flux reached 1.0686 g CH4 m−2 d−1 from the Zizania latifolia system, which is eight times larger than that of the Phalaris arundinacea system. The mixed systems exhibited a positive increase in CH4 flux with plant species richness due to the complementary effects of the root exudates excreted from different plants. The minimum CH4 value (−0.0084 g CH4 m−2 d−1) was observed in the three-species system (Oenanthe javanica, Phalaris arundinacea and J. effusus). These results demonstrate that selecting several species with lower methane fluxes such as Typha latifolia and C. papyrus and suitably regulating harvesting in constructed wetlands can be more effective for mitigating the potential of CH4 emissions while maintaining the efficiency of sewage purification.
Introduction
Constructed wetlands that are easy to operate and maintain are an ideal alternative to typical wastewater treatment technologies (Garcia et al. Citation2010; Mitsch et al. Citation2014). They have been used increasingly for treating various types of wastewater, such as municipal, domestic, and agricultural wastewater (Wu et al. Citation2015). The number of constructed wetlands in China has exponentially increased from less than 10 to over 400 in the last two decades (Zhang et al. Citation2012a; Li et al. Citation2018). Meanwhile, previous studies have demonstrated that the areas converted to wetlands will likely result in dramatic increases in CH4 emissions (Johansson et al. Citation2004). Hence, the large increase in constructed wetland areas has raised environmental concerns owing to the potential increase in the emissions of CH4, despite their potential ability to control pollutants (Xue et al. Citation1999; Niu et al. Citation2015).
According to some previous studies, the surface dry air molar fraction of atmospheric CH4 in 2012 reached 1810 ppb, which is 2.5 times larger than it was in 1750 (Kirschke et al. Citation2013). Owing to the sustained rise in atmospheric CH4 levels, CH4 has gradually become the second most important anthropogenic greenhouse gas after CO2 (Nouchi et al. Citation1994; Saunois et al. Citation2016). A large number of experimental papers on CH4 fluxes in constructed wetlands have been published, indicating that the wastewater treatment process plays an extremely important role in the various CH4 sources (IPCC Citation2014).
During the sewage purification process in constructed wetlands, plants are one of the most significant factors affecting CH4 emission because of their roots and stem systems (Segers Citation1998; Ström et al. Citation2005; Wang et al. Citation2008; Lai et al. Citation2011; Mander et al. Citation2014) that release oxygen and produce root exudates (Jackson and Armstrong Citation1999; Saarnio et al. Citation2004). CH4 fluxes are determined by the balance between the formation and consumption of CH4 (Wang et al. Citation2013a). The most frequently used plant in constructed wetlands worldwide is Phragmites australis, while Typha latifolia, Juncus effusus, Phalaris arundinacea, and Zizania latifolia are also commonly used. However, there have been no uniform results regarding the effect of plants on CH4 flux.
Phragmites australis is the most frequently used macrophyte in constructed wetlands, as it can form a monoculture and significantly modify the structure and functions of wetland ecosystems (McCormick et al. Citation2010; Uddin et al. Citation2017). The aboveground parts of P. australis die back at the end of the annual growth cycle but the underground parts remain viable for many years. Its physiological characteristics including high reproductive rate, well-developed aerated tissue, a rhizosphere that promotes colonization, and dense canopy associated with high productivity that can inhibit germination and growth of other plant species (Meyerson et al. Citation2000; Mozdzer and Zieman Citation2010; Guo et al. Citation2013). Greater biomass and different decomposition rates can cause changes in the CH4 flux in P. australis-based constructed wetlands.
Zizania latifolia is a well-known wild rice grass. As a perennial, herbaceous aquatic plant (Wang et al. Citation2014), it commonly grows to 1.5–3.0 m with well-developed fibrous roots usually in swampy regions and wetlands, normally alongside some emergent plants, such as T. angustifolia and P. australis.
Typha latifolia (commonly called broadleaf cattail) is a well-known aquatic wetland plant that grows widely in tropical and warm regions, including Asia, Africa, the Americas, and Europe (Ye et al. Citation1997; Ahmad et al. Citation2017). This species can expand to areas that were previously occupied by other species owing to their size, adaptability, and root exudates (Gallardo et al. Citation1998). Typha latifolia can quickly inhabit poor environments and has strong environmental adaptability (He et al. Citation2015).
Juncus effusus, which has cylindrical photosynthetic stems filled with a spongy pith, is widely used in sewage treatment and the stimulation of microbial activity (Moran and Hodson Citation1989; Garnet et al. Citation2005). It has since been established as a model plant in basic and applied research on wetland ecosystems.
Phalaris arundinacea is, a 1–3 m-high perennial herb that produces dense crowns and forms a strong root system that penetrates to depths of approximately 30–40 cm and spreads aggressively by asexual reproduction (Lewandowski et al. Citation2003). It is generally best suited for growth under cool and moist conditions, tending to form a single cultivar by inhibiting the growth of other plants (Lavergne and Molofsky Citation2004; Adams and Galatowitsch Citation2005). Studies have reported that P. arundinacea has more developed aboveground parts relative to its roots under enhanced pollutant treatment (Maurer and Zedler Citation2002).
The presence of aquatic plants is one of the most significant features of wetlands, and it distinguishes constructed wetlands from unvegetated traditional sewage treatment methods (Vymazal Citation2011). Many researchers have studied several plant factors that affect CH4 fluxes in constructed wetlands (Wang et al. Citation2008; Zhang et al. Citation2012b; Mander et al. Citation2014). Accordingly, to explore the plant factors that drive CH4 fluxes, we reviewed articles published from 1993 to 2018 to investigate the fluxes in various constructed wetlands by comparing plant conditions. Does the presence of plants inhibit methane emissions? Does the species richness facilitate methane emissions? Does the selected species increase the ecological effects of wetlands? Does harvesting reduce methane emissions?
Methods
Data collection
A literature survey of peer-reviewed publications published in the last 25 years (1993-2018) on constructed wetlands and CH4 flux was conducted. The terms ‘(aquatic) plant/macrophyte’, ‘methane (CH4)’, ‘methanogens’, and ‘methanotrophs’, in combination with the terms ‘(artificial/constructed/treatment) wetland’ were searched using ISI-Web of Science. We selected papers that focused on the factors that were most likely to affect CH4 flux, including (1) plant presence, (2) plant species, (3) plant species richness, and (4) plant harvesting. After manually eliminating less relevant works from 317 articles, 129 remaining papers were selected for further investigation. Data were collected from 62 studies conducted on 23 constructed wetlands from 15 countries that discussed plants as a prominent factor affecting CH4 flux in constructed wetlands (). Some of the data were excluded as they were greatly affected by other factors, such as water level, season, and peatland.
