Abstract
An instrument that collects cloud droplets one by one was designed to measure the fraction of cloud droplets containing mineral particles. Sampling was performed in the artificial cloud experimental system (ACES) established in the vertical shaft of an abandoned mine. The collected droplets were observed with a scanning electron microscope (SEM), and their constituents were analyzed by an energy dispersive X-ray analyzer (EDX). The ratio of droplets including mineral particles was above 50%, reflecting the high concentration of mineral particles at the bottom of the shaft. When small droplets were sprayed by an industrial fog generator at the bottom of the shaft, the fraction of cloud droplets with mineral particles decreased but remained above 20%. This result suggests that mineral particles in the free atmosphere may also act as cloud condensation nuclei and be incorporated into cloud droplets at the early stage of cloud formation. Significant influences on the climate model, biogeochemistry, and atmospheric chemistry should be considered.
INTRODUCTION
Aqueous solutions of droplets in the free atmosphere are not as simple to describe as bulk liquids in laboratories. One of the main reasons is that each droplet contains a variety of substances reflecting the various constituents of atmospheric aerosol particles that may have acted as cloud condensation nuclei. When water vapor is supersaturated in the free atmosphere, hygroscopic aerosol particles such as sodium chloride (NaCl) and ammonium sulfate ((NH4)2SO4) act as cloud condensation nuclei (CCN) on which water vapor condenses to form cloud droplets (CitationMason 1971; CitationPrupaccher and Klett 1980). Therefore the chemical constituents of cloud droplets primarily depend on the CCN. Further modifications may occur afterwards due to the uptake of gases and aerosol particles and the chemical reactions occurring between chemical species in the droplets. These chemical species in cloud droplets exist mainly as ions, and this has led researchers to conduct intensive analyses of the ionic components in cloud droplets in field observations (CitationHoughton 1955; CitationOkita 1968; CitationPetrenchuk and Selezneva 1970; CitationBrewer et al. 1983; CitationMunger et al. 1983; CitationJacob et al. 1985; CitationHegg and Hobbs 1986; CitationDaum et al. 1987; CitationFacchini et al. 1992).
Another modification of the aqueous solutions of droplets in the atmosphere derives from the drastic changes in the concentrations of solute during the formation and dissipation of cloud droplets. At the start, the concentration of the salt which acts as a CCN in a cloud droplet is extremely high. A very similar situation occurs during the dissipation of a cloud droplet, which results in the formation of one or more aerosol particles.
Due to this variety of concentrations and constituents in cloud droplets, atmospheric researchers have encountered difficulties in a systematic understanding of aqueous reactions in the atmosphere, although the occurrence of aqueous phase reactions in the atmosphere has been established (CitationHegg and Hobbs 1982; CitationEatough et al. 1984; CitationBizhak et al. 1986; CitationHusain et al. 1991; CitationQian et al. 1992; CitationPandis et al. 1992; CitationLaj et al. 1997). To interpret the aqueous phase reactions it is necessary to establish a technique to sample and analyze water droplets in the atmosphere as they occur. For this purpose all droplets must be analyzed one by one without averaging because droplets may have different chemical constituents, as mentioned above. CitationTenberken and Baechmann (1998) reported this kind of research by analyzing the ionic components of single cloud droplets by capillary electrophoresis. The focus of that report is on the influence of droplet size on the concentrations of ionic components in each droplet. Therefore insoluble components such as mineral components were not considered, although the significant roles of mineral particles in the free atmosphere have been pointed out by other researchers. Mineral particles contain various elements and supply these to the cloud droplets. Some act as catalysts for the reactions in the aqueous solution of cloud droplets (CitationBrimblecombe and Speddingin 1974; CitationBarrie and Georgii 1976; CitationPenkett et al. 1979; CitationKaplan et al. 1981; CitationMartin 1984; CitationGraedel and Weschler 1981) while others act as nutrients for plankton in the ocean (CitationDuce 1986; CitationMartin and Fitzwater 1988; CitationZhuang et al. 1992).
This investigation presents a technique for sampling and analyzing cloud droplets one by one, including an analysis of insoluble components, and reports results obtained with the technique for observations in the Artificial Cloud Experiment System (ACES) established in a vertical shaft of an abandoned mine (CitationYamagata et al. 1998, CitationUtiyama et al. 2001).
