Abstract
The collection characteristics of a small deposit area low pressure impactor (SDI) were studied in order to employ the impactor for size distribution measurements of carbonaceous matter. In this work, the SDI was calibrated for soft and porous quartz substrate material in a series of laboratory experiments. The collection efficiency curves were measured by using monodisperse dioctyl sebacate particles and by applying two different detection methods. One method was based on the detection of current carried by charged test particles, and the other measured number concentrations of particles in bipolar charge equilibrium by two condensation particle counters. Concerning the particle size corresponding to a 50% collection efficiency (D 50 ), significant shifts toward smaller particle sizes were found for the quartz fiber substrates compared with the flat plates. Also the shapes of the collection efficiency curves differed considerably: quartz substrate gave less steep curves than plain impaction plates. The new calibration was applied to field data from urban and rural sites. Compared with the original calibration of the SDI, the new calibration changed the measured size distributions of organic and elemental carbon. In addition, a reasonable size-segregated mass closure was achieved by combining data from thermal-optical analysis and ion-chromatography.
INTRODUCTION
Various types of impactors are widely used for the collection of size-segregated particulate matter. In the atmosphere, a multi-stage impactor can typically collect samples over the range 30 nm–10 μ m of particle aerodynamic diameter. Impactor stages operating in the range of 0.5–10 μ m are easy to design and manufacture, since the diameters of impactor orifices are large and easy to drill. In order to extend the size range below approximately 0.5 μ m, a special design is needed. Two basic approaches have been used for this purpose. In the design of CitationHering et al. (1978), there is a critical non-collecting orifice reducing the pressure of the lowest stages enabling collection of sub-0.5 μ m particles. In the design of the Berner low-pressure impactor (BLPI; Berner and Lürzer 1980), every stage is collecting but the pressure is reduced gradually using different combinations of the number of the nozzles and their diameters for each stage. A low pressure and high jet velocities (close to sonic for lowest stages) allow the collection of particles down to 30 nm. In the micro-orifice uniform deposit impactor (MOUDI), designed by CitationMarple et al. (1991), the extension of the particle size range is made using micro-orifices. Using a stage pressure of 55 kPa for the lowest stage and 50 μ m orifices, particles as small as 0.056 μ m can be collected. In a more recent design called nano-MOUDI, the size range is extended down to 10 nm using a combination of micro-orifices and low pressure (CitationMarple and Olson 1999).
Provided that there is a single nozzle or that nozzles are not very close to each other, and that the collection surface is a planar and smooth foil or film perpendicular to the nozzles, the theory predicts the collection properties of an impactor stage quite well (CitationRader and Marple 1985; CitationHillamo and Kauppinen 1991). For multiple nozzles and non-ideal collection surfaces, such as a porous surface, the situation is more complicated (CitationHuang et al. 2001; CitationMarjamäki and Keskinen 2004). The use of a non-ideal collection surface may be necessary because of required high mass loads (CitationKavouras and Koutrakis 2001; CitationSillanpää et al. 2003) or because of special analytical purposes (CitationViidanoja et al. 2002; CitationCarvalho et al. 2003; CitationHasegava et al. 2004; CitationJaffrezo et al. 2005; CitationYttri et al. 2005). Porous substrates have also been found to reduce particle bouncing from impactor stages (CitationFujitani et al. 2006).
A special analytical method that needs the use of a porous material in particle collection is the thermal-optical analysis (TOA; Chow et al. 1993; CitationBirch and Cary 1996). In that method the sampling media have to resist temperatures of up to 700–900°C, as a result of which quartz fiber filters are commonly used as impactor substrates for subsequent thermal analyses. The major disadvantage of using quartz filters is that the filter substrate is thick and porous. It can therefore be expected that the air jets carrying particles penetrate the filter surface and particle collection characteristics will be altered compared with dense films or foils as a collection medium.