Data analysis
The collected data were divided into two categories according to the source of water (sewage and modified Hoagland nutrient solution; Hoagland and Arnon Citation1950). The Hoagland nutrient solution can meet the trace element needs of the plant growth process while preventing its influence on the determination of root exudates (). In sewage-based constructed wetlands, the data were more likely to show the effects of plant species on CH4 fluxes, while the other data with the modified Hoagland nutrient solution were more likely to indicate the effects of plant species richness on CH4 fluxes. Furthermore, there are seasonal and daily variations in CH4 fluxes. We mainly selected data for similar seasons and took the average to minimize the error. All CH4 flux data were expressed using Origin 9.0.
Table 1. Nutrient composition of the modifying Hoagland nutrient solution used in the constructed wetland microcosms.
An independent samples t-test was conducted to determine the differences between the vegetated and unvegetated systems using SPSS Statistics 20 (SPSS Inc., Chicago, USA). One-way analysis of variance (ANOVA) was also conducted to test the differences between the mean values when different species were present in the communities. The statistical significance level was set at α = 0.05.
Results
The number of papers published from 1993 to 2018 on CH4 fluxes in constructed wetlands increased every 5-year period (). In particular, in recent years, the number of relevant studies is almost at a high level. Most data about CH4 emissions were obtained from laboratory and actual constructed wetlands field studies published in the last 10 years. The planting density of seedlings with similar sizes benefitting their survival was 9–16 individuals per microcosm. From analyzing the data from 62 papers of the 317 studies, 20 plant species were recorded. The most common plants used in constructed wetlands are P. australis (35.29%), T. latifolia (17.65%), J. effusus (8.82%), P. arundinacea (7.84%) and Z. latifolia (5.88%) ().
Figure 2. (a) The number of published papers from 1993 to 2018 in regard to CH4 flux from constructed wetlands; (b) The proportion of commonly used plants in constructed wetlands. Others include Acorus calamus, Carex crinita, Canna indica, Chrysantemum segetum, Iris pseudacorus, Lythrum salicaria, Scheuchzeria palustris, Schoenoplectus validus, Scirpus cyperinus.
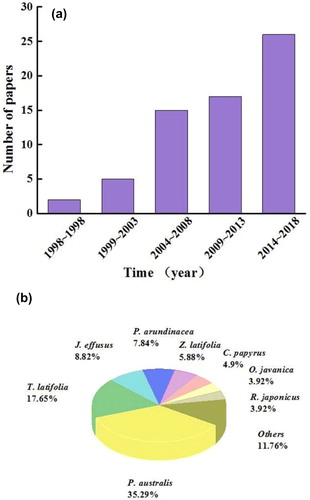
Plant presence
The methane flux did not exhibit a statistically significant difference, although the flux was higher for vegetated systems (mean of 0.4243 g CH4 m−2 d−1) than that of unvegetated systems (mean of 0.2549 g CH4 m−2 d−1) with sewage (p = 0.089). The range of CH4 emissions from vegetated systems (0.0236 to 2.208 g CH4 m−2 d−1) was larger than that from unvegetated systems (−0.0048 to 0.9192 g CH4 m−2 d−1) when treating sewage (). The highest CH4 emission value was observed in the Z. latifolia system, while relatively lower values were observed in constructed wetlands planted with Acorus calamus, C. papyrus, or J. effusus. As shown in , the methane flux was also similar between vegetated systems (mean of 0.0046 g CH4 m−2 d−1) and unvegetated systems (mean of 0.0036 g CH4 m−2 d−1) with the modified Hoagland nutrient pollution, as indicated by analyzing the data (p = 0.831). The maximum and minimum fluxes were recorded in a polyculture system (Rumex japonicas, Oenanthe hookeri, P. arundinacea and J. effusus) and a monoculture (J. effusus) system, respectively.
The effects of species-specific relationships on CH4 flux
In our study, significant differences were observed between different plant species in sewage systems (F = 2.99, p = 0.004, ). The highest CH4 emission values were observed for Z. latifolia constructed wetlands (mean of 1.069 g CH4 m−2 d−1), followed by J. effusus and P. australis (means of 0.528 and 0.523 g CH4 m−2 d−1, respectively). Compared to unvegetated systems, the systems planted with vascular plants, such as P. arundinacea, C. papyrus, or T. latifolia, exhibited lower CH4 fluxes into the atmosphere. These species are suitable for mitigating the greenhouse effect in the operation of constructed wetlands. Meanwhile, the CH4 flux (ranging from 0.0024 to 0.0065 g CH4 m−2 d−1) did not differ significantly between different plant species (F = 1.652, p = 0.182), but the relatively low CH4 fluxes of approximately 0.0024 g CH4 m−2 d−1 were observed in P. arundinacea systems with the modified Hoagland nutrient solution (). The maximum value was only 0.0089 g CH4 m−2 d−1. This may be because the effect of transportation on CH4 emissions through the aerenchyma of plants is not fully exerted with low concentrations of biodegradable organic matter in wastewater.
Figure 4. CH4 flux from constructed wetlands with different species (a) sewage. Others include Arundo donax, Canna indica, Glyceria maxima, Mischantus giganteus; (b) modified Hoagland nutrient solution. Boxes show median and interquartile range. Outliers are identified as points outside 1.5 times the interquartile range.
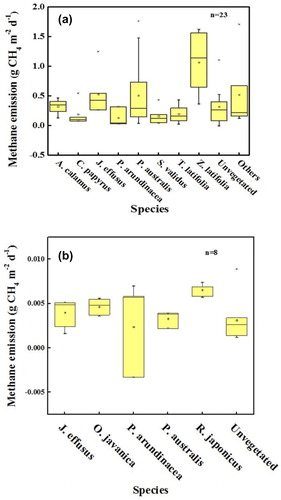
Species richness
The number of species in a plant community is referred to as the species richness. In this study, the CH4 flux increased with species richness during wastewater treatment in constructed wetlands, thus confirming that species richness was a factor affecting CH4 emissions. Regardless of the magnitude of methane emissions, the overall trend in methane flux is increasing with species richness. When the inflow is the modified Hoagland nutrient solution, the increasing CH4 flux value may be small, but the increasing trend with plant species richness is clear (). Although, plant species richness had almost no effect on CH4 emissions when the water had high nitrogen levels. It was noteworthy that the minimum methane value (−0.0084 g CH4 m−2 d−1) was observed in a three-species system (O. javanica, P. arundinacea, and J. effusus). However, limited relevant experimental studies on sewage-based constructed wetlands indicated that a high species richness was beneficial for CH4 emissions. In the polyculture system, the maximum CH4 emission value reached 5.88 g CH4 m−2 d−1, which is about 2.27 times larger than that in the monoculture system (2.592 g CH4 m−2 d−1).
