Factors influencing the delivery efficiency from HFA-134a MDIs were examined theoretically and experimentally. The time required for evaporation of HFA-134a and ethanol droplets were theoretically calculated. HFA-134a droplets were shown to evaporate approximately seven times faster than ethanol droplets of the same size, even though HFA-134a droplets cool to approximately 78 degrees below ambient temperature during evaporation. MDI delivery efficiency was experimentally shown to decrease with increasing ethanol concentration, however, the corresponding decrease in vapor pressure was not the primary variable responsible for the decreased efficiency. Rather, this was shown to be primarily due to the increased time required for the droplets to evaporate as ethanol concentration increased. Droplets that evaporate slowly remain for a longer period in the size range that is more likely to deposit via turbulent deposition in the actuator mouthpiece or USP Inlet during cascade impaction tests. Tests with experimental MDIs using alternative cosolvents confirmed that MDI delivery efficiency is much more sensitive to the time required for evaporation of the droplets than on the formulation vapor pressure or the size of the atomized droplets. This indicates that factors affecting the evaporation of an MDI aerosol play a larger role in determining MDI delivery efficiency than do atomization effects.
INTRODUCTION
Metered dose inhalers (MDIs) are widely used for the treatment of lung diseases, such as asthma and chronic obstructive pulmonary disease (CitationRoss and Gabrio 1999). Recently, MDIs have been investigated for treatment of systemic diseases due to the rapid absorption and systemic distribution of drugs delivered to the lung (CitationHirst et al. 2002; CitationKapitza et al. 2003; CitationWilson et al. 2002). Each time an MDI is actuated, a very precise amount of formulation is delivered from a metering valve. The primary component of an MDI formulation is a liquefied propellant that serves as the energy source to atomize the formulation into small droplets. One or more active drugs are either suspended as fine particles or dissolved in the formulation. The formulation may also contain surfactant to prevent aggregation of the drug particles (for suspension formulations), cosolvents (e.g., ethanol) to solubilize the drug or surfactant, and optionally other non-volatile excipients.
Traditionally, MDIs have used chlorofluorocarbon (CFC) propellants in the formulation. However, recently developed MDIs use hydrofluoroalkane (HFA) propellants due to the environmental concerns associated with CFC propellants (CitationMolina and Rowland 1974; CitationCicerone et al. 1974; CitationWofsy et al. 1975). While CFC MDIs were relatively inefficient, typically delivering only about 5 to 30 percent of the drug to the lung (CitationNewman et al. 1982; CitationDolovich 1993), many HFA-MDIs deliver 50% or more of the drug to the lung (CitationLeach et al. 1998; CitationLeach 1999; CitationLeach et al. 2002; CitationStein and Stefely 2003). The improved delivery efficiency achieved with HFA-MDIs is often attributed to the increase in vapor pressure for these formulations (CitationHarnor et al. 1993; CitationBrambilla et al. 1999; CitationSmyth et al. 2002).
A critical parameter affecting the efficiency of MDIs is the aerodynamic particle size distribution of the aerosol delivered to the patient (CitationMorrow 1966; CitationLippmann and Albert 1969; CitationHickey et al. 1996; CitationHarrison et al. 1997; CitationHowarth 2001). The process by which an MDI aerosol is generated is complex and has been studied extensively elsewhere (CitationClark 1991; CitationDunbar et al. 1997a; CitationDunbar et al. 1997b; CitationDalby and Byron 1988; CitationRance 1972, Citation1974). For simplicity, it is helpful to consider the MDI aerosol generation process to consist of two phases: (1) Droplet Formation—Phase I; and (2) Aerosol Maturation—Phase II.
Phase I—Droplet Formation
The key process during the “droplet formation phase” is the atomization of the formulation into small droplets. The mass median diameter (MMD) and geometric standard deviation (GSD) of the initial atomized droplets are important descriptors of the aerosol in Phase I. Critical factors influencing the size distribution of the initial droplets include the vapor pressure and surface tension of the formulation (CitationClark 1991; CitationDalby and Byron 1988) as well as the valve size and actuator orifice diameter (CitationStein and Myrdal 2004). Aerosolization from an MDI is a dynamic process lasting between 50 and 500 milliseconds (CitationClark 1991; CitationGabrio et al. 1999). During this process, the formulation is atomized into millions of droplets. The size distribution of the atomized droplets has been shown theoretically and experimentally to be a function of the formulation and device design (CitationClark 1991; CitationDunbar et al. 1997a; CitationDunbar et al. 1997b; CitationStein and Myrdal 2004).
Phase II—Aerosol Maturation
The key process during this “aerosol maturation phase” is the evaporation of the volatile and semi-volatile materials. Once the droplets are atomized, the high volatility propellant(s) and semi-volatile cosolvent(s) rapidly evaporate leaving the residual aerosol. If the formulation contains both propellant and a cosolvent (as most solution MDIs do), the evaporation of the propellant occurs more rapidly than that of the cosolvent due to the higher volatility of the propellant as compared to a cosolvent such as ethanol. For solution MDIs that contain both drug and non-volatile excipients, the residual particle usually consists of a matrix of drug and excipient (CitationStefely et al. 2000). For solution MDIs that do not have non-volatile excipients, the residual particles are usually amorphous drug spheres (CitationMcKenzie and Oliver 2000).
The MDI plume is highly dynamic during the aerosol maturation phase. The droplets undergo rapid deceleration from initial velocities of up to 60 m/s (CitationClark 1991; CitationDunbar et al. 1997a) down to a velocity corresponding to the patient's inspiratory airflow (typically on the order of a few m/s). The size of the droplets rapidly decrease as the volatile components of the formulation evaporate (CitationClark 1991; CitationDunbar et al. 1997a). As the high velocity plume interacts with the low velocity surrounding environment, intense turbulence occurs (CitationVersteeg et al. 2000). In this dynamic region of the MDI plume, a portion of the aerosol deposits via impaction or turbulent deposition in the actuator mouthpiece and oropharynx (CitationStein and Gabrio 2000). Both impaction and turbulent deposition are highly size-dependent (CitationMarple and Willeke 1976; CitationLiu and Agarwal 1974) phenomena with the probability of deposition increasing with increasing particle size. Thus, to successfully deposit in the lung, atomized droplets must rapidly evaporate to a size which does not deposit in the turbulent region, but reaches the patient's lung.
The size of the residual aerosol is determined by the size of the initial droplets and the composition of the formulation. The relationship between the size distribution of the residual aerosol and that of the initial droplets has been derived elsewhere (CitationStein and Myrdal 2004). For solution MDIs, this relationship is summarized in Equation (Equation1), where MMADResidual is the mass median aerodynamic diameter of the residual aerosol, MMDInitial is the mass median diameter of the initial atomized droplets, ρInitial is the initial droplet density which is assumed to be the same as the formulation density, (CitationStein and Myrdal 2004), ρResidual is the density of the non-volatile residual particles, and CNV is the weight fraction of the non-volatiles in the formulation. If the drug is the only non-volatile in the formulation, then ρResidual and CNV can be assumed to be the drug density and weight fraction of drug in formulation, respectively (CitationStein and Myrdal 2004).