EXPERIMENTAL
Sampling and Analysis of Cloud Droplets
A schematic diagram of the sampling instrument is shown in . The principle of this sampler is that of an impactor. Cloud droplets from the atmosphere are introduced into an impactor through an inlet, a 0.5 mm diameter opening, then inertially impact with the polytetrafuloroetylene (PTFE) belt facing the inlet opening. The flow rate of the sampled air was about 8 mL min−1, which makes the 50% cutoff diameter of this sampler 5 μ m. The PTFE belt is coated with candle soot to establish droplet traces clearly. The PTFE belt is driven by a roller with an electric motor powered by batteries. At the same time a gear shifts the table of the PTFE belt as a whole along the roller width, and the trace of droplets will not overlap but ideally result in a spiral train. After the sampling, PTFE belts were removed and preserved in an airtight box. They were brought back to the laboratory and observed with a scanning electron microscope (SEM; Hitachi, S3200N) and analyzed with an energy dispersive X-ray analyzer (EDX; Hitachi, Kevex SIGMA). The analysis by SEM equipped with EDX is a unique technique with which we can analyze whole constituents for each CCN in the trace of a droplet. However, the analysis by SEM with EDX has some defects. For example, their information is limited to the elemental, and we are not able to obtain information about chemical species. Moreover during observation and analyses by SEM with EDX the atmosphere around the sample needs to be evacuated, and this requirement creates artifacts in the sample, especially for volatile species such as NH4 + or NO3 −.
Simultaneous sampling of cloud water was performed with a string cloud water sampler (Usui industry, WRI-2). The impaction efficiency of a droplet with 7 μ m diameter, which was most frequently observed at the sampling site, was calculated to be around 70% (CitationMay and Clifford 1967). Cloud water was sampled every 15 min into plastic bottles. These were analyzed for the ionic components in the laboratory with an ion chromatograph (Dionex DX500; column: AS12A, CS12A). The concentration of carbonate was measured with a different ion chromatograph (Dionex100; column: IonPac ICE-AS1).
Artificial Cloud Experimental System
Sampling of cloud droplets was repeated for 1 h intervals during 11–14 November 1997 at 680 m ASL in the ACES, i.e., the Nippo-central shaft of the Kamaishi Mining Co. in Kamigo-machi Tono city Iwate Pref., Japan (). Details of the ACES are also shown in . The electric fan at the top of the shaft (680 m) creates an ascending air flow of several tenth m s−1, which causes a subadiabatic expansion of air and cloud formation. The number concentrations of the cloud droplet at the top were reported to be around 100 cm−3 with peak of droplet size at 7 μ m. The temperatures in the ACES were 12.6°C at the bottom (250 m ASL) and 11.1°C at the top. The relative humidity at the bottom is nearly 100% due to the groundwater in the tunnels. These parameters are stable owing to the large heat capacity of the rock surrounding the shaft. Although the cloud base during this study was not confirmed, it was estimated to be about 30 m above the bottom of the shaft according to preceding experiments in September 1996 when the experimental conditions were similar. Some of the aerosol particles at the bottom of the shaft may be derived from the compounds present in the mining tunnel, while others may have flown into the tunnel from the outside through an inlet at 250 m ASL about 6.4 km from the shaft. This inlet is about 20 km from the coast of the Pacific Ocean, and easterly winds carry air from the ocean along the valley to the inlet of the shaft, as shown in .
Spraying Small Droplets at the Bottom
Small droplets of aqueous solution of sodium chloride (200 g L−1) were sprayed for a comparison with the background conditions. The small droplets were generated by an industrial fog supply system (Ikeuchi, AKIMist D) with an air compressor (Annest IWATA Handy COMPAC, 750W). The amount of solution supplied as fog droplets was about 2 L h−1. Some fraction of droplets were deposited on the ground before they entered into the vertical shaft. The mean diameter of the fog droplets at the exit of the fog generator was around 10 μ m. Some of these do not dissipate and remain as droplets due to the high relative humidity in the tunnel, although much smaller droplets than the droplets around 10 μ m may have dissipated to produce small aerosol particles. Spraying continued 90 min, and all kinds of cloud-droplet sampling were performed during this period.
