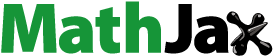
Abstract
Increasing demand for freshwater during the last century has so severely degraded many wetland ecosystems that they are some of the most seriously impacted environments in the world. Environmental flows are used as a management tool to restore parts of the hydrological regime altered by human water use, to rehabilitate these wetlands. Research and monitoring to date has focused on understanding ‘flow-ecology’ relationships, without investigating the mechanisms underlying them. We sought to understand how different basal food sources are incorporated into fish tissue in temporary wetland systems. This study provides a necessary first step toward the development of mechanistic research that investigates the effects of variation in fatty acids (FA) within the food and prey base on top predators. We added different sources of fatty acids to wetland mesocosms by adding extra food sources including redgum leachate to increase planktonic bacteria populations, cyanobacteria, green algae and biofilm matrix to observe how they were incorporated into secondary consumers. Wetland soil and water was added to replicate mesocosms, left for 28 days to produce zooplankton and then Western carp gudgeons added. There was a clear shift in the invertebrate assemblage structure following the introduction of the gudgeons. There was also a clear difference in assemblage structure and nutritional value between benthic and planktonic invertebrates. However, the addition of extra food sources did not generate differing FA profiles between treatments in the substratum fractions, invertebrates or fish after 14 days. We suggest that food sources generated within the mesocosms themselves may have outweighed any treatment effects. Using flooded wetland mesocosms potentially would have provided more realistic knowledge of the food web mechanisms of wetland inundation rather than feeding zooplankton fed specific primary food sources to fish. However, future experiments attempting to identify the mechanisms of the transfer of basal food sources in wetlands to secondary consumers may wish to directly feed fish primary consumers raised on specific basal food sources.
Introduction
Increasing demand for freshwater during the last century has severely affected wetland ecosystems to the point that they represent some of the most seriously degraded environments in the world (Lemly et al. Citation2000; Vorosmarty et al. Citation2010; Davidson et al. Citation2018). Most the world’s river systems are now subject to human impacts (Tonkin et al. Citation2019), requiring increasingly sophisticated approaches to river management (Thompson et al. 2019). An important tool which has emerged has been the management of flows to restore, protect or enhance environmental outcomes both in-channel and in associated floodplains (Whipple and Viers Citation2019). While there has been considerable progress in the science underpinning provision of flows for the environment (hereafter ‘environmental flows’) there remain substantive gaps in our understanding (Poff and Zimmerman Citation2010; Davies et al. Citation2014; Arthington et al. Citation2018). These include an understanding of the processes which link flow variability to fluxes of energy to enhance condition, fitness and recruitment of higher consumers such as fish and birds (Rolls et al. Citation2017).
Australia, the world’s driest inhabited continent, has experienced profound water stress which has had substantive environmental, social and economic impacts. In south‐eastern Australia the Murray–Darling Basin (MDB), Australia’s largest food growing region, has experienced acute ecological impacts of water resource development (Kingsford Citation2000; Jenkins et al. Citation2005; Murray-Darling Basin Authority Citation2011a). In the MDB, the high level of water use has proven to be unsustainable, both in meeting the needs of irrigators for agriculture and for the ecological health of rivers and wetlands (Kingsford Citation2000; Arthington and Pusey Citation2003; Murray-Darling Basin Authority 2011b; Docker and Robinson Citation2014). Increasing the share of environmental water and improving environmental water management are central elements of current water reform in the MDB (Murray-Darling Basin Authority 2011b; Docker and Robinson Citation2014). A monitored program of delivering water dedicated to the environment to instream channels and wetlands has now been in place for seven years, providing the basis for internationally significant insights into ecological responses to environmental flows (Horne et al. Citation2018).
Environmental flows are used as a management tool to restore parts of the hydrological regime altered by human water use to rehabilitate the environment (Arthington Citation2012). These flows aim to restore overall ecosystem health, but frequently express targets in terms of population level outcomes in higher-order consumers such as iconic fish and bird species (Gawne et al. Citation2014). Research and monitoring to date has focused on understanding these ‘flow-ecology’ relationships, without investigating the mechanisms underlying them (Rolls et al. Citation2017). The production and transfer of trophic energy within a system is a process fundamental to the success of higher-order consumers (Lowe et al. Citation2006). A better understanding of the interaction between hydrological flow regimes, trophic dynamics and higher-order consumers would allow a more thorough process-based evaluation of environmental flows (Robson et al. Citation2017).
A key element of the transfer of energy through food webs is the quality of different food resources. Food quality affects the growth, development, and mortality of consumers (Rossi et al. Citation2006; Malzahn et al. Citation2007; Masclaux et al. Citation2012; Paulsen et al. Citation2014). For vertebrate consumers, fatty acids are especially important, as they are required for neural and visual development (Kainz et al. Citation2004; Masclaux et al. Citation2012). The fatty acids 18:3n-3 α-linolenic acid (ALA), and 18:2n-6 linoleic acid (LA), cannot be directly produced by heterotrophic organisms and must come from food (Arts et al. Citation2009). The polyunsaturated fatty acids (PUFA) 20:5n-3 eicosapentaenoic acid (EPA), 22:6n-3 docosahexaenoic acid (DHA), and 20:4n-6 arachidonic acid (ARA) are required by all organisms and play a role in health and cell function (Dalsgaard et al. Citation2003). Fatty acids are chemically varied, are often incorporated unaltered into higher-order consumers, and different organisms can have distinct profiles (Arts et al. Citation2009). Fatty acids may therefore act as both dietary tracers in the food web and indicators of overall food quality (Iverson et al. Citation2004; Ravinet et al. Citation2010; Gonçalves et al. Citation2012; Magnone et al. Citation2015; Guo et al. Citation2017; Kohlbach et al. Citation2017).
Feeding trials in which invertebrates and fish were provided with various food sources of differing fatty acid composition generally indicate that quality ranges from bacteria (lowest) through cyanobacteria (moderate) to green algae (most nutritious) (Peltomaa et al. Citation2017). However there have been, to date, no studies of how environmental flows may influence resource availability and thus consumer growth and condition. We sought to understand how different food sources are incorporated into fish tissue in temporary wetland systems. We added different sources of fatty acids to artificial wetland mesocosms reflecting the inputs likely to occur due to environmental flows. These included; redgum (Eucalyptus camaldulensis) leaf leachate, cyanobacteria, green algae and biofilm matrix (comprising algae, bacteria and fungi). These were used to represent basal resources which are known to alter during provision of environmental flows (Wolfenden et al. Citation2018). Red gum is the dominant floodplain tree in the MDB, and leachate additions reflected scenarios where environmental water inundated leaf litter on in-stream benches or on the floodplain. Red gum leachate was intended to also increase planktonic bacteria through the provision of dissolved organic carbon (DOC). Cyanobacteria (e.g. Williamson et al. Citation2018), phytoplankton, green algae and biofilm availability can be altered both directly, and indirectly via nutrient inputs, during environmental flows (e.g. Sheldon and Walker Citation1997; Ryder et al. Citation2006).