Plant harvest on CH4 emission
The production and consumption of CH4 in wetlands are affected by the growth stage of plants and the net primary productivity of the ecosystem (Whiting and Chanton Citation1993; Hanson and Hanson Citation1996). The CH4 flux was influenced remarkably by plant harvesting, and it occurred immediately after the plants in the constructed wetlands were harvested (CH4 emissions were reduced by a factor of 12). The CH4 emissions were found to recover 0.0008 g CH4 m−2 d−1.
Discussion
Methane appears to be the most important GHG emitted from constructed wetlands (Maltais-Landry et al. Citation2009; Mander et al. Citation2014). The flux of CH4 is affected by various factors including the age of constructed wetlands (Liikanen et al. Citation2006; Zemanová et al. Citation2010; de la Varga et al. Citation2015), wastewater flow (Liu et al. Citation2009) and quality (Yan et al. Citation2012), feeding schemes (Jia et al. Citation2011) and environmental conditions (Liikanen et al. Citation2006). Meanwhile, a more detailed biogeochemical cycle can be learned from Megonigal's article (Megonigal and Neubauer Citation2019). Special attention should be paid to the plant species used in constructed wetlands, which heavily affect the CH4 flux from constructed wetlands by influencing microbial activity (Inamori et al. Citation2007). In general, the methane emitted to the atmosphere experiences the three processes of production, consumption and transport (). For production, plants provide an appropriate root surface and exudates for the growth and activity of microorganisms (Zhai et al. Citation2013), promoting the conversion of organic matter to CH4 (anoxic zone). Subsequently, the amount of oxygen released by root systems into the sediment would enhance the number of methanotrophs as they favor the aerobic rhizosphere environment. The transport process in the emission of CH4 is also crucial for the contribution of the quantity of atmospheric CH4. The CH4 flux emitted to the atmosphere in vascular plant-dominated constructed wetlands is primarily driven by three processes including ebullition and diffusion, and plant emissions (Brix et al. Citation2001). However, more CH4 would be oxidized in the first two processes than in plant-mediated transport through the aerenchyma resulting in a large CH4 flux from planted constructed wetlands. Furthermore, both electron donors and acceptors play important roles in regulating anaerobic microbial mineralization of soil organic matter (Sutton-Grier et al. Citation2011). The final CH4 flux in vegetated or unvegetated constructed wetlands is determined by the combined effects of plants on CH4 production, transport, and consumption. The effect of plants on CH4 emission is remarkable, and requires further study. Therefore, we reviewed published literature to evaluate the effects of different plant species on CH4 emissions.
Plant presence
The role of plants in constructed wetlands is crucial (Vymazal Citation2011). Most studies clearly demonstrated that constructed wetlands with plants have better pollutant removal efficiencies than those without (Vymazal Citation2011) and they have a significant effect on CH4 fluxes during the pollutant removal process (Henneberg et al. Citation2016). Although there was no significant difference between vegetated and unvegetated constructed wetlands, vegetated systems exhibited a higher average CH4 flux in vegetated systems than that of unvegetated systems. Previous studies support this trend, demonstrating that the presence of plants increases CH4 fluxes in constructed wetlands (Wild et al. Citation2001; Ström et al. Citation2007). McInerney and Helton (Citation2016) observed higher CH4 emissions from vegetated constructed wetlands by comparing the presence and absence of emergent herbaceous vegetation (T. angustifolia and T. latifolia). Recently, Wu et al. (Citation2017) continuously measured the flux of CH4 from free-water surface-constructed wetlands in northern China following the static-stationary chamber technique from 2012 to 2013, and observed higher CH4 emissions from planted wetlands than those from unplanted systems. Similarly, Wang et al. (Citation2013a) found that the CH4 flux of vegetated systems (1.6752 g CH4 m−2 d−1) is much higher than that of unvegetated systems (0.276 g CH4 m−2 d−1). Similar results were also obtained by Niu et al. (Citation2015), who reported that a vegetated system achieved a higher CH4 flux rate than an unvegetated system. Therefore, the presence of plants would boost the CH4 flux while increasing the pollutant removal efficiency. A greater amount of methanogens are found in vegetated constructed wetlands as plants provide a more beneficial environment for their growth and reproduction, such as their large root surfaces and carbon sources (Zhai et al. Citation2013), resulting in a high level of methane production. The aerenchyma contained inside plants facilitates the transport of methane from soils or water to the atmosphere (Terazewa et al. Citation2007; Rice et al. Citation2010). It has been reported that up to 95% of the CH4 emissions of vegetated wetlands may be transported through the aerenchyma (Schütz et al. Citation1989; Grünfeld and Brix Citation1999; Bhullar et al. Citation2013), and this pathway can reduce both the ebullition and diffusion of CH4 through the soil column (Sorrell and Boon Citation1994; Schimel Citation1995). Although the roots may modify the soil oxidation-reduction potential due to the release of oxygen, which may mitigate the CH4 flux, it was not decisive. Only a few studies stated that unplanted conditions have a higher CH4 flux than planted systems (Grünfeld and Brix Citation1999; Zhu et al. Citation2007; Maltais-Landry et al. Citation2009; Bateganya et al. Citation2015). Bateganya et al. (Citation2015) observed higher CH4 emissions from unplanted beds (0.624 g CH4 m−2 d−1) than that from planted beds (C. papyrus) (0.312 g CH4 m−2 d−1) for both vertical and horizontal subsurface flow constructed wetlands. However, de la Varga et al. (Citation2015) and Maucieri et al. (Citation2014) did not observe any significant differences in CH4 emissions between vegetated and unvegetated constructed wetlands. This might be due to the greater soil oxidation-reduction potential status in certain constructed wetlands, which inhibits methane emissions. Artificial aeration, the construction of intermittent vertical subsurface flow constructed wetlands, or wetland plants with strong oxygen capacity can all achieve this (Maltais-Landry et al. Citation2009; Bateganya et al. Citation2015). The abundant methanotrophics bacteria inhabiting aerobic regions can promote the oxidation of the large quantities of produced CH4 (Schütz et al. Citation1989; Denier vanderGon and Neue Citation1996).