In order for the drug particles to reach the desired target of the patient's lung, two criteria must be met: (Equation1) the aerodynamic size of the residual particles must be sufficiently small such that they can reach the lung; and (Equation2) the size of the droplets as they pass through the turbulent region of the actuator mouthpiece and oropharynx must be sufficiently small as to avoid deposition. The size of the atomized droplets formed during the droplet formation phase will clearly influence drug delivery efficiency since the initial droplet size distribution influences the ability of the aerosol to fulfill both of these criteria. Additionally, the rate at which the droplet size distribution changes during the aerosol maturation phase will influence drug delivery efficiency with oropharyngeal deposition being more likely for aerosols that evaporate slowly. The objective of this investigation was to study the relative importance of the processes occurring during the droplet formation and aerosol maturation phases on the delivery efficiency of solution MDIs. This was done through in-vitro aerodynamic particle size measurements characterizing the “respirable” portion of the delivered dose. Of particular interest was assessing whether the delivery efficiency was primarily determined by: (Equation1) the initial size of the droplets atomized—Phase I; or (Equation2) the evaporation rate of the droplets after atomization—Phase II. This was investigated through experimental measurements of the size distribution of the residual aerosol, theoretical calculation of the initial droplet size distribution, theoretical calculation of an “intermediate” droplet size distribution, and theoretical calculation of droplet evaporation rates.
EXPERIMENTAL PROCEDURES
Experimental Characterization of MDI Drug Delivery Efficiency
The delivery of experimental HFA-134a solution MDIs was evaluated experimentally using the Andersen Mark II cascade impactor (ACI). The ACI is widely used in the pharmaceutical industry to assess the delivery characteristics of MDI aerosols (CitationMitchell and Nagle 2003). All ACI tests were conducted by actuating the MDI five times into an ACI using a U.S. Pharmacopiea induction port (“USP Inlet”; CitationU.S. Pharmacopeia 2005). The amount of drug deposited on the MDI valve stem, MDI actuator, USP Inlet, and the various stages of the ACI was assessed by rinsing each component with a suitable solvent and analyzing the rinse solution with an appropriate HPLC method (for details on the beclomethasone dipropionate method, see CitationStein and Olson 1997; for details on the albuterol sulfate method, see CitationU.S. Pharmacopeia 2003). Based on the amount of drug deposited on the various stages of the ACI test setup, the size distribution of the emitted spray was calculated. A key output of this testing was the amount of dose likely to reach the lung, often referred to as the “Respirable Dose.” Historically, this has been defined as the amount of drug that deposits on Stages 3 through the Filter of the ACI. These particles have aerodynamic diameters smaller than about 4.7 μ m and have a relatively high probability of depositing in a patient's lung. The “delivery efficiency,” defined as the mass of drug deposited on Stages 3 through the Filter of the ACI divided by the total mass of drug released from the MDI valve, was utilized to evaluate the efficiency of the different formulations. The flowrate was 28.3 1pm for all tests—this is the flowrate that the ACI is designed to operate at.
Measurements for Estimating Initial Droplet Size Distributions Using the 3321 APS
It was hypothesized that delivery efficiency is highly dependent on the time required for droplets to evaporate to residual particles, with droplets that evaporate slowly being more likely to deposit in the USP inlet. In order to estimate the time required for evaporation, it was necessary to have an accurate assessment of the initial droplet size distribution. The 3321 Aerodynamic Particle Size Spectrometer (“APS,” TSI Inc., Shoreview, MN) was used to measure the residual particle size distribution from the various MDI configurations. Equations (Equation1) and (Equation2) were then used to calculate the size distribution of the initial droplets. For these measurements, the MDI was actuated three times into a large volume (∼ 20 liter) chamber coupled to the TSI 3306 Impactor Inlet, which was in turn coupled to the 3321 APS (). The large volume chamber was used to minimize deposition so that the initial droplet size distribution of the entire atomized aerosol could be estimated. Previous work has shown that when size distributions are measured using the USP inlet, a significant portion of the aerosol deposits in the USP inlet and that this deposition is highly size dependent (CitationNaini et al. 2004; CitationStein and Myrdal 2004). Thus, in order to get an unbiased estimate of the initial droplet size distribution, it was desired to minimize droplet deposition prior to measurement (previous results showed that only about five percent of the aerosol deposits on the interior surface of the large volume chamber—CitationStein and Myrdal 2004). The 3306 Impactor Inlet was used to dilute the aerosol measured by the APS (the 3306 Impactor Inlet isokinetically samples approximately 0.2% of the aerosol and sends it to the APS for size measurement). The flowrate through the 3306 Impactor Inlet was set to 28.3 lpm during testing.
FIG. 1 Schematic of the system used to measure the MMADResidual from solution MDIs. The MMADResidual obtained from this system, along with Equations (Equation1) and (Equation2), was used to calculate the initial droplet MMD.
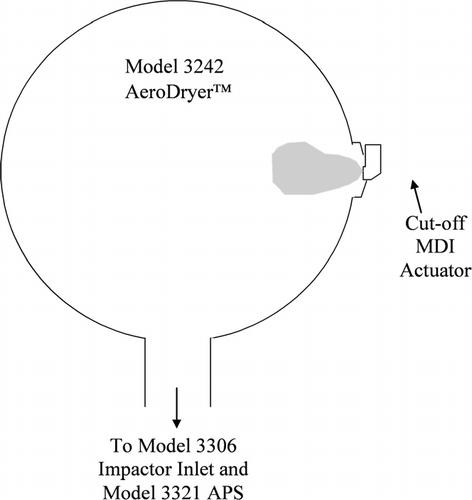
All of the formulations utilized HFA-134a as the propellant and had varying amounts of cosolvent. The formulations contained at least 0.4% non-volatile by weight in order to ensure that the residual particle size distribution was sufficiently large to allow for accurate particle size measurements with the 3321 APS (which has a lower particle size detection limit of approximately 0.5 microns). Once the residual particle size distributions were measured, Equations (Equation1) and (Equation2) were used to calculate the size distribution of the initial atomized droplets. The terms in Equation (Equation1) were rearranged in order to calculate the MMDInitial from the MMADResidual.
The formulations used in the APS size measurements used oligolactic acid (OLA) as a model non-volatile compound. OLA is an excipient used in MDIs to improve the stability of suspension formulations, to increase the solubility of drugs in HFA propellants, or to provide sustained delivery of drug (CitationLeach et al. 2000; CitationStefely et al. 2000; CitationStefely 2002). While OLA is not a drug, it was selected as the non-volatile component for these measurements because of its extraordinary solubility in HFA propellants. This allowed for the non-volatile concentration to be varied independently of the cosolvent level (an exercise that cannot be accomplished with most drugs). For these calculations, the density of OLA was assumed to be 1.25 g/ml.