This study provides a necessary first step toward the development of mechanistic research that investigates the effects of variation in FA within the food and prey base on top predators. Invertebrates and fish were placed in mesocosms treated with the different basal resource types. We hypothesised that 1. invertebrates and fish that were fed different food sources would attain differing FA profiles and 2. differences in the FAs available from those different food sources would result in differences in somatic growth of invertebrates and fish, ultimately resulting in differences in fish sizes and invertebrate community composition.
Methods
Mesocosm design
Mesocosm experiments were established at the University of New England (UNE) campus at Armidale, NSW. Soil was collected from a dry wetland (to a depth of approximately 10 mm) on the Gingham watercourse, New South Wales, Australia (29°16′S 149°21′E) in October 2017. Vegetation on the site is classified as water couch marsh grassland (Bowen and Simpson Citation2010; Southwell et al. Citation2015). Soil was transported to the UNE campus then passed through a 10-mm mechanical sieve for homogenization and to remove roots and vegetation. Fifteen 500-L vats with a basal surface area of 0.78 m2 were placed in an open-ended structure covered with white polyethylene which allowed enough ambient light for algae and vegetation growth. Vats were filled to a depth of 300 mm with potable water, allowed to stand for three days to dechlorinate and maintained at that depth for the remainder of the experiment. Ten kilograms of wetland soil was added at the end of the three days resulting in a soil depth of approximately 10 mm. The vats were gently aerated with diffusers. The vats were allowed to stand for 28 days prior to the introduction of fish in order to produce enough invertebrate biomass from sediments to sustain juvenile fish. Individual shield shrimp (Triops australiensis) were removed from the vats when observed, since a pilot study and (Boulton and Lloyd Citation1992) had shown that either their activity within the soils or their feeding behaviour reduced the populations of other invertebrates of suitable prey size for small fish.
Young Western carp gudgeons (Hypseleotris spp.) were collected in February 2018 from Tarban Creek (28°58′21″S; 151°54′1″E), using bait traps (360 × 240 × 240 mm, 5 mm mesh) set overnight. Fish were transported to the mesocosms, where eight individuals were randomly selected and added to each vat.
One of three potential food sources was added daily to each of three replicate vats; 1/cyanobacteria as commercially available dried Aphanizomenon flos-aquae powder, 2/green algae as commercially available dried Chlorella powder and 3/dried biofilm powder. In addition, three vats were dosed with dissolved organic carbon (DOC) every four to five days and three vats with no added food sources acted as a control treatment. Dissolved organic carbon (DOC) was sourced from river redgum (Eucalyptus camaldulensis) leaf leachate following the methods of O’Connell et al. (Citation2000). The leachate was produced by submerging 800 g of dry leaves in 45 L of chlorinated potable water for 72 hrs at 3 °C, to reduce the consumption by bacteria until added to the vats. The biofilm was collected from wooden redgum tiles that were incubated within the photic zone of a 1 ML dam for six weeks, dried at 40 °C to constant weight and ground to a fine powder. Seventy micrograms of powder was added to the cyanobacteria treatments, 30 µg of powder to the green algae treatments and 70 µg of powder to the biofilm treatments. The amount of cyanobacteria and green algal powder added to each vat was calculated to increase the chlorophyll a (Chla) concentration by 4 µg/L, effectively doubling the average concentration identified in a previous mesocosm experiment (Growns, unpublished data).
Sampling of the mesocosms
Prior to the introduction of the gudgeons planktonic and benthic invertebrates were sampled to estimate abundance and assemblage structure. Zooplankton were sampled by haphazardly taking five separate 200 ml aliquots of mesocosm water from each tank, combined, preserved in ethanol and stained with rose Bengal. Benthic invertebrates were sampled following King (Citation2004) by taking one core (50 mm diameter x 120 mm long with 250 mL volume) for each tank, put through a 250 µm sieve, preserved in ethanol and stained with rose Bengal. Fourteen days after the introduction of fish planktonic and benthic invertebrates were again sampled from each vat using the same methods. Additional plankton and benthos were collected using a 400 µm net to provide enough material for fatty acid profiling from each vat prior to them being drained to remove the remaining gudgeons. Following the draining of the vats organic components of the sediments were collected by elutriation with water and passed through a series of sieves to obtain coarse particulate organic matter (CPOM; 400 µm to 250 µm) , fine particulate organic matter (FPOM; 250 µm to 125 µm) and very fine particulate organic matter (VFPOM; 125 µm to 63 µm).
Gudgeons were anesthetised, measured for standard length, weighed and their entire alimentary canal removed and preserved in ethanol for gut content analysis. Invertebrates were identified to Genus for clam shrimp, shield shrimp and snails (Spinicaudata, Notostraca and Gastropoda, respectively), Family for true flies (Diptera) and water-fleas (Cladocera), Order for copepods (Copepoda), Class for seed shrimp (Ostracoda) and Phylum for roundworms (Nematoda) and rotifers (Rotifera).
Gut content analysis
The contents of the alimentary canal of each fish captured at the end of the experiment were spread out on a small petri dish and examined under a binocular microscope. All taxa were counted and identified to the taxonomic level outlined in the previous section, with the exception of Macrothricidae and Daphnidae, which were pooled as most of them could not be distinguished due to partial digestion of their exoskeletons.
Fatty acid analyses
Profiling of FA followed the methods used by Conlan et al. (Citation2017). Briefly, lipid was extracted from dry samples soaked dichloromethane: methanol (CH2Cl2:CH3OH) and quantified gravimetrically on a 4-digit balance. FA were extracted following lipid class analysis determined using an Iatroscan MK 6 s thin layer chromatography-flame ionisation detector. Following extraction, FA were esterified into methyl esters using the acid catalysed methylation method (Christie Citation2003). Gas chromatography was then used to identify the FA methyl esters relative to known external standards. FA profiling was undertaken on benthic and pelagic invertebrates, gudgeons taken from the start and finish of the experiment, the three substratum size fractions from each of the three replicate mesocosms and the algal and biofilm powders.