RESULTS AND DISCUSSION
Background Droplets
shows examples of SEM images of the trace of cloud droplets, which had impacted on the soot film, sampled in the ACES. The soot film is the background and appears spongelike, while the impacts of cloud droplets create darker areas inside which the particles appear. When particles appear inside the trace of a droplet it may be interpreted in two ways: the particles were inside the droplets in the atmosphere, or the particles were outside the cloud droplets in the atmosphere but impacted at the position of the droplet just after/before the droplet's impaction. The probability of the latter is low according to the working principle of the impactor. It is designed to collect particles larger than 5 μ m, and the collection efficiency for particles with diameters smaller than 5μ m—the order of particles commonly observed in this study—is very low. Further, very few mineral particles impacted on the soot, but outside the trace of droplets support this. This suggests that free mineral particles which were not contained in cloud droplets seldom impacted at the same position as cloud droplets, and it was concluded that the particles observed inside droplet traces were already included in the droplets in the atmosphere.
FIG. 3 SEM images of traces of cloud droplets sampled in ACES. The bars in the images are 50 μ m (left) and 20 μ m (right).
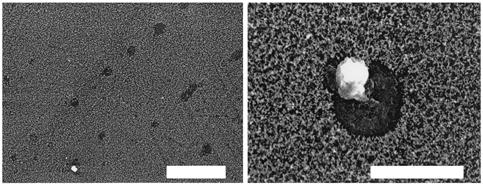
These particles inside the droplets were analyzed with the EDX and categorized as follows: sea salt (Na, Cl), sea salt and mineral (Cl, Al, Si, Ca), and mineral (Al, Si, Ca). The major particles observed in the droplets were minerals which may have originated from the tunnel, although some may have come from outside the tunnel. We had no means to distinguish the origins of mineral particles. The sodium chloride in the cloud droplets may come from sea salt transported from the coast. In about 10% of the droplet traces, the X-ray analyses failed to identify the elements in the droplet, although it was apparent that these droplets contained particles. The ratio of each particle category except the droplet with unidentified particles is shown in , which shows that more than half the droplets contain mineral particles. This result may significantly influence a wide range of environmental phenomena.
Chemical reactions in cloud droplets are influenced by mineral particles. Mineral particles contain alkaline or alkaline-earth metal such as Na, K, Ca, and Mg, which neutralize acidic compounds and maintain high pH values in the cloud droplets. The pH of aqueous solutions is a significant parameter that strongly influences the reactions in droplets. Transition metals in mineral particles also dissolve into the aqueous phase and act as catalysts for many reactions, including sulfur dioxide oxidation. The mineral particles themselves may also act as heterogeneous catalysts/photocatalysts (CitationYamagata et al. 1994).
Another influence of mineral particles in cloud droplets is the influence on ecosystems in the ocean. In remote ocean areas essential minerals are believed to be transported by the atmosphere in the form of aerosol particles. Weathering by chemical reactions in cloud droplets during transportation from land to ocean may induce or accelerate the dissolution of many kinds of essential nutrients available to the plankton and other marine organisms.
Simultaneous bulk measurements of ionic components in droplets have shown that the major anion was carbonate and the major cation was calcium (). Other ions such as sodium, ammonia, chloride, and sulfate were also detected, but at much lower instances than calcium and carbonate. The concentrations of all these ions were in the order of several mg L−1, which is similar to or relatively lower than that observed in fog water collected on the ground around this area (CitationAdzuhata 2001). The low concentration of ion species may be due to the dry deposition of aerosol particles during the transport from the air-inlet of the tunnel system to the bottom of the vertical shaft.
FIG. 5 Concentrations of ionic components in cloud water analyzed by bulk analyses. BG, background; NaCl containing, samples during the NaCl solution spraying. The abscissa is logarithmic.