Experimental Characterization of Performance of MDIs with Varying Vapor Pressures
In order to assess the influence of vapor pressure on the delivery characteristics of solution MDIs, MDI configurations with a wide range of vapor pressures were examined. The vapor pressure of the formulations was modified by varying the ethanol concentration in the formulations from 1 to 20 percent by weight. While the vapor pressure can be modified by varying the ethanol concentration, this allows for only modest changes in vapor pressure of the system. In order to further modify the vapor pressure, the temperature of the MDI canisters and actuators was varied during testing. This permitted a much wider range of vapor pressures to be investigated. Tests were conducted with the MDI canister/actuator at room temperature (approximately 20°C), 35°C, or 45°C. For the tests of at the elevated temperatures, the MDI canisters were primed and rinsed and then placed in a controlled temperature oven for at least one hour. The vials were then removed and the tests were completed within 30 seconds of removal from the oven in order to minimize the cooling of the system.
Description of MDI Configurations Tested
All of the formulations tested in this investigation were experimental HFA-134a solution MDI formulations and utilized 50 microliter SpraymiserTM valves, and QVAR® actuators with an orifice diameter of approximately 0.3 mm. The amount of cosolvent and the non-volatile concentration varied for each set of experiments described in this paper. For simplicity, these formulation details are described in the relevant sections of the Results and Discussion portion of this article.
Theoretical Assessment of Droplet Evaporation Rates
Theoretical expressions describing the evaporation of droplets have been described in detail elsewhere (CitationDavies 1978; CitationHinds 1999). A thorough derivation of these equations is outside the scope of this investigation. However, the equations used for a theoretical calculation of evaporation rates of MDI droplets are described along with the relevant assumptions for the use of these equations. The evaporation rate of a volatile droplet is described by Equations (Equation3)–(Equation6). Equation (Equation3) describes the rate of change of diameter for an evaporating droplet. Equations (Equation4)–(Equation6) describe the terms TD, pD, and λ in Equation (Equation3). This equation assumes that the droplets were evaporating into air free of solvent vapor. This is not true since solvent vapor enters into the surrounding air as the droplets evaporate. However, this is outside the scope of this investigation and was assumed to have a negligible influence on the droplet evaporation.
-
DD—droplet diameter
-
t—time
-
Dv—diffusion coefficient of solvent vapor in air
-
M—molecular weight of solvent
-
R—gas constant
-
ρD—density of solvent in liquid phase
-
pD—vapor pressure of solvent at droplet surface
-
ps—vapor pressure of bulk solvent
-
TD—temperature of droplet
-
T∞—ambient temperature
-
λ—mean free path of solvent gas molecule in air
-
H—latent heat of evaporation of solvent
-
kv—thermal conductivity of solvent vapor
-
γ – surface tension of solvent
-
ram—collision radius of an “air molecule”
-
rsm—collision radius of a solvent molecule
-
N—the number of gas molecules per unit volume of air
Equation (Equation5), sometimes referred to as the Kelvin Equation, describes the vapor pressure of the solvent at the droplet surface and is mostly important as droplet diameter decreases below about 0.1 μ m. Equation (Equation6) describes the mean free path of a solvent vapor molecule. The average radius of an “air molecule” was assumed to be 1.85 Angstroms (CitationHinds 1999). For the evaporation calculations in this article, the average radius of the evaporating solvent molecule was calculated from the pure component molar volume. While there is likely to be some error in these estimates, the significance of these errors on the calculated droplet evaporation rates is minimal. For example, for the ethanol evaporation calculations, a 50% change in mean free path resulted in only a 5% change in droplet lifetime.
Equations (Equation3) through (Equation6) were used to estimate droplet lifetimes (i.e., the time required for complete evaporation). However, a key challenge when using these equations was the fact that many of the variables in the equations are inter-related. For example, the temperature of an evaporating solvent droplet is lower than the ambient temperature due to the latent heat of evaporation of the solvent, which itself is a function of temperature. Thus, the procedure for using these equations was relatively complex. Prior to conducting the evaporation calculations the solvent and propellant physical properties were obtained from the manufacturer or from standard textbooks and reference manuals.
Using Equation (Equation3), the change in droplet size, dDD, for a small increment of time, dt, was determined for a given particle size, DD, through iterative solution of Equations (Equation4) and (Equation5). These equations were iteratively solved due to the inter-related factors in these two equations. This procedure was then repeated over many small time intervals to determine the time required for a droplet to evaporate from a starting droplet size to a final droplet size.
Equations (Equation3)–(Equation6) were used to estimate the droplet lifetimes for pure solvent droplets as well as for droplets with compositions that were relevant to MDI droplets. These equations describe the evaporation of droplets in still air where the heat required for evaporation is balanced with the heat gained from conduction by the warmer surrounding air. In reality, in an MDI plume convective heat transfer also occurs. The movement of the droplets in the extremely complex and dynamic flow conditions of an MDI plume was not considered for these calculations. All of the calculations assumed that the ambient temperature was 20°C, that the concentration of solvent vapor in the air was negligible, and that the presence of a solute in the formulation did not affect the evaporation rate. While it has been shown elsewhere that the presence of a solute can decrease the evaporation rate of a solvent, this depends on the solute and solvent and was outside of the scope of this article.
For the calculations of droplet lifetimes for pure solvents, the droplets were assumed to evaporate to a diameter of zero. For the calculation of droplet lifetimes for MDI formulations, the evaporation was assumed to occur in two steps. In reality, the ethanol and propellant evaporate simultaneously, but in order to simplify the calculations it was assumed that the evaporation occurs in these two discrete stages: (Equation1) evaporation of the propellant as the droplets decrease from the initial droplet diameter to an “intermediate droplet diameter” consisting of the cosolvent and the dissolved drug; and (Equation2) evaporation of the cosolvent as the droplets decrease in size from the intermediate droplet diameter to the residual particle diameter. However, this assumption is reasonable since the cosolvent evaporation will be negligible when the droplet is at the wet bulb temperature of the propellant (i.e., during the first stage of the evaporation process). Once the majority of the propellant has evaporated, the droplet temperature will increase (towards the wet bulb temperature of the cosolvent) and cosolvent evaporation will begin. Equations (Equation7) and (Equation8) were used to calculate the size distribution of the intermediate droplets, where ρIntermediate is the density of the intermediate droplets.
RESULTS AND DISCUSSION
The results and discussion section begins with a theoretical assessment of the evaporation of pure solvent droplets. The two solvents discussed are ethanol and HFA-134a. Then experiments assessing the delivery characteristics of various experimental HFA-134a MDIs with varying amounts of ethanol are then discussed. Then, theoretical droplet evaporation calculations are used to provide insight into the experimental MDI drug delivery results. Lastly, the delivery characteristics of HFA-134a MDIs with a variety of alternative cosolvents are described both theoretically and experimentally.
Theoretical Droplet Evaporation Calculations for Pure Ethanol and HFA-134a Droplets
The time required for complete evaporation of HFA-134a droplet were calculated using Equations (Equation3)–(Equation6). For reference, the HFA-134a properties required for these calculations are listed in . The estimated wet bulb temperature for HFA-134a was calculated to be −58°C. This is similar to the value of −62°C estimated by CitationFinlay (2001).
TABLE 1 Material properties of HFA-134a and ethanol used for the estimation of droplet evaporation rates
The time required for complete evaporation of HFA-134a droplets is plotted as a function of initial droplet diameter in . Similar calculations were done for ethanol droplets using the ethanol properties listed in . The wet bulb temperature for evaporating ethanol droplets was estimated to be −1.3°C. The calculated droplet lifetimes for ethanol droplets is shown in . The predicted droplet lifetimes compare favorably to those reported elsewhere (CitationHinds 1999) as shown in .