To determine the primary food source in gudgeons at the end of the experiment we used the following individual FA as biomarkers, myristic acid (14:0) for cyanobacteria (Carpenter et al. Citation1997; Kelly and Scheibling Citation2012), pentadecylic acid (15:0) and margaric acid (17:0) for bacteria (Dalsgaard et al. Citation2003; Alfaro et al. Citation2006), oleic acid (18:1n-9) for fungi (Vestal and White Citation1989; Willers et al. Citation2015), alpha-linolenic acid (18:3n-3) for green algae (D’Souza and Loneragan Citation1999; Kelly and Scheibling Citation2012) and eicosapentaenoic acid (20:5n-3) for diatoms (Volkman et al. Citation1989). The nutritional value of various food sources was defined as the sum of n-3 and n-6 PUFA.
Fish dietary preference
We used the Manly-Chesson index α (Manly Citation1974; Chesson Citation1978, Citation1983) to assess prey selection. The equation for the Manly-Chesson index is as follows:
where ri is the relative abundance of prey taxon ‘i’ found in the larval diet, pi is the relative abundance of the same prey item found in the environment and m is the number of food items. A value less than 1/m indicates a prey group that was consumed disproportionately less than its relative abundance in the environment. Values near 1/m indicate that a prey taxon was consumed in direct proportion to its abundance, and values greater than 1/m indicate a prey group was consumed disproportionately more than its relative abundance in the resource base, with values near 1.0 indicating a strong selection of a prey item or ‘preference’. Separate calculations were performed for the planktonic and benthic invertebrates in each treatment.
Statistical analyses
All statistical analyses were conducted using PERMANOVA + for PRIMER software (Anderson et al. Citation2008). Permutational analysis of variance (PERMANOVA) (Anderson Citation2001) was used to identify significant sources of variation among factors (Supplementary material Table S1) using 9999 randomisations. Bray–Curtis dissimilarity was used to define distances among samples for multivariate data (e.g. FA profiles or invertebrate assemblages) and Euclidean distance for the univariate data. Invertebrate abundance data was converted to ranks within each habitat type and Kendall rank correlation coefficients were used to form the similarity matrix for PERMANOVA analysis. The homogeneity of variance, that is equal dispersion, among groups of the main factors was tested using PERMDISP (Anderson Citation2001). Patterns of differences in invertebrate assemblages and fatty acid profiles identified by PERMANOVA were presented graphically using bootstrap averaging ordination with 100 randomized starts (Clarke et al. Citation2014). The variables responsible for the separation of significantly different groups in multivariate data were tested using similarity percentages (SIMPER) in the PRIMER software (Clarke Citation1993). The consistency ratio (the ratio of the average to standard deviation of the dissimilarities between groups) was calculated for each species that had a greater than 3% contribution to the average dissimilarity.
Results
Fatty acid profiles
A total of 47 individual fatty acids were identified from the food sources, substratum fractions and animal groups (Supplementary material Table S2). Palmitic acid (16:0) was present in greater than 20% of all samples and oleic acid (18:1n-9) greater than 10% in all the substratum factions, green algae and biofilm (Supplementary material Table S2). At commencement of our mesocosm experiment gudgeon tissue contained palmitic acid and oleic acid in similar proportions to the substratum fractions, however the proportion of oleic acid among total lipids increased in gudgeon tissue to more than 15% by the end of the experiment. Palmitic acid accounted for more than 30% of total lipids among all benthic invertebrate samples, while stearic acid (18:0) occurred in proportions above 15%, with no other single fatty acid accounting for more than 10% of total lipids. Planktonic taxa also had palmitic acid and oleic acid in proportions exceeding 10% of total lipids as well as two additional fatty acids alpha linolenic acid (ALA, 18:3n-3) and palmitoleic acid (16:1n-7). The cyanobacteria food source differed from the other two food sources by having the greatest percentage of palmitic acid, myristic acid (14:0) and tetracosapentaenoic acid 24:5n-3. Biofilm had the greatest percentage of stearic acid and oleic acid (18:1n-9). In contrast, green algae had the highest concentration of alpha-linolenic acid (18:3n-3).
The fatty acid profiles of the different groups (substratum fractions and animal groups) and between treatments were significantly different with no interaction between those factors (). Pairwise tests demonstrated fatty acid profiles of each group differed significantly from one another (). In addition, the variation within groups differed significantly between the different groups, with fish demonstrating little variation compared with benthic invertebrates and zooplankton (, ). The fatty acid profiles from the different treatments of the different groups (substratum fractions and animal groups) were all significantly different from each other with the exception that the control treatments were not different from the DOC and green algal treatments and the green algal treatment was not different to the biofilm and BGA treatments.
Figure 1. Bootstrap average ordination of fatty acid profiles of the different groups. Solid symbols represent average locations for particular groups. Ellipses represent regions in which 95% of these bootstrap averages fall for each group.
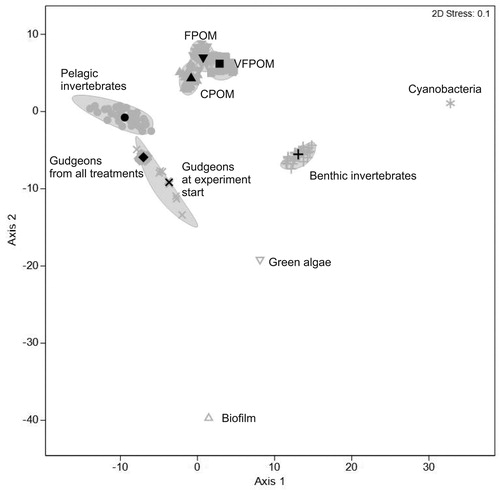
Table 1. Pseudo-F values and probability levels for fatty acid profiles and food source biomarkers for differences between groups (substratum fractions and biotic groups) and mesocosm treatments.
The mean percentage of each of the basal source biomarkers differed between groups and the bacterial and fungal biomarkers differed between treatments, although the low pseudo-F values indicated that only a small fraction of the variance was explained by treatment (). The cyanobacterial fatty acid biomarker (myristic acid) was highest in the benthic invertebrate group regardless of treatment (Supplementary material Figure S1). In contrast, the mean percentage of the fungal biomarker (oleic acid) was lowest in the benthic invertebrates. In general, the mean percentage of green algal and diatom biomarkers (alpha-linolenic and eicosapentaenoic acids, respectively) was highest in the planktonic invertebrates, followed by the fish.