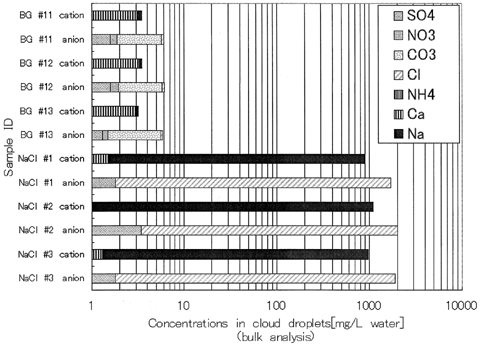
Calcium carbonate, the major chemical species in droplet, is the only product which Kamaishi Mining Co. yielded during this experiment. It may have approached to the vertical shaft through its bottom or some other tunnels connected to the shaft at different altitudes. Calcium carbonate is common species in mineral dusts from arid areas such as North China, Sahara Desert, etc., and it may contribute to the high concentration of Ca in rain water (CitationIchikuni 1978). Therefore the presence of CaCO3 in ACES may not have significantly altered the microphysical conditions around cloud droplets from those in the atmosphere.
Droplets Observed During Mist Generation at the Bottom of ACES
When spraying an aqueous sodium chloride solution (200 g L−1), the cloud at the top of the shaft became dense and the bulk concentrations of chemical species in droplets changed, as shown in . The increase in the NaCl ratios in droplets indicates that some of the small droplets produced at the bottom moved to the top of the ACES. On the other hand 40% of cloud droplets still contained mineral particles, although the bulk analysis of the cloud droplets showed that the concentration of NaCl was overwhelmingly larger than the other ionic components during the spraying, as shown in . Here 26% of cloud droplets contained only mineral particles and undetectable sprayed salt, i.e., sodium chloride. This result provides insight into the incorporation processes of mineral particles into cloud droplets. If the incorporation of airborne mineral particles takes place only after the droplet formation through collisions of droplets with mineral particles due to faster falling velocities of droplets than those of mineral particles, all droplets would contain sodium chloride regardless of the mineral-particle inclusion. Furthermore, the fraction of droplets with mineral particles would be same as one observed in background condition, i.e., without spraying NaCl solution because mineral particles have no effect on the cloud droplet formation.
The contribution of this collision process to the incorporation is estimated as follows. First we assume that mineral particles distribute in the vertical shaft homogeneously and that gravitational sedimentation is the only process to incorporate mineral particles, into the droplet. Then collection rate of mineral particles by a cloud droplet can be expressed by following equation:
Based on these considerations it might be concluded that the mineral particles acted as CCN. There are various components in the mineral particles which are soluble in water and cause mineral particles to function as CCN. One such component is salts such as calcium carbonate, an original component of the particles; another is the salt which is produced in the atmosphere by reactions between mineral components and atmospheric constituents. Although the technique presented here is not adequate to answer which part of a particle acted as the cloud condensation nucleus, the results here provide evidence of the presence of mixtures of mineral particles and salt that become attached in the atmosphere. Once cloud droplets also include mineral particles, particles comprising both mineral and salt would simply form, as has been observed in the atmosphere (CitationAndreae et al. 1986). Another estimation supporting this is that cloud droplets often evaporate before they grow sufficiently enough to precipitate (CitationHobbs 1993).
SUMMARY
A novel cloud-droplet impactor was designed and its performance examined in ACES. The impactor showed excellent results, indicating that insoluble particles appeared inside droplets. The process of mineral-particle incorporation into cloud droplets and the significance of the incorporation were discussed.
Acknowledgments
This research is supported by a Grant-in-Aid for Encouragement of Young Scientists of MEXT Japan 9770474.
REFERENCES
- Adzuhata , T. , Inotsume , J. , Okamura , T. , Kikuchi , R. , Ozeki , T. , Kajikawa , M. and Ogawa , N. 2001 . Evaluation of Ionic Pollutants of Acid Fog and Rain Using a Factor Analysis and Back Trajectories . Anal. Sci. , 17 : 71 – 76 .
- Andreae , M. O. , Charlson , R. J. , Bruynseels , F. , Storms , H. , Van Grieken , R. and Maenhaut , W. 1986 . Internal Mixture of Sea Salt, Silicates, and Excess Sulfate in Marine Aerosols . Science , 23 : 21620 – 21623 .
- Barrie , L. and Georgii , H. W. 1976 . An Experimental Investigation of the Absorption of Sulphur Dioxide by Water Drops Containing Heavy Metal Ions . Atmos. Environ. , 10 : 743 – 749 .
- Bizhak , M. , Hudnik , V. , Hansen , A. D. H. and Novakov , T. 1986 . Evidence for Heterogeneous SO2 Oxidation in Ljubljana, Yugoslavia . Atmos. Environ. , 20 : 2199 – 2204 .