FIG. 2 The theoretically calculated time required for complete evaporation of various sized HFA-134a droplets in a 20°C environment.
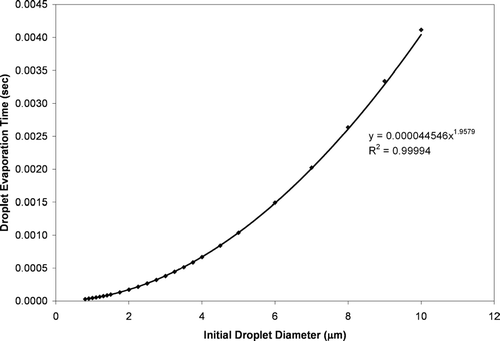
FIG. 3 The theoretically calculated time required for complete evaporation of various sized ethanol droplets in a 20°C environment. The three values represented by squares are previous literature estimates (CitationHinds 1999).
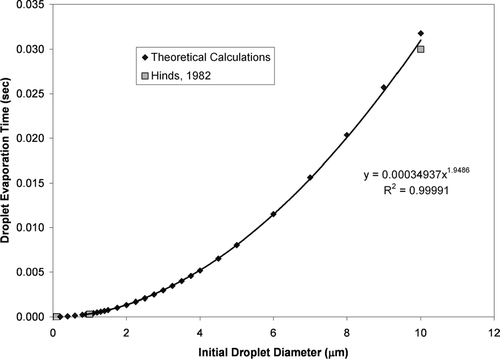
The time required for evaporation of ethanol droplets was substantially longer than for equivalently sized HFA-134a droplets (e.g., a 10 μ m ethanol droplet takes an estimate 0.032 seconds to evaporate compared to 0.0041 seconds for a 10 μ m HFA-134a droplet). In comparison, a 10 micron water droplet takes approximately 0.08 seconds to evaporate in vapor free air, i.e., zero percent relative humidity (CitationHinds 1999). Thus, even though the wet bulb temperature is extremely low for HFA-134a, the evaporation of the propellant in typical MDI formulations will occur much more rapidly than the evaporation of semi-volatile cosolvents such as ethanol or water.
The Influence of Ethanol Concentration on MDI Drug Delivery
The delivery of experimental MDIs with formulations consisting of HFA-134a, 0.017% beclomethasone dipropionate and ethanol concentrations of 1–25% were evaluated using the ACI and the USP Inlet. The results from this study are summarized in and show that delivery efficiency significantly decreased with increasing ethanol concentration. Similar findings have been reported elsewhere (CitationGupta et al. 2003; CitationStein and Myrdal 2004).
Ethanol concentration can influence the delivery efficiency of MDIs in two ways: (1) by changing the ability of the formulation to be atomized into fine droplets (i.e., Phase I effects); and (Equation2) by changing the evaporation rate of these droplets towards their residual particle sizes (i.e., Phase II effects). The theoretical evaporation calculations from the previous section demonstrate that atomized droplets which contain a higher percentage of ethanol will evaporate more slowly than droplets consisting primarily of HFA-134a. As the ethanol concentration increases, the droplets have a higher probability of depositing in the turbulent region and the delivery efficiency will decrease. However, without an understanding of the influence that ethanol concentration has on the initial droplet MMD, it is impossible to determine which of these two effects is most important.
In order to assess the influence of ethanol concentration on the atomization of the formulation into droplets, similar MDI formulations with varying ethanol concentrations (1 to 20%) were investigated using the large volume chamber (). These formulations contained 1% OLA as the non-volatile material to be measured (as opposed to 0.0167% BDP as was used in the formulations from ) in order to provide an aerosol of the optimal particle size for measurement with the APS. Equations (Equation1) and (Equation2) were used along with the MMAD values measured by the system shown in in order to calculate the initial droplet MMD for the various formulations. These results are shown in . These results show that the formulation was atomized into coarser droplets as the ethanol concentrations increased. This may be due to the fact that as ethanol concentration increased, the formulation vapor pressure decreased and there was less energy to atomize the formulation into fine droplets. Additionally, changes in the surface tension or viscosity of the formulation as the ethanol concentration changes may have influenced the atomization process (CitationDalby and Byron 1988)
FIG. 5 The initial droplet MMD from HFA-134a solution MDIs with a range of ethanol concentrations. The MDIs contained 1%w/w OLA in order to provide residual particles of a sufficiently large size to ensure accurate particle sizing.
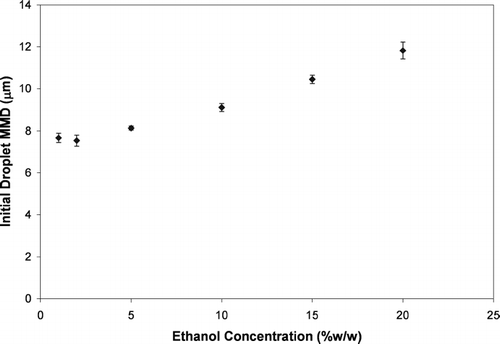
Ethanol concentration influences both the ability of the formulation to be atomized into fine droplets () and the time required for the droplets to evaporate into the smaller residual particles (by inference from and ). Thus both the atomization of the formulation (Phase I effects) and the evaporation rate of the droplets (Phase II effects) appear to be involved in the relationship between delivery efficiency and ethanol concentrations in . The subsequent experiments described in this paper shed light into the relative importance of these two effects in determining MDI delivery efficiency.
The Influence of Phase I (Atomization) Factors on Delivery Efficiency
A difficulty in interpreting the results from the previous section is that ethanol concentration affects both the evaporation rate of the droplets, but also affects key atomization factors such as the formulation vapor pressure. In order to further understand the role that vapor pressure played in the trend observed in , MDIs with a range of vapor pressures were evaluated by testing MDIs with varying ethanol concentrations at various temperatures. The MDIs contained 0.0167% beclomethasone dipropionate as the non-volatile component. The drug delivery from MDIs with 1, 5, 10, and 20% ethanol were tested at formulation temperatures of 20, 35, and 45°C.
The results from ACI testing of these MDI configurations are shown in . As expected based on , the delivery efficiency significantly decreased with increasing ethanol concentration. There was also a slight increase in the delivery efficiency at the elevated testing temperatures. While this influence was statistically significant (p-value < 0.001 from an analysis of variance using general linear model techniques regression of delivery efficiency using ethanol concentration and temperature as the variables), it was much smaller than the influence of ethanol level. This was somewhat surprising since the formulation temperature had a much larger influence on the formulation vapor pressure than does the ethanol concentration.
FIG. 6 The delivery efficiency of HFA-134a solution MDIs with varying ethanol concentrations tested at various formulation temperatures.