Fatty acid profiles of gudgeons at the start of the experiment were significantly different to fatty acid profiles of gudgeons from all treatments at the end of the mesocosm experiment (, ). However, there was no significant difference in the fatty acid profiles of gudgeons in treatments at completion of the experiment. SIMPER analysis suggested that three fatty acids contributed to the differences between gudgeons at the beginning and the end of the experiment. These included oleic acid (18:1n-9) and arachidonic acid (ARA, 20:4n-6) which increased from 10.8% to 15.6% and 4.7% and 7.6% respectively and palmitoleic acid (16:1n-7) which decreased from 8.6% to 5.8%.
Figure 2. Mean percentage (±1 S.E.) of fatty acid biomarkers for different possible basal food sources among six gudgeon treatments. Black columns indicate the cyanobacterial biomarker, striped indicate bacterial markers, grey indicate the fungal marker, white indicate the green algal biomarker and spotted the diatom biomarker.
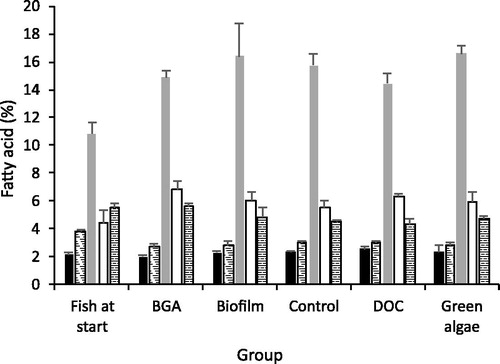
Table 2. Pseudo-F values and probability levels for fatty acid profiles and basal source biomarkers for differences between fish groups.
The fatty acid biomarkers for bacteria and fungi differed between the fish groups (). Pairwise tests indicated that, at the start of the experiment gudgeons had a significantly greater mean percentage of the bacterial markers than at the end (). In contrast, the fish at the start of the experiment had significantly lower mean percentages of the fungal biomarker than at the end.
Nutritional values
Nutritional value (sum of n-3 and n-6 PUFA) differed significantly between groups (substratum fractions and animal groups) (F5,54 = 51.5, p = 0.0001) but not between treatments and there was no significant interaction between factors. In addition, there was significant heterogeneity between groups (F1,5 = 3.6, p = 0.0115) with fish having the lowest variation among samples and the zooplankton the greatest. Pairwise tests indicated that zooplankton and fish had the highest nutritional values of the six groups ().
Figure 3. Mean nutrition value (±1 S.E.) of substratum factions and animal groups. Black columns the BGA treatment, striped columns biofilm, light grey control mesocosms, dotted columns DOC and white columns green algae treatments. Dark grey column represents fish at the start of the experiment.
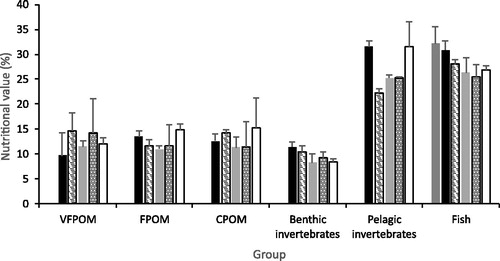
There was a significant difference in the nutritional value of fish among treatments (including fish at the start of the experiment) (pseudo F5,12 = 3.5, p = 0.0249), with no significant heterogeneity between treatments. Fish at the start of the experiment had a significantly higher nutritional value than fish in the remaining treatments ().
Invertebrate assemblage structure
A total of 4264 invertebrates from seven taxa were collected from benthic and planktonic habitats during the course of the experiment. Approximately half of the animals were Daphniidae (49%), and were followed in abundance by Macrothricidae (29%), Ostracoda (12%) and Chironomidae (9%). Chydoridae, Harpacticoida and Nematoda comprised less than one percent of the total abundance.
Time and habitat were the main sources of variation in the invertebrate assemblage structure, explaining 32% and 35%, respectively (). The only other significant source of variation was the habitat by treatment interaction. Post-hoc pairwise tests indicated that the assemblage structure in the DOC and biofilm treatments differed significantly (p < 0.05) from the green algae treatment in the benthic habitat but all the other comparisons between treatments in the benthic and planktonic habitats were not significantly different.
Table 3. Results of PERMANOVA for invertebrate assemblage structure.
Both benthic and planktonic invertebrate communities showed clear shifts in composition from prior to introduction of gudgeons to the mesocosms to those at the end (). Taxa contributing most to dissimilarity between benthic and planktonic assemblages included Chironomidae, Macrothricidae, Ostracoda and Daphniidae (). The ranked abundance of all taxa with the exception of Daphniidae was greater in the benthos. The taxa associated with the differences in assemblage structure through time included the same four taxa associated with the differences between habitats (). The ranked abundance of Chironomidae and Daphniidae increased though time compared with the other two taxa.
Figure 4. Bootstrapped average ordination of invertebrate assemblages in benthic and planktonic habitats before of the introduction of gudgeons into the mesocosms and at the finish of the experiment. Triangles represent benthos and squares planktonic samples. Light grey represents samples prior to the introduction of fish and darker grey invertebrates at the finish of the experiment. Ellipses represent regions in which 95% of these bootstrap averages fall for each group.
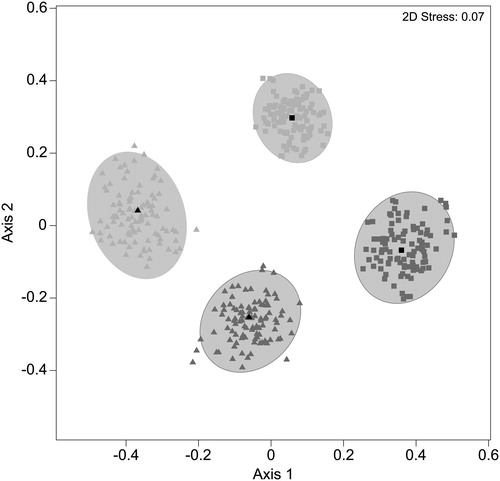
Table 4. Mean ranked abundance and percentage contribution of taxa contributing to the differences between (a) habitat types and (b) time as indicated by SIMPER analysis.
Western carp gudgeons and gut content analysis
Eighty six fish (72%) were recovered from the mesocosms. There was no significant difference in either survival or weight of fish between treatments (pseudo F4,10 = 0.8 and 1.2, respectively, p > 0.05).