In summary, the flux of CH4 from constructed wetlands is positively related to the presence of plants in most case. The main reason for this is that CH4 emission conditions are optimized by the physiological functions of plants in constructed wetlands.
The effects of plant species on CH4 flux
Most recent studies focused on the influence of plant species on CH4 flux (Barbera et al. Citation2015; Cao et al. Citation2017). Aquatic macrophytes can have different anatomical and physiological properties (Zhang et al. Citation2011), and their diversity has a remarkable effect on the CH4 flux. Owing to the ability of plants to raise the sediment redox potential, the release of root exudate and transport capacity correlate with the ability of the macrophytes to influence the CH4 flux (van der Nat and Middelburg Citation1998; Wang et al. Citation2008; Duan et al. Citation2009; Wang et al. Citation2013a; Girkin et al. Citation2018). Until now, significant differences in CH4 emissions were observed between constructed wetlands with different plant species. Considering the difference in plant species, such as P. arundinacea and Z. latifolia, their degree of influence in methane flux would be different. Markedly higher CH4 emissions from Z. latifolia constructed wetland than those from P. arundinacea wetlands have been observed. The plant species determines the release of root exudates and oxygen, and the capacity of transport, which indirectly highlights the specific effects of plant species on the CH4 flux due to the variation in the biogeochemical processes involved in organic matter degradation (Brix et al. Citation1992; Maltais-Landry et al. Citation2009). Most researchers have studied the plant species-specific effects on methane emissions and have monitored the differences in methane emission fluxes of constructed wetlands with different plants, such as Z. latifolia, P. australis and T. latifolia (Inamori et al. Citation2007; Ström et al. Citation2007; Wang et al. Citation2008; Barbera et al. Citation2015; Wu et al. Citation2017).
Understanding plant physiology might be useful in estimating CH4 emissions, as CH4 emissions vary between plant species and functional traits. For example, P. australis has an extensive rhizome system that usually penetrates to depths of approximately 0.6–1.0 m, and rigid stems with hollow internodes. Consequently, oxidation-reduction potential values of P. australis systems are higher than those of Z. latifolia systems at the same BOD concentration. This may explain the lower methane emissions observed from P. australis systems (Inamori et al. Citation2007). In this article, CH4 emissions were found to be significantly higher from Z. latifolia than those from some other species, which a potential benefit of using some monospecific stands for constructed wetlands. Furthermore, P. arundinacea, C. papyrus, or T. latifolia might be the best choice for reducing the CH4 flux during the sewage treatment process of constructed wetlands.
The authors have suggested that oxygen transfer, CH4 transfer, and root exudate release capacities vary between plant species, confirming the species-specific effects on CH4 emissions (Inamori et al. Citation2007; Ström et al. Citation2007; Wang et al. Citation2008; Barbera et al. Citation2015; Wu et al. Citation2017). Therefore, selecting an adequate plant species can modify the microbial processes of constructed wetlands and change (promote or inhibit) the CH4 formation, oxidation, and transport processes, subsequently changing the level of CH4 emissions (Wang et al. Citation2008). Remarkably, the rhizosphere is a key zone for CH4 formation and oxidation as it determines the quantity and activity of methanogens and methanotrophs during pollutant removal in constructed wetlands. Roots release a degradable substrate as a carbon source that would increase the number of methanogens and methanotrophs (Wang et al. Citation2013b). For example, Inamori et al. (Citation2007) found that 90% of the root biomass was concentrated in the top 10 cm of the substrate in vertical subsurface flow constructed wetlands vegetated with Z. latifolia, while the root biomass extends deeper and is evenly distributed along the substrate profile in P. australis systems. Meanwhile, it has been reported that methanotrophs are mainly distributed in the first 10 cm and then decrease along the depth of the rhizosphere in Z. latifolia systems. However, P. australis systems showed that bacteria are mainly distributed in the deeper layers (20 and 30 cm) rather than the first 10 cm due to the effects of activities involving plant roots. However, plant species that have a high biomass and dense root system may exude more organic matter, promoting the growth and reproduction of CH4-oxidizing bacteria and methanogens (Wang et al. Citation2013a). For CH4 production, the quantity of organic matter determined the growth of microbial bacteria, rather than the chemical characteristics of the substrate, which are similar between different plants. Therefore, the species-specific effects of plants are an important factor causing the CH4 flux to vary. The release of oxygen by plant root systems creates an aerobic microsite favored by methanotrophs that grow in the process of CH4 consumption (Wang et al. Citation2008). Many studies suggested that oxygen release is greater from some plants that have a higher above-ground biomass, such as Phragmites sp., than that of Typha sp. and Iris sp. (Wießner et al. Citation2002; Wang et al. Citation2009). However, the aerenchyma of a plant not only can play a significant role in the transportation of CH4 from wetlands to the atmosphere, but it also enables the transportation of oxygen from the air to the underground parts of the plants (Chanton et al. Citation1993; Rusch and Rennenberg Citation1998; Laanbroek Citation2010). For example, increased oxygen input from plants with greater biomass can offset any potential stimulation of methanogenic microbes from additional carbon inputs. Because the species composition of plant communities influences both electron donor and acceptor availability in wetland soils, changes in plant species as a consequence of anthropogenic disturbance have the potential to trigger profound effects on microbial processes, including changes in anaerobic decomposition rates and the proportion of mineralized carbon emitted as the greenhouse gas methane (Sutton-Grier and Megonigal Citation2011). Therefore, the differences in the transport function between different plant species in the CH4 emission process are noteworthy. In summary, through reviewing associated papers, the structure and physiological function of different plant species were found to be closely related to CH4 emissions.
Plant species richness
Hybrid plants generally elevate the CH4 flux to a certain extent, although the effect is small at high nitrogen levels. Our finding was most likely attributed to the complementary effect of the root exudates excreted from the different plants in polyculture systems. Zhang et al. (Citation2012b) found that the CH4 flux increased significantly with an increase in the plant species richness (O. hookeri, R. japonicus, P. arundinacea and Reineckia carnea). Wang et al. (Citation2013a), compared a Z. latifolia monoculture system with a polyculture planted with Z. latifolia, P. australis and T. latifolia, and observed a higher CH4 flux in the polyculture system (mean of 2.208 g CH4 m−2 d−1) than that in the monoculture system (mean of 1.1424 g CH4 m−2 d−1), with the lowest emissions in the senescence period and highest emissions during growth season. In the same year, Wang et al. (Citation2013b) concluded that CH4 emissions could be stimulated in polyculture systems. The results reported by Niu et al. (Citation2015) agreed with those of the previously mentioned study, indicating that a higher CH4 flux was observed in the mixed system (R. japonicus, O hookeri, P. arundinacea and J. effusus) than that in the monoculture due to the higher abundance of methanogens and lower number of methanotrophs.