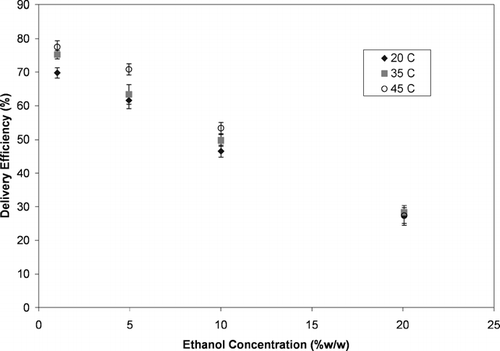
The delivery efficiency from the experiments summarized in is plotted as a function of the formulation vapor pressure in . The formulation vapor pressure was determined for each formulation at each temperature from vapor pressure data provided by the propellant manufacturer and summarized elsewhere (CitationStein and Myrdal 2004). There was a very poor correlation between the delivery efficiency and formulation vapor pressure (Rˆ2 = 0.087 from linear regression with vapor pressure as the sole variable) indicating that the vapor pressure alone explained only a very small amount of the variability in delivery efficiency. When an analysis of covariance on delivery efficiency was conducted (using ethanol concentration and vapor pressure as the variables, with vapor pressure being the covariate), the formulation vapor pressure had a statistically significant influence on the delivery efficiency (p-value < 0.001). However, this influence was much weaker than the influence of ethanol concentration. This indicates that the influence of ethanol concentration on delivery efficiency observed in and was not primarily due to the influence that ethanol concentration has on vapor pressure.
FIG. 7 The delivery efficiency of HFA-134a solution MDIs plotted as a function of formulation vapor pressure. These delivery efficiency results are from the experiments summarized in .
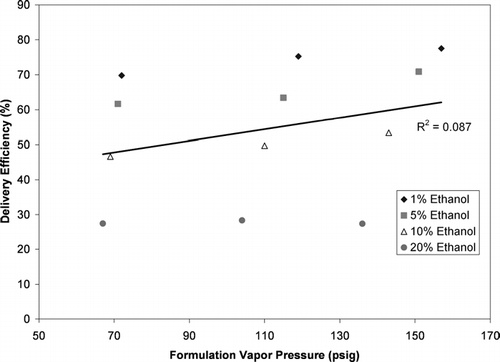
While the vapor pressure of the bulk formulation is important with respect to MDI drug delivery, the pressure inside the MDI expansion chamber is potentially more relevant to drug delivery than the bulk formulation vapor pressure. The pressure inside the MDI expansion chamber was previously measured for each of the configurations from (CitationStein et al. 2005). It was found that the peak pressure in the MDI expansion chamber was highly correlated to (rˆ2 = 0.995), but on average 5% lower the bulk formulation vapor pressure due to slight cooling of the formulation in the expansion chamber during atomization (CitationStein et al. 2005). When the delivery efficiency was plotted as a function of the peak expansion chamber pressure (not shown), the trend was nearly identical to that found in .
The data in indicate that formulation vapor pressure only minimally affected delivery efficiency. In order to further understand these results, it was desired to estimate the initial droplet MMD of these MDI configurations. The initial droplet MMD from these exact formulations could not be measured since the very low non-volatile concentration (0.0167%) resulted in residual particle sizes near the lower range for characterization with the APS. Instead, MDIs were formulated with 1% OLA and the identical ethanol concentrations as the MDIs in and and were tested at the same temperatures (20, 35, and 45°C). The initial droplet MMAD was then determined for these configurations using the apparatus in was estimated and Equations (Equation1) and (Equation2). The initial droplet MMD from these measurements was assumed to be representative of that for the MDIs tested in and since it was previously shown that initial droplet MMD is relatively insensitive to non-volatile concentration (CitationStein and Myrdal 2004).
The average delivery efficiency from the results in is plotted versus the estimated initial droplet MMD and is shown in . The delivery efficiency correlated reasonably well to the initial droplet MMD (Rˆ2 = 0.812 from a regression analysis of delivery dfficiency and initial droplet MMD ignoring ethanol concentration). While there was a reasonable correlation between the delivery efficiency and the initial droplet MMD, much of the unaccounted variability in appears to be related to the ethanol concentration. More specifically, the linear regression shown in provides a reasonable approximation for the entire data set, but did not accurately represent the trend in the test results for any one of the ethanol concentrations. Thus, while the delivery efficiency was influenced by the size of the atomized droplets (Phase I), it did not fully explain the results in .
FIG. 8 The delivery efficiency of HFA-134a solution MDIs plotted as a function of initial droplet MMD. These delivery efficiency results are from and the initial droplet MMD was estimated using (otherwise) identical configurations that used 1% OLA as the non-volatile component to provide residual particles of an optimal size for measurement.
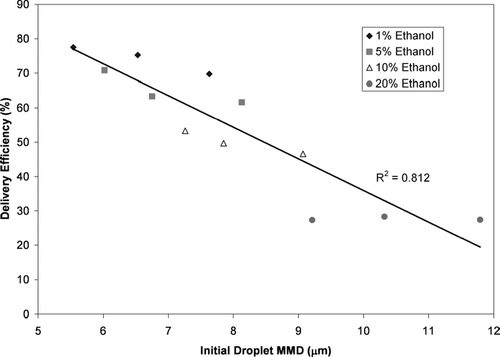
The Influence of Phase II (Evaporation) Factors on Delivery Efficiency
The results in the previous section indicate that the size of the atomized droplets was moderately correlated to the delivery efficiency (rˆ2 = 0.812). However, the initial droplet size does not explain all of the differences observed in the delivery efficiency. Thus, in this section, the influence of droplet evaporation on delivery efficiency was examined.
The time required for complete evaporation of a droplet depends on the fraction of ethanol in the droplet as well as the initial size of the droplet. The more ethanol that is present in the droplet, the longer the droplet lifetime is and the higher probability for deposition in the USP inlet. The intermediate droplet MMD, representing the diameter of the droplet consisting of drug and ethanol after all of the propellant has evaporated, was calculated for the MDI configurations evaluated in the previous section. These values were calculated from the initial droplet MMD shown in , the formulation composition, and Equations (Equation7) and (Equation8).
The delivery efficiency is plotted as a function of the intermediate droplet MMD in . The delivery efficiency was highly correlated to the intermediate droplet MMD. The intermediate droplet MMD was much more predictive of the delivery efficiency than the initial droplet MMD (Rˆ2 = 0.950 versus Rˆ2 = 0.812). Since the intermediate droplet MMD (which represents a characterization of the aerosol at a point in Phase II) correlated much better to delivery efficiency than does the initial droplet MMD (which is a characterization of the aerosol at Phase I), this implies that evaporation effects influence the delivery efficiency more than atomization effects.
FIG. 9 The delivery efficiency (from ) of HFA-134a MDIs plotted as a function of the intermediate droplet MMD.
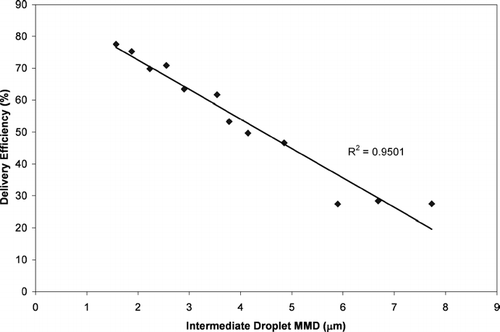
The results in indicate that the droplet evaporation process plays a significant role in the drug delivery from MDIs. A droplet lifetime was estimated for each of the configurations by theoretically calculating the time required for complete evaporation of the HFA-134a and ethanol in the droplets using Equations (Equation3)–(Equation6). As mentioned previously, the evaporation of the HFA-134a and ethanol was assumed to occur in two discrete stages. The regression equation in was used to estimate the time required for the HFA-134a to evaporate from the initial size to the intermediate size. Similarly the regression equation in was used to estimate the time required for evaporation of the ethanol as the droplet size decreased from the intermediate droplet MMD to the residual particle MMD. The total evaporation time was the sum of these two incremental times. Despite the simplifying assumptions the droplet lifetime was highly predictive of the MDI drug delivery ().