A total of 1212 invertebrates from five taxa were identified from the gut contents of the fish. The majority of the invertebrates were Chironomidae (44%) followed by Daphniidae (43%), Chydoridae (11%) and Ostracoda (2%). There were no significant differences in the proportion of dietary items between treatments (pseudo F4,10 = 1.5, p = 0.21).
The mean Manly-Chesson index α differed significantly between taxa (pseudo F3,80 = 31.9, p = 0.0001) but interacted significantly with habitat and treatment (pseudo F3,80 = 11.6 and F12,80 = 3.0, respectively, p = 0.0001). Pairwise tests indicated that the mean α for Chironomidae, Chydoridae and Daphniidae/Macrothricidae differed significantly between habitats but not for Ostracoda. The index was greater for Chironomidae in the planktonic habitat compared with the benthic habitat (). In contrast, the index was greater in the benthic habitat for Chydoridae and the pooled Daphniidae and Macrothricidae. The significant taxon by treatment interaction was most likely the result of a significant difference in mean α between the control and DOC treatments for Chironomidae and Chydoridae but not the other two taxa. Mean α was greater in the control treatment compared with the DOC treatment for Chironomidae in both habitats (). In contrast, the index was greater in the DOC treatment compared with the control in both habitats for Chydoridae.
Figure 5. Mean (±1 S.E.) Manly-Chesson α for different habitats, taxa and treatments. Black columns indicate the cyanobacteria treatment, white columns control, grey columns dissolved organic carbon, diagonal striped columns green algae and horizontal lines the biofilm treatment. The horizontal line is the neutral value for selectiveness.
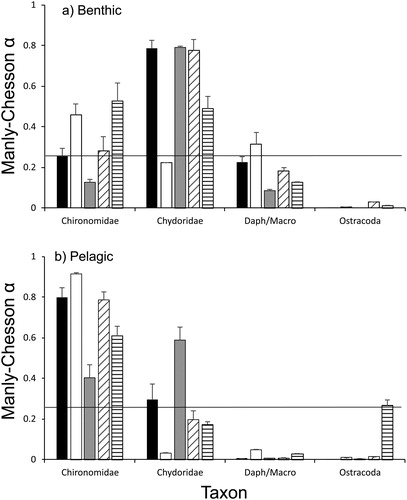
In general, the mean α was greater than the neutral value for selectiveness for Chironomidae in the planktonic habitat and Chydoridae in the benthic habitat (). This indicates that the gudgeons were selecting different taxa in different habitats. In contrast, the mean α was less than the neutral value for selectiveness for both Daphniidae/Macrothricidae and Ostracoda in both habitats, suggesting that gudgeons are avoiding those prey.
Discussion
There was a clear shift in the invertebrate assemblage structure following the introduction of the gudgeons. There was also a clear difference in assemblage structure and nutritional value between habitat types. In addition, the gudgeons appeared to target different taxa in the different habitat types, Chironomidae from the plankton and Chydoridae from the benthos and avoided Ostracoda and Daphniidae/Macrothricidae in both habitat types. Western carp gudgeons are known to eat Daphnidae, Chironomidae and to a lesser extent ostracods and chydorids (Meredith et al. Citation2003; Balcombe and Humphries Citation2006; Balcombe and Closs Citation2016). Through their dietary preferences gudgeons can alter the assemblage structure of zooplankton (Ning et al. Citation2010) but it is unclear if this is the case for benthos. Young gudgeons are known to inhabit benthic and pelagic habitats (Stoffels and Humphries Citation2003) so it is possible that the change in benthic assemblage structure was the result of gudgeon feeding preferences. It is unclear why the gudgeons were selectively feeding in our mesocosms. It may be that the different taxa have different nutritional values but overall the planktonic invertebrates were more nutritious in terms of total PUFA. Experiments which involve the addition of different food sources to wetland mesocosms are rare. We are aware on only one other study, Williams et al. (Citation2007) who added extra leaf material and algal cultures to pond mesocosms. However, similar to our results they found few differences in the invertebrates colonizing the different treatments. This may suggest that food sources generated within the mesocosms themselves may have outweighed any treatment effects.
The addition of extra food sources did not generate differing FA profiles between treatments in the substratum fractions, invertebrates or fish. This is despite the FA profiles of the food sources added differing and being indicative of their different biomarkers; 14:0 for cyanobacteria, 18:3n-3 for green algae and 18:1n-9 for fungi in the biofilm. This suggests that food sources generated within the mesocosms themselves may have outweighed any treatment effects. The significant increase in the fungal FA biomarker in all treatments suggests that the main food source in the mesocosms is derived from fungi. The use of FA as biomarkers is commonplace in the food web literature (e.g. Johns et al. Citation1979; Goedkoop et al. Citation2000; Falk-Petersen et al. Citation2002). However, there are some specific FA that have been attributed to different biotic groups by different authors. For example, the FA 16:0 has been used as a biomarker for green algae, cyanobacteria and fungi (Vestal and White Citation1989; Kelly and Scheibling Citation2012). Biomarkers for specific species of algae are easily overlapped, such as the 18:3n6 has been simultaneously used as a marker for green algae (Meziane and Tsuchiya Citation2000; Xu et al. Citation2014) and cyanobacteria (Hayakawa et al. Citation2002; de Kluijver et al. Citation2012). In addition, the one biomarker for a particular group may not be present for environmental reasons. The FA 20:5 × 3 although an often consistent biomarker for diatoms is often not found in large quantities relative to other FA (Napolitano Citation1999) and may be found in lower light or nutrient concentrations (Ahlgren et al. Citation1992). Given biomarkers for individual species of plankton, aquatic and terrestrial materials are not always specific enough to identify a single source and the fact that the internal biosynthetic capabilities of most organisms have not been elucidated, some authors caution their use in food web studies (Wakeham Citation1995; Dalsgaard et al. Citation2003).
An explanation for the lack of difference in Western carp gudgeon size or survival and FA profiles between treatments may be that the mesocosm experiment was run for insufficient time for any changes to manifest themselves. However, three fatty acids contributed to the differences between gudgeons at the beginning and the end of the experiment. These included increases in oleic acid and ARA and a decrease in palmitoleic acid suggesting that there was sufficient time for changes to occur. In addition, a previous mesocosm experiment involving larval Murray cod demonstrated two weeks was sufficient time for changes in FA profiles (Growns, unpublished data).