- Brewer , R. L. , Gordon , R. J. , Shepard , L. S. and Ellis , E. C. 1983 . Chemistry of Mist and Fog from the Los Angeles Urban Area . Atmos. Environ. , 17 : 2267 – 2270 .
- Brimblecombe , P. and Speddingin , D. J. 1974 . The Catalytic Oxidation of Micromolar Aqueous Sulphur Dioxide-I . Atmos. Environ. , 8 : 937 – 945 .
- Daum , P. H. , Kelly , T. J. , Strapp , J. W. , Leaitch , W. R. , Joe , P. , Schemenauer , R. S. , Isaac , G. A. , Anlauf , K. G. and Wiebe , H. A. 1987 . Chemistry and Physics of a Winter Stratus Cloud Layer: A Case Study . J. Geophys. Res. , 92 : 8426 – 8436 .
- Duce , R. A. 1986 . The Role of Air–Sea Exchange in Geochemical Cycling , Edited by: Buat-Menard , D. pp. 497 – 529 . Dordrecht : Reudel Publishing .
- Eatough , D. J. , Arthur , R. J. , Eatough , N. L. , Hill , M. W. , Mangelson , N. F. , Richter , B. E. and Hansen , L. D. 1984 . Rapid Conversion of SO2 to Sulfate in a Fog Bank . Envrion. Sci. Technol. , 18 : 855 – 859 .
- Facchini , M. C. , Fuzzi , S. , Kessel , M. , Wobrock , W. , Jaeschke , W. , Arends , B. G. , Moels , J. J. , Berner , A. , Solly , I. , Kruisz , C. , Reischl , G. , Pahl , S. , Hallberg , A. , Ogren , J. A. , Fierlinger-Berlinninger , H. and Marzorati , A. 1992 . The Chemistry of Sulfur and Nitrogen Species in a Fog System a Multiphase Approach . Tellus , 44B : 505 – 521 .
- Graedel , T. E. and Weschler , C. J. 1981 . Chemistry within Aqueous Atmospheric Aerosols and Raindrops . Rev. Geophys. Space Phys. , 19 : 505 – 539 .
- Hegg , D. A. and Hobbs , P. V. 1982 . Measurements of Sulfate Production in Natural Clouds . Atmos. Environ. , 16 : 2663 – 2668 .
- Hegg , D. A. and Hobbs , P. B. 1986 . Sulfate and Nitrate Chemistry in Cumuliform Clouds . Atmos. Environ. , 20 : 901 – 909 .
- Hobbs , P. V. 1993 . Aerosol-Cloud-Climate Interactions , p. 11 New York : Academic Press .
- Houghton , H. J. 1955 . On the Chemical Composition of Fog and Cloud Water . J. Meteorol. , 12 : 355 – 357 .
- Husain , L. , Dutkiewicz , V. A. , Hussain , M. M. , Khwaja , H. A. , Burkhard , E. G. , Mehmood , G. , Parekh , P. P. and Canelli , E. 1991 . A Study of Heterogeneous Oxidation of SO2 in Summer Clouds . J. Geophys. Res. , 96 : 18789 – 18806 .
- Ichikuni , M. 1978 . Calcite as a Source of Excess Calcium in Rainwater . J. Geophys. Res. , 83 : 6249 – 6252 .
- Jacob , D. J. , Waldman , J. M. , Munger , J. W. and Hoffmann , M. R. 1985 . Chemical Composition of Fogwater Collected Along the California Coast . Environ. Sci. Technol. , 19 : 730 – 736 .
- Kaplan , D. J. , Himmelblau , D. M. and Kanaoka , C. 1981 . Oxidation of Sulfur Dioxide in Aqueous Ammonium Sulfate Aerosols Containing Manganese as a Catalyst . Atmos. Environ. , 15 : 763 – 773 .
- Laj , P. , Fuzzi , S. , Facchini , M. C. , Orsi , G. , Berner , A. , Kruisz , C. , Wobrock , W. , Hallberg , A. , Bower , K. N. , Gallagher , M. W. , Beswick , K. M. , Colvile , R. N. , Choularton , T. W. , Nason , P. and Jones , B. 1997 . Experimental Evidence for In-cloud Production of Aerosol Sulphate . Atmos. Environ. , 31 : 2503 – 2514 .