Drug Delivery from Alternative MDI Cosolvents
The results in the previous section suggest that MDI drug delivery efficiency was highly correlated to the time required for the evaporation of the volatile propellant and semi-volatile cosolvent and that evaporation kinetics strongly influenced MDI drug delivery efficiency—even more than the initial droplet diameter of the droplets. As cosolvent concentration increased, the time required for evaporation increased and the resulting drug delivery efficiency decreased. While reducing the concentration of ethanol is one approach to minimizing the droplet lifetime, an alternative approach is to use more volatile cosolvents. Traditionally, ethanol has been used as the cosolvent for almost all marketed MDI products. However, drug delivery could potentially be improved by using cosolvents that evaporate more rapidly than ethanol.
In order to examine this hypothesis, experimental solution MDIs were formulated that used various model cosolvents. All of the formulations used HFA-134a as the propellant and had cosolvent concentrations of 7% by weight. The cosolvents were selected primarily based on their volatility—no consideration was given to the toxicity or solvent capability of the cosolvent. The formulations all had non-volatile concentrations of 0.40% (0.36% OLA and 0.04% albuterol base—added as a marker for the chemical analysis). OLA and albuterol base were selected as the non-volatile components since the combination is soluble in HFA-134a regardless of the cosolvent selected. The criteria used to select the cosolvents were: (1) the cosolvent had to be miscible with HFA-134a at the level used; and (2) the cosolvents had to have a wide range of volatilities. For example, HFA-227 was considered a “cosolvent” in this case—even though it has virtually no solvent capability for most drugs and even though it is in reality a highly volatile propellant. It was selected as a model for the ideal (most volatile) cosolvent. The cosolvents examined were acetonitrile, butyl acetate, ethanol, ethyl acetate, HFA-227, methanol, and methyl acetate.
The delivery efficiency of the formulations with various cosolvents was characterized using the ACI and the residual particle MMAD was measured using the apparatus in . The results from these measurements are summarized in . The delivery efficiency and residual particle MMAD values represent averages of four tests. The cosolvent vapor pressure at 20°C and the wet bulb temperature drop calculated using Equation (Equation4) are also listed in to provide an indication of the volatility of the various cosolvents. The initial droplet MMD was calculated using Equations (Equation1) and (Equation2) and the droplet lifetime was calculated using the same approach described in the previous section.
TABLE 2 Results from theoretical and experimental assessment of MDIs using novel cosolvents
The initial droplet MMDs were very similar (8.73 to 9.28 μ m) for all of the formulations except for the acetonitrile formulation which had a slightly higher (10.55 μ m) initial droplet MMD. The initial droplet MMD and the delivery efficiency were unrelated for these formulations (Rˆ2 = 0.10 from linear regression). While the changes in delivery efficiency in was not due to changes in the size of the atomized droplets, the delivery efficiency appeared to be related to the volatility of the cosolvent used. For example, the formulation that used the most volatile cosolvent (HFA-227) was the most efficient formulation and the formulation with the least volatile cosolvent (butyl acetate) was least efficient. This is reasonable since HFA-227 droplets evaporate to residual particles more rapidly and are less likely to deposit on the actuator mouthpiece or USP inlet.
It is interesting that the ethanol and methanol formulations had almost identical delivery efficiencies despite the fact that methanol has a much higher vapor pressure than ethanol. This can be understood by examining the droplet lifetimes of the two formulations. Methanol has a wet bulb temperature drop of 35°C compared to a temperature drop of only 21.3°C for ethanol, leading to nearly identical droplet lifetimes despite the very different (room temperature) vapor pressures for the two cosolvents. Similarly, the ethyl acetate formulation was substantially more efficient than either of the methanol or acetonitrile formulations despite the fact that the vapor pressure of ethyl acetate is almost the same as acetonitrile and is lower than methanol. This can once again be explained by the more rapid evaporation of the ethyl acetate droplets compared to methanol or acetonitrile droplets. The droplet lifetime appeared to have a more significant influence on the delivery efficiency than the initial diameter of the atomized droplets or even the vapor pressure of the cosolvent used. Thus, for these formulations the drug delivery appeared to be determined more by evaporation kinetics than by the atomization conditions.
The delivery efficiency of the formulations with various cosolvents was plotted versus the droplet lifetime in . The data from the different cosolvent formulations was plotted along with the results from the testing of the formulations with different ethanol concentrations and different formulation temperatures (i.e., the data from ). Both sets of experiments showed a strikingly similar relationship between delivery efficiency and droplet lifetime. This is particularly noteworthy since test results from a very wide range of MDI configurations (various cosolvents, cosolvent concentrations, and testing temperatures) are summarized in . It should be noted that all of the experiments summarized in used the same valve (50 mcl SpraymiserTM) and actuators (QVAR® actuator with an orifice diameter of 0.3 mm). Therefore, the correlation should not be extrapolated to other valve sizes or actuator configurations. Nevertheless, indicates that evaporation kinetics play an extremely important role in determining MDI delivery efficiency.
CONCLUSIONS
Factors influencing the delivery efficiency from MDIs were examined theoretically and experimentally. The time required for evaporation of HFA-134a and ethanol droplets was theoretically calculated. HFA-134a droplets were calculated to evaporate approximately seven times faster than ethanol droplets of the same size, even though HFA-134a droplets cool to approximately 78°C below ambient temperature during evaporation. Delivery efficiency was experimentally shown to decrease with increasing ethanol concentration. This was determined not to be due to the changes in vapor pressure that occur as ethanol concentration increases. Delivery efficiency was shown to be somewhat related to the initial size of the atomized droplets, but much more strongly related to the time required for evaporation of the propellant and cosolvent. This is likely due to the fact that droplets which evaporate more slowly remain larger for a longer period and are more likely to deposit via turbulent deposition in the turbulent regions of the actuator mouthpiece and USP Inlet since turbuluent deposition preferably collects large particles. Tests with experimental MDIs using alternative cosolvents confirmed that MDI delivery efficiency is much more sensitive to the time required for evaporation of the droplets than on the formulation vapor pressure or the size of the atomized droplets. This indicates that factors affecting the evaporation of an MDI aerosol (Phase II effects) play a larger role in determining MDI delivery efficiency than do atomization effects (Phase I).
Acknowledgments
The authors would like to acknowledge Bill Harter for his assistance with the statistical analyses reported in this paper and Brian Gabrio for his consultation on the evaporation calculations.
Notes
a Ambient air temperature was assumed to be 20° Celsius.
b Properties listed as a function of temperature, T, in Kelvin.
a Initial droplet MMD calculated from residual MMAD, formulation composition, and Equations (Equation1) and (Equation2).
c Wet bulb temperature calculated using Equation (Equation4).