In polyculture systems, the plant biomass increases with the plant species richness due to the complementary use of plants for nutrients in diverse species communities (van Ruijven and Berendse Citation2003; Zhang et al. Citation2011). The plant biomass increased with the plant species richness in these microcosms, which further lead to an increase in microbial biomass. Furthermore, the increased microbial biomass promoted the degradation of organic matter around the rhizosphere (Zhang et al. Citation2012a, Citationb; Zhao et al. Citation2016a,Citationb). Therefore, a higher number of methanogens and lower number of methanotrophs were observed in the rhizosphere regions of polyculture systems, resulting in large contributions to CH4 generation. Moreover, the more-developed aeration tissue increased the emission of CH4 with increasing plant species richness.
Han et al. (Citation2017) used four common local plant species (J. effusus, O. javanica, P. arundinacea and R. japonicus) in a microcosm experiment and found that plant species richness had no effect on CH4 flux. Furthermore, Mo et al. (Citation2015) and Zhao et al. (Citation2016a) also investigated the effect of plant species richness on the CH4 flux in constructed wetlands, and observed no significant relationship between plant species richness and CH4 flux. This is most likely due to the weak complementarity of some plant species, resulting in little effects on the CH4 flux.
So far, most researchers have observed a positive correlation between CH4 flux and plant species richness (Zhang et al. Citation2012b; Niu et al. Citation2015), while a few studies reported that CH4 flux was not affected by species richness in constructed wetlands (Zhao et al. Citation2016a; Han et al. Citation2017). This might be due to the diversity of plants during the experiment, which changed the quantity and activity of microbes by affecting the conditions in the constructed wetlands (Wang et al. Citation2012). However, different environmental factors, such as temperature, oxidation-reduction potential, ratio of C/N and water level, also played roles in the relationship between plant species richness and CH4 flux (Henneberg et al. Citation2016). Under these scenarios, the quantities of microbial bacteria were affected, resulting in different CH4 flux levels, and the effect of plant species richness on CH4 flux was greatly weakened.
The influence of plant harvesting
Although the physiological and structural characteristics of plants significantly affected the CH4 flux, the effect of plant harvesting on CH4 emissions from wetlands to the atmosphere cannot be ignored. Zhu et al. (Citation2007) found that CH4 emissions increased immediately after cutting P. communis in both free water-surface and horizontal subsurface-flow systems, which is likely due to the rapid release of the CH4 retained in the vascular systems of plant stems. After a few days, the CH4 flux from constructed wetlands was still higher in plant-harvested regions than un-cut zones as the stems above the water surface still served as conduits for constructed wetlands bed gases until the stem butts died. In agreement, higher CH4 emissions from horizontal subsurface flow constructed wetlands vegetated with M. giganteus were observed after plant cutting by Barbera et al. (Citation2015), and from horizontal and vertical subsurface flow constructed wetlands vegetated with P. australis and Arundo donax by Maucieri et al. (Citation2016).
After harvesting the plants, the CH4 stored in their stems releases to the atmosphere within a short period of time, and the transport of CH4 through the aerenchyma is still a viable mechanism of releasing CH4 into the atmosphere from constructed wetlands. In addition, the physiological activities that release exudate and oxygen through the root systems during sewage purification would disappear with the harvesting of plants, and the number of methanotrophs would significantly decrease due to the smaller aerobic rhizosphere environment. These factors contribute to the emission of CH4 until the roots rot. Subsequently, owing to the disappearance of plants, similar CH4 emission fluxes would be observed in vegetated and unvegetated constructed wetlands. Similarly, controlled harvesting in free water-surface and horizontal subsurface-flow constructed wetlands can minimize CH4 emissions.
Conclusion
Methane fluxes are only slightly higher in vegetated constructed wetlands than those in unvegetated constructed wetlands while obtaining a better pollutant removal efficiency. The emission of CH4 is significantly influenced by plant specific-species factors during the operation of constructed wetlands, especially Z. latifolia systems, which emit more CH4 than systems with other plant species. Overall, the CH4 flux increases with species richness; however, there is also a special scenario in which the lowest CH4 flux was observed in the three-species system (O. javanica, P. arundinacea and J. effusus). Additionally, no significant result has been observed regarding the effect of plant harvesting on CH4 emissions. Further research on selecting an optimal plant and harvest management in constructed wetlands are required to effectively reduce the emissions of CH4. It is worth noting that the release of root oxygen and exudates, and the mediating function of plants could be used as a parameter for selecting wetland plants when assessing greenhouse gas budgets.
Notes on contributors
Guangming Xu is a post graduated who is studying in our research group. He has studied here since 2017.
Yue Li is the Professor and president of the College of Environmental Science and Engineering, Qingdao University. He is also the leader of the Wetland Ecological and Environmental Research Center of Qingdao University. He mainly studies in the field of environmental manage/risk assessment/water environmental engineering.
Sen Wang Dr. is mainly exploring the waste water treatment.
Fanlong Kong is Professor mainly focus on ecological restoration.
Zhengda Yu Dr. mainly studies the wetland ecology. Professor Kong, Dr. Wang, and Dr. Yu are group members of Wetland Ecological and Environmental Research Center of Qingdao University.
Acknowledgements
The authors acknowledge all colleagues for their contribution to the fieldwork. We really appreciate the enlightening comments from the editor and two reviewers, which have helped us much in improving the quality of our manuscript.
Disclosure statement
No potential conflict of interest was reported by the authors.
Additional information
Funding
References
- Adams CR, Galatowitsch SM. 2005. Phalaris arundinacea (reed canary grass): rapid growth and growth pattern in conditions approximating newly restored wetlands. Ecoscience. 12(4):569–573.
- Ahmad MS, Mehmood MA, Taqvi STH, Elkamel A, Liu CG, Xu JR, Rahimuddin SA, Gull M. 2017. Pyrolysis, kinetics analysis, thermodynamics parameters and reaction mechanism of Typha latifolia to evaluate its bioenergy potential. Bioresource Technol. 245:491–501.