Food web and diet studies that attempt to identify the mechanisms for basal food source uptake and conversion generally only occur from the source itself to primary consumers (Taipale et al. Citation2012; Freese and Martin-Creuzburg Citation2013). In these studies the biota are mainly cladoceran species fed specific food sources, i.e. animals cannot choose between food types. Linking FA profiles from basal food sources through primary consumers to secondary consumer are rare and have only occurred in marine systems (Oberg and Fuiman Citation2015). Previous field investigations of fish FA composition in freshwater systems have mainly focused on temporal and spatial changes e.g. Dayhuff and Wells (Citation2005), and a direct, quantitative comparison of fish FA profile to their food sources has yet to be made (Guo et al. Citation2017). Our mesocosm experiment attempted to identify the fate of different primary production sources through primary and then secondary consumers by providing alternate sources of food in addition to that generated from flooding dry wetland sediment. Using flooded wetland mesocosms potentially would have provided more realistic knowledge of the food web mechanisms of wetland inundation rather than feeding zooplankton fed specific primary food sources to fish. However, future experiments attempting to identify the mechanisms of the transfer of basal food sources in wetlands to secondary consumers may wish to directly feed fish primary consumers raised on specific basal food sources.
Supplemental Material
Download MS Word (77.8 KB)Acknowledgements
Michael Faint from University of New England is thanked for proving space in the agronomy polytunnel for the mesocosms. David Francis from Deakin University is thanked for organising the fatty acid profile analyses. Simon Mitrovic from University of Technology Sydney is thanked for organising the counting of bacteria. Sam Lewis is thanked for assisting with the collection of wetland soils. Lindsey Frost made useful comments on earlier drafts of the manuscript.
Disclosure statement
No potential conflict of interest was reported by the author(s).
Additional information
Funding
Notes on contributors
Ivor Growns
Ivor Growns is a Research Fellow at the University of New England, where he has conducted research on food webs and environmental flows.
Darren Ryder
Darren Ryder is a Professor at the University of New England, where he leads the Aquatic Ecology and River Restoration Research Group.
Paul McInerney
Paul McInerney is a Research Scientist at the Commonwealth Scientific and Industrial Research Organisation, where his primary interest is in energy flow within aquatic ecosystems.
Nick Bond
Nick Bond is a Professor at LaTrobe University, where he studies the effects of flow variability on riverine ecosystems.
Galen Holt
Galen Holt is an Associate Research Fellow at Deakin University, where his primary focus on the effects of variation on populations, communities, and ecosystems.
Rebecca Lester
Rebecca Lester is an Associate Professor at Deakin University, where she studies the function and structure of ecosystems and in the development of management strategies that incorporate good ecological outcomes with human activities.
Ross Thompson
Ross Thompson is Director and Professor in the Centre for Applied Water Science at the University of Canberra, where his fundamental research is in food web ecology.
References
- Ahlgren G, Gustafsson IB, Boberg M. 1992. Fatty acid content and chemical composition of freshwater microalgae. J Phycol. 28(1):37–50.
- Alfaro AC, Thomas F, Sergent L, Duxbury M. 2006. Identification of trophic interactions within an estuarine food web (northern New Zealand) using fatty acid biomarkers and stable isotopes. Estuarine Coastal Shelf Sci. 70(1-2):271–286.
- Anderson M, Gorley RN, Clarke RK. 2008. Permanova + for primer: guide to software and statistical methods. Plymouth: Primer-E Limited.
- Anderson MJ. 2001. Permutation tests for univariate or multivariate analysis of variance and regression. Can J Fish Aquat Sci. 58(3):626–639.
- Christie W. 2003. Lipid analysis: isolation, separation, identification and structural analysis of lipids. England: Oily Press.
- Arthington AH. 2012. Environmental flows: saving rivers in the third millennium. London: University of California Press.
- Arthington AH, Kennen JG, Stein ED, Webb JA. 2018. Recent advances in environmental flows science and water management—innovation in the Anthropocene. Freshw Biol. 63(8):1022–1034.
- Arthington AH, Pusey BJ. 2003. Flow restoration and protection in Australian rivers. River Res Appl. 19(5-6):377–395.
- Arts MT, Brett MT, Kainz M. 2009. Lipids in aquatic ecosystems. New York, NY: Springer Science & Business Media.
- Balcombe SR, Closs GP. 2016. Patchiness in food resources drives fish abundances in emergent macrophytes under field and experimental conditions. Hydrobiologia. 781(1):127–142.
- Balcombe SR, Humphries P. 2006. Diet of the western carp gudgeon (Hypseleotris klunzingeri Ogilby) in an Australian floodplain lake: the role of water level stability. J Fish Biology. 68(5):1484–1493.
- Boulton AJ, Lloyd LN. 1992. Flooding frequency and invertebrate emergence from dry floodplain sediments of the River Murray, Australia. Regul Rivers Res Mgmt. 7(2):137–151.
- Bowen S, Simpson SL. 2010. Changes in extent and condition of the vegetation communities of the Gwydir wetlands and floodplain 1996-2008: final report. NSW Wetland Recovery Program, NSW Department of Environment Climate Change and Water, Sydney.
- Carpenter EJ, Harvey HR, Fry B, Capone DG. 1997. Biogeochemical tracers of the marine cyanobacterium Trichodesmium. Deep Sea Res Part I. 44(1):27–38.
- Chesson J. 1978. Measuring preference in selective predation. Ecology. 59(2):211–215.
- Chesson J. 1983. The estimation and analysis of preference and its relatioship to foraging models. Ecology. 64(5):1297–1304.
- Clarke KR. 1993. Non‐parametric multivariate analyses of changes in community structure. Austral Ecol. 18(1):117–143.
- Clarke KR, Gorley RN, Somerfield PJ, Warwick RM. 2014. Change in marine communities: an approach to statistical analysis and interpretation. 3rd ed. Plymouth: PRIMER-E Limited.
- Conlan JA, Rocker MM, Francis DS. 2017. A comparison of two common sample preparation techniques for lipid and fatty acid analysis in three different coral morphotypes reveals quantitative and qualitative differences. PeerJ. 5:e3645.
- D’Souza FML, Loneragan NR. 1999. Effects of monospecific and mixed-algae diets on survival, development and fatty acid composition of penaeid prawn (Penaeus spp.) larvae. Mar Biol. 133(4):621–633.