REFERENCES
- Brambilla , G. , Ganderton , D. , Garzia , R. , Lewis , D. , Meakin , B. and Ventura , P. 1999 . Modulation of Aerosol Clouds Produced by Pressurized Inhalation Aerosols . Int. J. Pharm. , 186 : 3 – 12 . [CSA]
- Cicerone , R. J. , Stolarski , R. S. and Walters , S. 1974 . Stratospheric Ozone Destruction by Man-Made Chlorofluoromethanes . Science , 185 : 1165 – 1167 . [CSA]
- Clark , A. 1991 . Metered Atomization for Respiratory Drug Delivery , Loughborough, , UK : Loughborough University . Ph.D. thesis
- Dalby , R. N. and Byron , P. R. 1988 . Comparison of Output Particle Size Distributions from Pressurized Aerosols Formulated as Solutions or Suspensions . Pharm. Res. , 5 ( 1 ) : 36 – 39 . [PUBMED] [INFOTRIEVE] [CROSSREF] [CSA]
- Davies , C. N. 1978 . “ Evaporation of Airborne Droplets ” . In Fundamentals of Aerosol Science , Edited by: Shaw , D. T. New York : Wiley .
- Dolovich , M. 1993 . Lung Dose, Distribution, and Clinical Response to Therapeutic Aerosols . Aerosol Sci. Technol. , 19 : 230 – 240 . [CSA]
- Dunbar , C. A. , Watkins , A. P. and Miller , J. F. 1997a . Theoretical Investigation of the Spray from a Pressurized Metered-Dose Inhaler . Atomization and Sprays , 7 : 417 – 436 . [CSA]
- Dunbar , C. A. , Watkins , A. P. and Miller , J. F. 1997b . An experimental Investigation of the Spray Issued from a pMDI Using Laser Diagnostic Techniques . J. Aerosol Med. , 10 : 351 – 368 . [PUBMED] [INFOTRIEVE] [CSA]
- Finlay , W. H. 2001 . The Mechanics of Inhaled Pharmaceutical Aerosols. , London : Academic Press .
- Gabrio , B. J. , Stein , S. W. and Velasquez , D. J. 1999 . A New Method to Evaluate Plume Characteristics of HFA and CFC Metered Dose Inhalers . Int. J. Pharm. , 186 : 3 – 12 . [PUBMED] [INFOTRIEVE] [CROSSREF] [CSA]
- Gupta , A. , Stein , S. W. and Myrdal , P. B. 2003 . Balancing Ethanol Cosolvent Concentration with Product Performance in 134a Based Pressurized Metered Dose Inhalers . J. Aerosol Medicine , 16 ( 2 ) : 167 – 174 . [CSA]
- Harnor , K. J. , Perkins , A. C. , Wastie , M. , Wilson , C. G. , Sims , E. E. , Feely , L. C. and Farr , S. J. 1993 . Effect of Vapour Pressure on the Deposition Pattern from Solution Phase Metered Dose Inhalers . Int. J. Pharm. , 95 : 111 – 116 . [CROSSREF] [CSA]
- Harrison , L. , Leach , C. , Machacek , J. , Vanden Burgt , J. and Vogel , J. 1997 . Beneficial Effects with Reduced Particle Size and CFC-free Extrafine Aerosol Steroid on Lung Deposition, Absorption, Efficacy and Safety . Am. J. Respir. Crit. Care Med. , 155 : A666 [CSA]
- Hickey , A. J. , Martonen , T. B. and Yang , Y. 1996 . Theoretical Relationship of Lung Deposition to the Fine Particle Fraction of Inhalation Aerosols . Pharmaceut. Acta Helvet. , 71 : 185 – 190 . [CROSSREF] [CSA]
- Hinds , W. C. 1999 . Aerosol Technology: Properties, Behavior, and Measurement of Airborne Particles. , New York : John Wiley and Sons .
- Hirst , P. H. , Pitcairn , G. R. , Weers , J. G. , Tarara , T. E. , Clark , A. R. , Dellamaary , L. A. , Hall , G. , Shorr , J. and Newman , S. P. 2002 . In Vivo Lung Deposition of Hollow Porous Particles from a Pressurized Metered Dose Inhaler . Pharmaceutical Research , 19 ( 3 ) : 258 – 264 . [PUBMED] [INFOTRIEVE] [CROSSREF] [CSA]
- Howarth , P. H. 2001 . Why Particle Size Should Affect Clinical Response to Inhaled Therapy . J. Aerosol Med. , 14 : S27 – S34 . [PUBMED] [INFOTRIEVE] [CROSSREF] [CSA]
- Kapitza , C. , Heise , T. , McGovern , M. , Cefali , E. , Buchwald , A. , Heinemann , L. and Hompesch , M. Time-Action Profile of a New Pulmonary Insulin Applied with a Metered Dose Inhaler . American Diabetes Association, 63rd Scientific Session . June 13–17 2003 , New Orleans, La.
- Leach , C. L. , Davidson , P. J. and Boudreau , R. J. 1998 . Improved Airway Targeting with the CFC-Free HFA-Beclomethasone Metered-Dose Inhaler Compared with CFC-Beclomethasone . Eur. Respir. J. , 12 : 1346 – 1353 . [PUBMED] [INFOTRIEVE] [CROSSREF] [CSA]
- Leach , C. 1999 . Effect of Formulation Parameters on Hydrofluoroalkane-Beclomethasone Dipropionate Drug Deposition in Humans . J. Allergy Clin. Immunol. , 104 : S250 – S252 . [PUBMED] [INFOTRIEVE] [CROSSREF] [CSA]
- Leach , C. L. , Hameister , W. H. and Tomai , M. A. 2000 . “ Oligolactic Acid (OLA) Biomatracies for Sustained Release of Asthma Therapeutics ” . In Respiratory Drug Delivery VII , Edited by: Byron , P. R. , Dalby , R. N. , Farr , S. J. and Peart , J. 75 – 81 . Raleigh, NC : Serentec Press .
- Leach , C. L. , Davidson , P. J. , Hasselquist , B. E. and Boudreau , R. J. 2002 . Lung Deposition of Hydrofluoroalkane-134a Beclomethasone is Greater than that of Chlorofluorocarbon Fluticasone and Chlorofluorocarbon Beclomethasone: a Cross-over Study in Healthy Volunteers . Chest. , 122 : 510 – 516 . [PUBMED] [INFOTRIEVE] [CROSSREF] [CSA]
- Lewis , D. , Ganderton , D. , Meakin , B. and Brambilla , G. 2004 . “ Theory and Practice with Solution Systems ” . In Respirator Drug Delivery IX , Edited by: Dalby , R. R. , Byron , P. R. , Peart , J. , Suman , J. and Farr , S. J. pp. 109 – 115 . River Grove, IL : Davis Healthcare Int'l. Publ. .