- Barbera AC, Borin M, Cirelli GL, Toscano A, Maucieri C. 2015. Comparison of carbon balance in Mediterranean pilot constructed wetlands vegetated with different C4 plant species. Environ Sci Pollut Res. 22(4):2372–2383.
- Bateganya NL, Mentler A, Langergraber G, Busulwa H, Hein T. 2015. Carbon and nitrogen gaseous fluxes from subsurface flow wetland buffer strips at mesocosm scale in East Africa. Ecol Eng. 85:173–184.
- Bhullar GS, Edwards PJ, Venterink HO. 2013. Variation in the plant-mediated methane transport and its importance for methane emission from intact wetland peat mesocosms. J Plant Ecol. 6(4):298–304.
- Brix H, Sorrel BK, Orr PT. 1992. Internal pressurization and convective gas flow in some emergent freshwater macrophytes. Limnol Oceanogr. 37(7):1420–1433. https://www.jstor.org/stable/2837960
- Brix H, Sorrell BK, Lorenzen B. 2001. Are Phragmites-dominated wetlands a net source or net sink of greenhouse gases?. Aquat Bot. 69(2-4):313–324.
- Cao X, Huang W, Huang DY, Tian YF, Jiang XY, Zhang JB. 2017. Effects of plant species on CH4 emission from integrated vertical subsurface flow constructed wetlands. DWT. 85:358–364.
- Chanton JP, Whiting GJ, Happell D, Gerard G. 1993. Contrasting rates and diurnal patterns of methane emission from emergent aquatic macrophytes. Aquat Bot. 46(2):111–128.
- de la Varga D, Ruiz I, Álvarez JA, Soto M. 2015. Methane and carbon dioxide emissions from constructed wetlands receiving anaerobically pretreated sewage. Sci Total Environ. 538:824–833.
- Denier vanderGon HACD, Neue HU. 1996. Oxidation of methane in the rhizosphere of rice plants. Biol Fertil Soils. 22(4):359–366.
- Duan XN, Wang XK, Ouyang ZY. 2009. Influence of common reed (Phragmites australis) on CH4 production and transport in wetlands: results from single-plant laboratory experiments. Water Air Soil Pollut. 197(1-4):185–191.
- Gallardo MT, Martin BB, Martin DF. 1998. Inhibition of water fern Salvinia minima by cattail (Typha domingensis) extracts and by 2-chlorophenol and salicylaldehyde. J Chem Ecol. 24(9):1483–1490.
- Garcia J, Rousseau DPL, Morato J, Lesage E, Matamoros V, Bayona JM. 2010. Contaminant removal processes in subsurface-flow constructed wetlands: a review. Crit Rev Environ Sci Technol. 40(7):561–661.
- Garnet KN, Megonigal JP, Litchfield C, Taylor GE. 2005. Physiological control of leaf methane emission from wetland plants. Aquat Bot. 81(2):141–155.
- Girkin NT, Turner BL, Ostle N, Craigon J, Sjogersten S. 2018. Root exudate analogues accelerate CO2 and CH4 production in tropical peat. Soil Biol Biochem. 117:48–55.
- Grünfeld S, Brix H. 1999. Methanogenesis and methane emissions: effects of water table, substrate type and presence of Phragmites australis. Aquat Bot. 64(1):63–75.
- Guo WY, Lambertini C, Li XZ, Meyerson LA, Brix H. 2013. Invasion of Old World Phragmites australis in the New World: precipitation and temperature patterns combined with human influences redesign the invasive niche. Global Change Biol. 19(11):3406–3422. http://10.1111/gcb.12295
- Han WJ, Shi MM, Chang J, Ren Y, Xu RH, Zhang CB, Ge Y. 2017. Plant species diversity reduces N2O but not CH4 emissions from constructed wetlands under high nitrogen levels. Environ Sci Pollut Res. 24(6):5938–5948.
- Hanson RS, Hanson TE. 1996. Soil microorganisms as controllers of atmosphere trace gases (H2, CO, CH4, OCS, N2O, and NO. Microbiol Rev. 60(4):609–640.
- He D, Simoneit BRT, Jara B, Jaffé R. 2015. Gas chromatography mass spectrometry based profiling of alkyl coumarates and ferulates in two species of cattail (Typha domingensis P., and Typha latifolia L.). Phytochem Lett. 13:91–98.
- Henneberg A, Brix H, Sorrell BK. 2016. The interactive effect of Juncus effusus and water table position on mesocosm methanogenesis and methane emissions. Plant Soil. 400(1-2):45–54.
- Hoagland DR, Arnon DI. 1950. The water culture method for growing plants without soil. Circ Calif Agric Exp Stn. 347(5406):357–359.
- Inamori R, Gui P, Dass P, Matsumura M, Xu KQ, Kondo T, Ebie Y, Inamori Y. 2007. Investigating CH4 and N2O emissions from eco-engineering wastewater treatment processes using constructed wetland microcosms. Process Biochem. 42(3):363–373.
- IPCC 2014. Climate Change 2014: Mitigation of Climate Change. Contribution of Working Group III to the Fifth Assessment Report of the Intergovernmental Panel on Climate Change. Cambridge University Press, Cambridge, United Kingdom and New York, NY, USA.
- Jackson MB, Armstrong W. 1999. Formation of aerenchyma and the processes of plant ventilation in relation to soil flooding and submergence. Plant Biol (Stuttg). 1(3):274–287.
- Jia W, Zhang J, Li P, Xie H, Wu J, Wang J. 2011. Nitrous oxide emissions from surface flow and subsurface flow constructed wetland microcosms: effect of feeding strategies. Ecol Eng. 37(11):1815–1821.
- Johansson AE, Gustavsson AM, Öquist MG, Svensson BH. 2004. Methane emissions from a constructed wetland treating wastewater-seasonal and spatial distribution and dependence on edaphic factors. Water Res. 38(18):3960–3970.
- Kirschke S, Bousquet P, Ciais P, Saunois M, Canadell JG, Dlugokencky EJ, Bergamaschi P, Bergmann D, Blake DR, Bruhwiler L, et al. 2013. Three decades of global methane sources and sinks. Nature Geosci. 6(10):813–823.
- Laanbroek HJ. 2010. Methane emission from natural wetlands: interplay between emergent macrophytes and soil microbial processes. A mini-review. Ann Bot. 105(1):141–153.
- Lai W, Wang S, Peng C, Chen Z. 2011. Root features related to plant growth and nutrient removal of 35 wetland plants. Water Res. 45(13):3941–3950.