- Dalsgaard J, John MS, Kattner G, Müller-Navarra D, Hagen W. 2003. Fatty acid trophic markers in the pelagic marine environment. Adv Mar Biol. 46:225–340.
- Davidson NC, Fluet-Chouinard E, Finlayson CM. 2018. Global extent and distribution of wetlands: trends and issues. Mar Freshwater Res. 69(4):620–627.
- Davies PM, Naiman RJ, Warfe DM, Pettit NE, Arthington AH, Bunn SE. 2014. Flow–ecology relationships: closing the loop on effective environmental flows. Mar Freshwater Res. 65(2):133–141.
- Dayhuff LE, Wells MJM. 2005. Identification of fatty acids in fishes collected from the Ohio River using gas chromatography-mass spectrometry in chemical ionization and electron impact modes. J Chromatogr A. 1098(1-2):144–149.
- de Kluijver A, Yu J, Houtekamer M, Middelburg JJ, Liu Z. 2012. Cyanobacteria as a carbon source for zooplankton in eutrophic Lake Taihu, China, measured by 13C labeling and fatty acid biomarkers. Limnol Oceanogr. 57(4):1245–1254.
- Docker B, Robinson I. 2014. Environmental water management in Australia: experience from the Murray-Darling Basin. Int J Water Resour Dev. 30(1):164–177.
- Falk-Petersen S, Dahl TM, Scott CL, Sargent JR, Gulliksen B, Kwasniewski S, Hop H, Millar R-M. 2002. Lipid biomarkers and trophic linkages between ctenophores and copepods in Svalbard waters. Mar Ecol Prog Ser. 227:187–194.
- Freese HM, Martin-Creuzburg D. 2013. Food quality of mixed bacteria–algae diets for Daphnia magna. Hydrobiologia. 715(1):63–76.
- Gawne B, Roots J, Hale J, Stewardson M. 2014. Commonwealth Environmental Water Office long–term intervention monitoring project: basin evaluation plan. Report prepared for the Commonwealth Environmental Water Office by The Murray–Darling Freshwater Research Centre. MDFRC Publication 42:2014.
- Goedkoop W, Sonesten L, Ahlgren G, Boberg M. 2000. Fatty acids in profundal benthic invertebrates and their major food resources in Lake Erken, Sweden: seasonal variation and trophic indications. Can J Fish Aquat Sci. 57(11):2267–2279.
- Gonçalves A, Azeiteiro U, Pardal M, De Troch M. 2012. Fatty acid profiling reveals seasonal and spatial shifts in zooplankton diet in a temperate estuary. Estuarine Coastal Shelf Sci. 109:70–80.
- Guo F, Bunn SE, Brett MT, Kainz MJ. 2017. Polyunsaturated fatty acids in stream food webs – high dissimilarity among producers and consumers. Freshw Biol. 62(8):1325–1334.
- Hayakawa K, Tsujimura S, Napolitano GE, Nakano S-i, Kumagai M, Nakajima T, Jiao C. 2002. Fatty acid composition as an indicator of physiological condition of the cyanobacterium Microcystis aeruginosa. Limnology. 3:29–35.
- Horne AC, Kaur S, Szemis JM, Costa AM, Nathan R, Webb JA, Stewardson MJ, Boland N. 2018. Active management of environmental water to improve ecological outcomes. J Water Resour Plann Manage. 144(12):04018079.
- Iverson SJ, Field C, Don Bowen W, Blanchard W. 2004. Quantitative fatty acid signature analysis: a new method of estimating predator diets. Ecol Monogr. 74(2):211–235.
- Jenkins KM, Boulton AJ, Ryder DS. 2005. A common parched future? Research and management of Australian arid-zone floodplain wetlands. Hydrobiologia. 552(1):57–73.
- Johns R, Nichols P, Perry GJP. 1979. Fatty acid composition of ten marine algae from Australian waters. Phytochemistry. 18(5):799–802.
- Kainz M, Arts MT, Mazumder A. 2004. Essential fatty acids in the planktonic food web and their ecological role for higher trophic levels. Limnol Oceanogr. 49(5):1784–1793.
- Kelly JR, Scheibling RE. 2012. Fatty acids as dietary tracers in benthic food webs. Mar Ecol Prog Ser. 446:1–22.
- King AJ. 2004. Density and distribution of potential prey for larval fish in the main channel of a floodplain river: pelagic versus epibenthic meiofauna. River Res Appl. 20(8):883–897.
- Kingsford RT. 2000. Ecological impacts of dams, water diversions and river management on floodplain wetlands in Australia. Austral Ecol. 25(2):109–127.
- Kohlbach D, Schaafsma FL, Graeve M, Lebreton B, Lange BA, David C, Vortkamp M, Flores H. 2017. Strong linkage of polar cod (Boreogadus saida) to sea ice algae-produced carbon: evidence from stomach content, fatty acid and stable isotope analyses. Prog Oceanogr. 152:62–74.
- Lemly AD, Kingsford RT, Thompson JR. 2000. Irrigated agriculture and wildlife conservation: conflict on a global scale. Environ Manage. 25(5):485–512.
- Lowe WH, Likens GE, Power ME. 2006. Linking scales in stream ecology. BioScience. 56(7):591–597.2.0.CO;2]
- Magnone L, Bessonart M, Gadea J, Salhi M. 2015. Trophic relationships in an estuarine environment: a quantitative fatty acid analysis signature approach. Estuarine Coastal Shelf Sci. 166:24–33.
- Malzahn AM, Aberle N, Clemmesen C, Boersma M. 2007. Nutrient limitation of primary producers affects planktivorous fish condition. Limnol Oceanogr. 52(5):2062–2071.
- Manly B. 1974. A model for certain types of selection experiments. Biometrics. 30(2):281–294.
- Masclaux H, Bec A, Kainz MJ, Perriere F, Desvilettes C, Bourdier G. 2012. Accumulation of polyunsaturated fatty acids by cladocerans: effects of taxonomy, temperature and food. Freshwater Biol. 57(4):696–703.
- Meredith SN, Matveev VF, Mayes P. 2003. Spatial and temporal variability in the distribution and diet of the gudgeon (Eleotridae: Hypseleotris spp.) in a subtropical Australian reservoir. Mar Freshwater Res. 54(8):1009–1017.
- Meziane T, Tsuchiya M. 2000. Fatty acids as tracers of organic matter in the sediment and food web of a mangrove/intertidal flat ecosystem, Okinawa, Japan. Mar Ecol Prog Ser. 200:49–57.