- Lippman , M. and Albert , R. E. 1969 . The Effect of Particle Size on the Regional Deposition of Inhaled Aerosols in the Human Respiratory Tract . Am. Ind. Hyg. Ass. J. , 30 : 257 – 275 . [CSA]
- Liu , B. Y. H. and Agarwal , J. K. 1974 . Experimental Observation of Aerosol Deposition in Turbulent Flow . J. Aerosol Sci. , 5 : 145 – 155 . [CROSSREF] [CSA]
- Marple , V. A. and Willeke , K. 1976 . Impactor Design . Atmos. Environ. , 10 : 891 – 896 . [CROSSREF] [CSA]
- McKenzie , L. and Oliver , M. J. 2000 . Evaluation of the Particle Formation Process After Actuation of Solution MDIs . J. Aerosol Med. , 13 : 59 [CSA]
- Mitchell , J. P. and Nagel , M. W. 2003 . Cascade Impactors for the Size Characterization of Aerosols from Medical Inhalers: Their Uses and Limitations . J. Aerosol Med. , 16 : 341 – 377 . [PUBMED] [INFOTRIEVE] [CROSSREF] [CSA]
- Molina , M. J. and Rowland , F. S. 1974 . Stratospheric Sink for Chlorfluoromethanes: Chlorine Atom-Catalyzed Destruction of Ozone . Nature , 249 : 810 – 812 . [CROSSREF] [CSA]
- Morrow , P. H. 1966 . Deposition and Retention Models for Internasal Dosimetry of the Human Respiratory Tract . Health Phys. , 12 : 173 – 207 . [PUBMED] [INFOTRIEVE] [CSA]
- Naini , V. , Chaudhry , S. , Berry , J. , Sharpe , S. , Hart , J. and Sequeira , J. 2004 . Entry Port Selection for Detecting Particle Size Differences in Metered Dose Inhaler Formulations Using Cascade Impaction . Drug Deliv. Industrial Pharm. , 30 ( 1 ) : 75 – 82 . [CROSSREF] [CSA]
- Newman , S. P. , Moren , F. , Pavia , D. , Corrado , O. and Clarke , S. W. 1982 . The Effects of Changes in Metered Volume and Propellant Vapor Pressure on the Deposition of Pressurized Inhalation Aerosols . Int. J. Pharm. , 11 : 337 – 344 . [CROSSREF] [CSA]
- Rance , R. W. 1972 . Particle Size Distribution Measurement of Hair Spray Using an Image Splitting Particle Size Analyzer . J. Cos. Chem. , 23 : 197 – 208 . [CSA]
- Rance , R. W. 1974 . Studies of the Factors Controlling the Action of Hairsprays. III: The Influence of Particle Velocity and Diameter on the Capture of Particles by Arrays of Hair Fibres . J. Cos. Chem. , 25 : 545 – 561 . [CSA]
- Ross , D. L. and Gabrio , B. J. 1999 . Advances in Metered Dose Inhaler Technology with the Development of a Chlorofluorocarbon-Free Drug Delivery System . J. Aerosol Med. , 12 : 151 – 160 . [PUBMED] [INFOTRIEVE] [CSA]
- Smyth , H. D. C. , Mejia-Millan , E. A. and Hickey , A. J. 2002 . “ The Effect of Ethanol on Solvancy, Vapor Pressure, and Emitted Droplet Size of Solution Metered Dose Inhalers Containing HFA-134a ” . In Respiratory Drug Delivery VII , Edited by: Dalby , R. R. , Byron , P. R. , Peart , J. and Farr , S. J. pp. 735 – 738 . Raleigh, NC : Davis Horwood Int. Pub. .
- Stefely , J. S. , Duan , D. C. , Myrdal , P. B. , Ross , D. L. , Schultz , D. W. and Leach , C. L. 2000 . “ Design and Utility of a Novel Class of Biocompatible Excipients for HFA-based MDIs ” . In Respiratory Drug Delivery VII , Edited by: Byron , P. R. , Dalby , R. N. , Farr , S. J. and Peart , J. pp. 83 – 90 . Raleigh, NC : Serentec Press .
- Stefely , J. S. 2002 . Novel Excipients for Inhalation Drug Delivery: Expanding the Capabilities of the MDI . Drug Deliv. Tech. , 2 : 62, 64 – 69 . [CSA]
- Stein , S. W. and Gabrio , B. J. 2000 . “ Understanding Throat Deposition During Cascade Impactor Testing ” . In Respiratory Drug Delivery VII Edited by: Byron , P. R. , Dalby , R. N. , Farr , S. J. and Peart , J. Vol 2 , pp. 287 – 290 .
- Stein , S. W. and Olson , B. A. 1997 . Variability in Size Distribution Measurements Obtained Using Multiple Andersen Mark II Cascade Impactors . Pharmaceutical Research , 14 : 1718 – 1725 . [PUBMED] [INFOTRIEVE] [CROSSREF] [CSA]
- Stein , S. W. and Stefely , J. S. 2003 . Reinventing Metered Dose Inhalers: From Poorly Efficient CFC MDIs to Highly Efficient HFA MDIs . Drug Del. Technol. , 3 ( 1 ) : 46 – 51 . [CSA]
- Stein , S. and Myrdal , P. 2004 . A Theoretical and Experimental Analysis of Formulation and Device Parameters Affecting Solution MDI Size Distributions . J. Pharm. Sci. , 93 : 2158 – 2175 . [PUBMED] [INFOTRIEVE] [CROSSREF] [CSA]
- Stein , S. , Gabrio , B. and Myrdal , P. 2005 . “ The Influence of Vapor Pressure on the Size of Atomized MDI Droplets ” . In Respiratory Drug Delivery Europe , Edited by: Dalby , R. N. , Byron , P. R. , Peart , J. and Suman , J. D. 151 – 155 . River Grove, IL : Davis Healthcare Intl. Publishing .
- U.S. Pharmacopeia . 2005 . Physical Tests and Determinations < 601 > Aerosols, Nasal Sprays, Metered Dose Inhalers, and Dry Powder Inhalers , pp. 2359 – 2377 . Rockville, MD : The United States Pharmacopial Convention, Inc. . USP 28
- U.S. Pharmacopeia . 2003 . The Official Compendia of Standards, USP 26/NF 21 , 56 – 57 . Rockville, MD : United States Pharmacopeial Convention, Inc . Official Monographs
- Versteeg , H. K. , Hargrave , G. , Harrington , L. , Shrubb , I. and Hodson , D . 2000 . “ The Use of Computational Fluid Dynamics (CFD) to Predict pMDI Air Flows and Aerosol Plume Formation ” . In Respiratory Drug Delivery VII , Edited by: Byron , P. R. , Dalby , R. N. , Farr , S. J. and Peart , J. Vol. 1 , pp. 257 – 264 . Raleigh, NC : Serentec Press .
- Wilson , D. M. , Peart , J. , Martin , B. R. , Bridgen , D. T. , Byron , P. R. and Lichtman , A. H. 2002 . Physicochemical and Pharmacological Characterization of a Δ 9-THC Aerosol Generated in a Metered Dose Inhaler . Drug and Alcohol Dependence , 67 : 259 – 267 . [PUBMED] [INFOTRIEVE] [CROSSREF] [CSA]
- Wofsy , S. C. , McElroy , M. B. and Sze , N. D. 1975 . Freon Consumption: Implications for Atmospheric Ozone . Science , 187 : 535 – 537 . [CSA]