- Martin , J. H. and Fitzwater , S. E. 1988 . Iron Deficiency Limits Phytoplankton Growth in the North-east Pacific Subarctic . Nature , 331 : 341 – 343 .
- Martin , L. R. 1984 . “ Kinetic Studies of Sulfite Oxidation in Aqueous Solution ” . In SO2, NO and NO2 Oxidation Mechanisms: Atmospheric Considerations , Edited by: Calvert , J. G. pp. 63 – 100 . Boston : Butter Worth Publishers .
- Mason , B. J. 1971 . The Physics of Clouds , London : Oxford Univ. Press .
- May , K. R. and Clifford , R. 1967 . The Impaction of Aerosol Particles on Cylinders, Spheres, Ribbons and Discs . Ann. Occup. Hyg. , 10 : 83 – 95 .
- Munger , J. W. , Jacob , D. J. , Waldman , J. M. and Hoffmann , M. R. 1983 . Fog Chemistry in an Urban Atmosphere . J. Geophys. Res. , 88 : 5109 – 5121 .
- Okita , T. 1968 . Concentration of Sulfate and Other Inorganic Materials in Fog and Cloud Water and in Aerosol . J. Meteorological Soc. Japan , 46 : 120 – 127 .
- Pandis , S. N. , Seinfeld , J. H. and Pilinis , C. 1992 . Heterogeneous Sulfate Production in an Urban Fog . Atmos. Environ. , 26(A) : 2509 – 2522 .
- Penkett , S. A. , Jones , M. R. and Eggleton , A. E. J. 1979 . A Study of SO2 Oxidation in Stored Rainwater Samples . Atmos. Environ. , 13 : 139 – 147 .
- Petrenchuk , O. P. and Selezneva , E. S. 1970 . Chemical Composition of Precipitation in Regions of the Soviet Union . J. Geophys. Res. , 75 : 3629 – 3634 .
- Prupaccher , H. R. and Klett , J. D. 1980 . Microphysics of Clouds and Precipitation , pp. 225 – 240 . Dordrecht, , Holland : D. Reidel Publishing C. .
- Qian , G.-W. , Ishizaka , Y. , Minami , Y. , Kurahashi , Y. and Tjandradewi , B. I. 1992 . Transformation of Individual Aerosol Particles in Acidic Fog Evolution . J. Meteorological Soc. Japan , 70 : 711 – 721 .
- Seinfeld , J. H. and Pandis , S. N. 1998 . Atmospheric Chemistry and Physics—From Air Pollution to Climate Change , New York : John-Wiley & Sons, Inc. .
- Tenberken , B. and Baechmann , K. 1998 . Sampling and Analysis of Single Cloud and Fog Drops . Atmos. Environ. , 32 : 1757 – 1763 .
- Utiyama , M. , Fukuyam , T. , Yamagata , S. , Ohta , S. , Izumi , K. , Harimaya , T. , Fujiyoshi , Y. , Yamada , T. and Inage , M. 2001 . Rate of Sulfur Dioxide Removal Artificial Cloud Experiments Utilizing a Long Vertical Shaft . Water Air Soil Pollution , 130 : 325 – 330 .
- Yamagata , S. , Baba , S. , Murao , N. , Ohta , S. , Fukuyama , T. , Utiyama , M. , Yamada , T. , Fujiyoshi , Y. , Harimaya and Inage , M. 1998 . Real Scale Experiment of Sulfur Dioxide Dissolution into Cloud Droplets Generated in Artificial Cloud Experimental System (ACES) . J. Global Environ. Eng. , 4 : 53 – 63 .
- Yamagata , S. , Nishijo , M. , Murao , N. , Ohta , S. and Mizoguchi , I. 1994 . Photocatalytic Oxidation of Sulfite to Sulfate in the Presence of Yellow Sand . Denki Kagaku , 62 : 257 – 259 .
- Zhuang , G. , Zhen , Y. , Duce , R. A. and Brown , P. R. 1992 . Link Between Iron and Sulphur Cycles Suggested by Detection of Fe(II) in Remote Marine Aerosols . Nature , 355 : 537 – 539 .