- Murray-Darling Basin Authority. 2011a. Delivering a healthy working basin: about the draft basin plan. Canberra: Murray-Darling Basin Authority.
- Murray-Darling Basin Authority. 2011b. The proposed ‘environmentally sustainable level of take’ for surface water of the Murray-Darling Basin: methods and outcomes. Canberra: MDBA.
- Napolitano GE. 1999. Fatty acids as trophic and chemical markers in freshwater ecosystems. In: Arts MT, Wainman BC, editors. Lipids in freshwater ecosystems. New York, NY: Springer; p. 21–44.
- Ning NS, Nielsen DL, Hillman TJ, Suter PJ. 2010. The influence of planktivorous fish on zooplankton communities in riverine slackwaters. Freshwater Biol. 55(2):360–374.
- O'Connell M, Baldwin DS, Robertson AI, Rees G. 2000. Release and bioavailability of dissolved organic matter from floodplain litter: influence of origin and oxygen levels. Freshwater Biol. 45(3):333–342.
- Oberg EW, Fuiman LA. 2015. Linking fatty acids in the diet and tissues to quality of larval southern flounder (Paralichthys lethostigma). J Exp Mar Biol Ecol. 467:7–15.
- Paulsen M, Hammer C, Malzahn AM, Polte P, Von Dorrien C, Clemmesen C. 2014. Nutritional situation for larval Atlantic herring (Clupea harengus L.) in two nursery areas in the western Baltic Sea. ICES J Mar Sci. 71(4):991–1000.
- Peltomaa ET, Aalto SL, Vuorio KM, Taipale SJ. 2017. The importance of phytoplankton biomolecule availability for secondary production. Front Ecol Evol. 5:128.
- Poff NL, Zimmerman JKH. 2010. Ecological responses to altered flow regimes: a literature review to inform the science and management of environmental flows. Freshwater Biol. 55:194–205.
- Ravinet M, Syväranta J, Jones RI, Grey J. 2010. A trophic pathway from biogenic methane supports fish biomass in a temperate lake ecosystem. Oikos. 119(2):409–416.
- Robson BJ, Lester RE, Baldwin DS, Bond NR, Drouart R, Rolls RJ, Ryder DS, Thompson RM. 2017. Modelling food-web mediated effects of hydrological variability and environmental flows. Water Res. 124:108–128.
- Rolls RJ, Baldwin DS, Bond NR, Lester RE, Robson BJ, Ryder DS, Thompson RM, Watson GA. 2017. A framework for evaluating food-web responses to hydrological manipulations in riverine systems. J Environ Manage. 203:136–150.
- Rossi S, Sabatés A, Latasa M, Reyes E. 2006. Lipid biomarkers and trophic linkages between phytoplankton, zooplankton and anchovy (Engraulis encrasicolus) larvae in the NW Mediterranean. J Plankton Res. 28(6):551–562.
- Ryder DS, Watts RJ, Nye E, Burns A. 2006. Can flow velocity regulate epixylic biofilm structure in a regulated floodplain river? Mar Freshwater Res. 57(1):29–36.
- Sheldon F, Walker KF. 1997. Changes in biofilms induced by flow regulation could explain extinctions of aquatic snails in the lower River Murray, Australia. Hydrobiologia. 347:97–108.
- Southwell M, Wilson G, Ryder D, Sparks P, Thoms M. 2015. Monitoring the ecological response of Commonwealth Environmental Water delivered in 2013-14 in the Gwydir River system. A report to the Department of Environment. Armidale: University of New England.
- Stoffels RJ, Humphries P. 2003. Ontogenetic variation in the diurnal food and habitat associations of an endemic and an exotic fish in floodplain ponds: consequences for niche partitioning. Environ Biol Fishes. 66(3):293–305.
- Taipale SJ, Brett MT, Pulkkinen K, Kainz MJ. 2012. The influence of bacteria-dominated diets on Daphnia magna somatic growth, reproduction, and lipid composition. FEMS Microbiol Ecol. 82(1):50–62.
- Thompson R, Bond N, Poff N, Byron N 2019. Towards a systems approach for river basin management—lessons from Australia’s largest river. River Res Appl. 35(5):466–475.
- Tonkin JD, Poff NL, Bond NR, Horne A, Merritt DM, Reynolds LV, Olden JD, Ruhi A, Lytle DA. 2019. Prepare river ecosystems for an uncertain future. Nat Publish Group. 570(7761):301–303.
- Vestal JR, White DC. 1989. Lipid analysis in microbial ecology. BioScience. 39(8):535–541.
- Volkman J, Jeffrey S, Nichols P, Rogers G, Garland C. 1989. Fatty acid and lipid composition of 10 species of microalgae used in mariculture. J Exp Mar Biol Ecol. 128(3):219–240.
- Vorosmarty CJ, McIntyre PB, Gessner MO, Dudgeon D, Prusevich A, Green P, Glidden S, Bunn SE, Sullivan CA, Liermann CR, et al. 2010. Global threats to human water security and river biodiversity. Nature. 467(7315):555–561.
- Wakeham SG. 1995. Lipid biomarkers for heterotrophic alteration of suspended particulate organic matter in oxygenated and anoxic water columns of the ocean. Deep Sea Res Part I. 42(10):1749–1771.
- Whipple AA, Viers JH. 2019. Coupling landscapes and river flows to restore highly modified rivers. Water Resour Res. 55:4512–4532.
- Willers C, Jansen van Rensburg P, Claassens S. 2015. Phospholipid fatty acid profiling of microbial communities–a review of interpretations and recent applications. J Appl Microbiol. 119(5):1207–1218.
- Williams DD, Heeg N, Magnusson AK. 2007. Habitat background selection by colonizing intermittent pond invertebrates. Hydrobiologia. 592(1):487–498.
- Williamson N, Kobayashi T, Outhet D, Bowling LC. 2018. Survival of cyanobacteria in rivers following their release in water from large headwater reservoirs. Harmful Algae. 75:1–15.
- Wolfenden BJ, Wassens SM, Jenkins KM, Baldwin DS, Kobayashi T, Maguire J. 2018. Adaptive management of return flows: lessons from a case study in environmental water delivery to a floodplain river. Environ Manage. 61(3):481–496.
- Xu X, Li W, Fujibayashi M, Nomura M, Sakamaki T, Nishimura O, Li X. 2014. Feedback of threshold via estimating sources and composition of sedimentary organic matter across trophic gradients in freshwater lakes. Sci Total Environ. 500-501:373–382.