- Lavergne S, Molofsky J. 2004. Reed canary grass (Phalaris arundinacea) as a biological model in the study of plant invasion. Crit Rev Plant Sci. 23(5):415–429.
- Lewandowski I, Scurlock JMO, Lindvall E, Christou M. 2003. The development and current status of perennial rhizomatous grasses as energy crops in the US and Europe. Biomass Bioenergy. 25(4):335–361.
- Li XY, Ding AZ, Zheng L, Xing L, Dong YL, Tan Z, Liu BY, Zhang L. 2018. Application of Constructed Wetlands for Water Pollution Treatment in China During 1990–2015. Environ Eng. 26(4):11–17.
- Liikanen A, Huttunen JT, Karjalainen SM, Heikkinen K, Väisänen TS, Nykänen H, Martikainen PJ. 2006. Temporal and seasonal changes in greenhouse gas emissions from a constructed wetland purifying peat mining runoff waters. Ecol Eng. 26(3):241–251.
- Liu D, Ge Y, Chang J, Peng CH, Gu BH, Chan GYS, Wu XF. 2009. Constructed Wetlands in China: recent developments and future challenges. Front Ecol Environ. 7(5):261–268.
- Maltais-Landry G, Maranger R, Brisson J, Chazarenc F. 2009. Greenhouse gas production and efficiency of planted and artificially aerated constructed wetlands. Environ Pollut. 157(3):748–754.
- Mander U, Tournebize J, Kasak K, Mitsch WJ. 2014. Climate regulation by free water surface constructed wetlands for wastewater treatment and created riverine wetlands. Ecol Eng. 72:103–115.
- Maucieri C, Borin M, Barbera AC. 2014. Role of C3 plant species on carbon dioxide and methane emissions in Mediterranean constructed wetland. Ital J Agronomy. 9(3):120–126.
- Maucieri C, Mietto A, Barbera AC, Borin M. 2016. Treatment performance and greenhouse gas emission of a pilot hybrid constructed wetland system treating digestate liquid fraction. Ecol Eng. 94:406–417.
- Maurer D, Zedler JB. 2002. Differential invasion of a wetland grass explained by tests of nutrients and light availability on establishment and clonal growth. Oecologia. 131(2):279–288.
- McCormick MK, Kettenring KM, Baron HM, Whigham DF. 2010. Extent and reproductive mechanisms of Phragmites australis spread in brackish wetlands in Chesapeake Bay, Maryland (USA). Wetlands. 30(1):67–74.
- McInerney E, Helton AM. 2016. The effects of soil moisture and emergent herbaceous vegetation on carbon emissions from constructed wetlands. Wetlands. 36(2):275–284.
- Megonigal JP, Neubauer SC. 2019. Biogeochemistry of tidal freshwater wetland. In: Coastal wetlands. Amsterdam: Elsevier, p. 641–683. DOI: https://doi.org/10.1016/B978-0-444-63893-9.00019-8
- Meyerson LA, Saltonstall K, Windham L, Kiviat E, Findlay S. 2000. A comparison of Phragmites australis in freshwater and brackish marsh environments in North America. Wetlands Ecol Manag. 8(2/3):89–103.
- Mitsch WJ, Zhang L, Waletzko E, Bernal B. 2014. Validation of the ecosystem services of created wetlands: two decades of plant succession, nutrient retention, and carbon sequestration in experimental riverine marshes. Ecol Eng. 72:11–24.
- Mo Y, Deng ZH, Gao JQ, Guo YX, Yu FH. 2015. Does richness of emergent plants affect CO2 and CH4 emissions in experimental wetlands?. Freshw Biol. 60(8):1537–1544.
- Moran MA, Hodson RE. 1989. Bacterial secondary production on vascular plant detritus: relationships to detritus composition and degradation rate. Appl Environ Microbiol. 55(9):2178–2189.
- Mozdzer TJ, Zieman JC. 2010. Ecophysiological differences between genetic lineages facilitate the invasion of non-native Phragmites australis in North American Atlantic coast wetlands. J Ecol. 98(2):451–458.
- Niu CZ, He ZX, Ge Y, Chang J, Lu ZM. 2015. Effect of plant species richness on methane fluxes and associated microbial processes in wetland microcosms. Ecol Eng. 84:250–259.
- Nouchi I, Hosono T, Aoki K, Minami K. 1994. Seasonal-variation in methane fluxes from rice paddies associated with methane concentration in soil-water, rice biomass and temperature, and its modeling. Plant Soil. 161(2):195–208.
- Rice AL, Butenhoff CL, Shearer MJ, Teama D, Rosenstiel TN, Khalil MAK. 2010. Emissions of anaerobically produced methane by trees. Geophys Res Lett. 37:1–5.
- Rusch H, Rennenberg H. 1998. Black alder (Alnus glutinosa (L.) Gaertn.) trees mediate methane and nitrous oxide emission from the soil to the atmosphere. Plant Soil. 201(1):1–7.
- Saarnio S, Wittenmayer L, Merbach W. 2004. Rhizospheric exudation of Eriophorum vaginatum L.—potential link to methanogenesis. Plant Soil. 267(1-2):343–355.
- Saunois M, Bousquet P, Poulter B, Peregon A, Ciais P, Canadell JG, Dlugokencky EJ, Etiope G, Bastviken D, Houweling S, et al. 2016. The global methane budget 2000–2012. Earth Syst Sci Data. 8(2):697–751.
- Schimel JP. 1995. Plant transport and methane production as controls on methane flux from arctic wet meadow tundra. Biogeochemistry. 28(3):183–200.
- Schütz H, Seuler W, Conrad R. 1989. Processes involved in formation and emission of methane in rice paddies. Biogeochemistry. 7(1):33–53.
- Segers R. 1998. Methane production and methane consumption: a review of processes underlying wetland methane fluxes. Biogeochemistry. 41(1):23–51.
- Sorrell BK, Boon PI. 1994. Convective gas flow in Eleocharis sphacelata R. Br.: methane transport and release from wetlands. Aquat Bot. 47(3-4):197–212.
- Ström L, Lamppa A, Christensen TR. 2007. Greenhouse gas emissions from a constructed wetland in southern Sweden. Wetlands Ecol Manage. 15(1):43–50.
- Ström L, Mastepanov M, Christensen TR. 2005. Species-specific effects of vascular plants on carbon turnover and methane emissions from wetlands. Biogeochemistry. 75(1):65–82.
- Sutton-Grier AE, Megonigal JP. 2011. Plant species traits regulate methane production in freshwater wetland soils. Soil Biol Biochem. 43(2):413–420. DOI: https://doi.org/10.1016/j.soilbio.2010.11.009
- Sutton-Grier AE, Keller JK, Koch R, Gilmour C, Megonigal JP. 2011. Electron donors and acceptors influence anaerobic soil organic matter mineralization in tidal marshes. Soil Biol Biochem. 43(7):1576–1583. DOI: https://doi.org/10.1016/j.soilbio.2011.04.008
- Terazewa K, Ishizuka S, Sakata T, Yamada K, Takahashi M. 2007. Methane emissions from stems of Fraxinus mandshurica var. japonica trees in a floodplain forest. Soil Biol Biochem. 39(10):2689–2692.
- Uddin MN, Robinson RW, Buultjen A, Al Harun MAY, Shampa SH. 2017. Role of allelopathy of Phragmites australis in its invasion processes. J Exp Mar Biol Ecol. 486:237–244.
- Van Der Nat FJWA, Middelburg JJ. 1998. Effects of two common macrophytes on methane dynamics in freshwater sediments. Biogeochemistry. 43(1):79–104.
- van Ruijven J, Berendse F. 2003. Positive effects of plant species diversity on productivity in the absence of legumes. Ecol Lett. 6(3):170–175.
- Vymazal J. 2011. Plants used in constructed wetlands with horizontal subsurface flow: a review. Hydrobiologia. 674(1):133–156.
- Wang Q, Chen J, Liu F, Li W. 2014. Morphological changes and resource allocation of Zizania latifolia (Griseb.) Stapf in response to different submergence depth and duration. Flora. 209(5-6):279–284.
- Wang R, Korboulewsky N, Prudent P, Baldy V, Bonin G. 2009. Can vertical-flow wetland systems treat high concentrated sludge from a food industry? A mesocosm experiment testing three plant species. Ecol Eng. 35(2):230–237.
- Wang RY, Baldy V, Périssol C, Korboulewsky N. 2012. Influence of plants on microbial activity in a vertical-downflow wetland system treating waste activated sludge with high organic matter concentrations. J Environ Manag. 9:S158–S164.
- Wang Y, Inamori R, Kong H, Xu KQ, Inamori Y, Kondo T, Zhang J. 2008. Influence of plant species and wastewater strength on constructed wetland methane emissions and associated microbial populations. Ecol Eng. 32(1):22–29.
- Wang YH, Yang H, Ye C, Chen X, Xie B, Huang CC, Zhang JX, Xu MN. 2013a. Effects of plant species on soil microbial processes and CH4 emission from constructed wetlands. Environ Pollut. 174:273–278.
- Wang YH, Ye C, Yang H, Zhang JX, Huang CC, Xie B. 2013b. Methane formation in soil–plant systems treating wastewater as influenced by microbial populations. Environ Earth Sci. 70(4):1647–1652.
- Whiting GJ, Chanton JP. 1993. Primary production control of methane emission from wetlands. Nature. 364(6440):794–795.
- Wießner A, Kuschk P, Stottmeister U. 2002. Oxygen release by roots of Typha latifolia and Juncus effusus in laboratory hydroponic systems. Acta Biotechnol. 22(1-2):209–216.
- Wild U, Kamp T, Lenz A, Heinz S, Pfadenhauer J. 2001. Cultivation of Typha spp. in constructed wetlands for peatland restoration. Ecol Eng. 17(1):49–54.
- Wu HM, Zhang J, Ngo HH, Guo WS, Liang S. 2017. Evaluating the sustainability of free water surface flow constructed wetlands: methane and nitrous oxide emissions. J Clean Prod. 147:152–156.
- Wu SB, Wallace S, Brix H, Kuschk P, Kirui WK, Masi F, Dong RJ. 2015. Treatment of industrial effluents in constructed wetlands: challenges, operational strategies and overall performance. Environ Pollut. 201:107–120.
- Xue Y, Kovacic DA, David MB, Gentry LE, Mulvaney RL, Lindau CW. 1999. In situ measurements of denitrification in constructed wetlands. J Environ Qual. 28(1):263–269.
- Yan C, Zhang H, Li B, Wang D, Zhao Y, Zheng Z. 2012. Effects of influent C/N ratios on CO2 and CH4 emissions from vertical subsurface flow constructed wetlands treating synthetic municipal wastewater. J Hazard Mater. 203:188–194.
- Ye ZH, Baker AJM, Wong MH, Willis AJ. 1997. Zinc, lead and cadmium tolerance, uptake and accumulation by Typha latifolia. New Phytol. 136(3):469–480.
- Zemanová K, Picek T, Dušek J, Edwards K, Šantrůčková H. 2010. Carbon, nitrogen and phosphorus tranformations are related to age of a constructed wetland. Water Air Soil Pollut. 207(1-4):39–48.
- Zhai X, Piwpuan N, Arias CA, Headley T, Brix H. 2013. Can root exudates from emergent wetland plants fuel denitrification in subsurface flow constructed wetland systems?. Ecol Eng. 61:555–563.
- Zhang CB, Liu WL, Wang J, Ge Y, Ge Y, Chang SX, Chang J. 2011. Effects of monocot and dicot types and species richness in mesocosm constructed wetlands on removal of pollutants from wastewater. Bioresour Technol. 102(22):10260–10265.
- Zhang CB, Sun HY, Ge Y, Gu BJ, Wang H, Chang J. 2012a. Plant species richness enhanced the methane emission in experimental microcosms. Atmos Environ. 62:180–183.
- Zhang T, Xu D, He F, Zhang YY, Wu ZB. 2012b. Application of constructed wetland for water pollution control in China during 1990–2010. Ecol Eng. 47:189–197.
- Zhao ZY, Chang J, Han WJ, Wang M, Ma DP, Du YY, Qu ZL, Chang SX, Ge Y. 2016a. Effects of plant diversity and sand particle size on methane emission and nitrogen removal in microcosms of constructed wetlands. Ecol Eng. 95:390–398.
- Zhao ZY, Zhong YC, Han WJ, Tang YL, Fan LK, Fan X, Chang J, Ge Y. 2016b. Effects of plant diversity on methane emission in microcosms of constructed wetlands. Chin J Ecol. 35(7):1783–1790.
- Zhu NW, An P, Krishnakumar B, Zhao L, Sun LW, Mizuochi M, Inamori YH. 2007. Effect of plant harvest on methane emission from two constructed wetlands designed for the treatment of wastewater. J Environ Manag. 85(4):936–943.