In this study, the collection characteristics of the small deposit area low pressure impactor (SDI; Maenhaut et al. 1996) were studied in order to employ the impactor for size distribution measurements of carbonaceous matter. Because of the small deposit area of the SDI, the whole impactor substrate of the SDI can be analyzed using the thermal-optical transmission method. The SDI was calibrated for quartz fiber substrates and the new collection efficiency curves were used to extract the size distributions of carbonaceous matter at two different environments: rural and urban. By combining OC and EC size distributions with the size distributions measured for the mass and inorganic ions using another SDI, a size-segregated mass closure was obtained.
IMPACTOR DESIGN, CALIBRATION, AND DATA REDUCTION
Most often the practical impactor design is based on the theory of CitationMarple and Liu (1974). For a certain nozzle design there is a dimensionless parameter, called the Stokes number (Stk), which is defined as the ratio of the particle stopping distance (S = τ V) to the nozzle radius (W/2) at the average jet velocity V0 (CitationHinds 1999):
Although the impactor theory predicts the collection efficiency of impactor stages quite well also for designs different than an ideal single round (or slit) nozzle, most impactors are calibrated experimentally. Calibration data can be used in two different ways. In a simple approach, only one particle size (diameter) corresponding to the 50% collection efficiency of a stage (often called cut-off diameter D50) is used to represent the stage collection. If the collection efficiency curve is steep and the impactor has 4–5 stages per decade, this approach may give reasonable size distributions for different mass concentration modes of atmospheric aerosols. However, for more detailed analysis of impactor data or less steep collection efficiency curves, data inversion procedures (CitationWolfenbarger and Seinfeld 1990; CitationWinklmayer et al. 1990) are useful. For data inversion one usually needs the whole collection efficiency curve as input data. In most cases collection efficiency curves are obtained experimentally.
EXPERIMENTAL
Small Deposit Area Impactor
The details of the design of the SDI have been presented in CitationMaenhaut et al. (1996). Briefly, the SDI has 12 collecting stages with the nominal aerodynamic cut-off diameters equal to 0.045, 0.086, 0.153, 0.231, 0.343, 0.591, 0.796, 1.06, 1.66, 2.68, 4.08, and 8.50 μ m. The basic constraint in the design has been to limit the deposits within circular area with a diameter of 7 mm. This feature is useful for several reasons. First, it often optimizes the detection limit for the combination of sample collection and subsequent analysis. Second, the handling of small substrates is easy, which minimizes the potential contamination of the sample. Third, and most importantly for the application of this study, the whole impactor substrate of the SDI can be analyzed using the thermal-optical transmission method. summarizes the design of the SDI.
TABLE 1 The design of the SDI
The collection efficiency measurements were made both for the plain impaction plates (stainless steel) and quartz fiber substrates. Concerning the sampling characteristics, the plain plate can be assumed to behave similar to a plate covered with a film substrate. Quartz fiber substrates (diameter 21 mm) were punched from 47-mm quartz fiber filters (Q-MA, Whatman International Ltd, Maidstone, England). Also substrates cut from the Gelman Pallflex quartz fiber filters (type 2500QAT-UP) were tested, but those results were congruent with those using Whatman filters and are therefore not presented here. Quartz material is thicker compared with aluminum foil or polycarbonate film used generally in the SDI. Therefore the support disks of the SDI were thinned for the quartz substrates in order to keep the jet-to-plate distance the same as in the original impactor stage. The adjustment of the jet-to-plate distance was done before the calibration using a depth gauge with a 10-μ m resolution. Only the eight lowest impactor stages (stages 1–8) were calibrated, since the main interest of this study was in submicron carbonaceous matter, and since the used particle generation system was limited to produce particles smaller than 1 μ m.
Collection Efficiency Curve Measurements
Particle Generation
Calibrations were performed using monodisperse dioctyl sebacate particles (DOS, density 0.914 g/cm3). The primary aerosol consisting of submicrometer particles (0.01–1 μ m) was generated with a Constant Output Atomizer (TSI Model 3068, St. Paul, Minnesota, USA) from 0.002% to 2% solutions of DOS in 2-propanol. The aerosol obtained from the atomizer was transported into an evaporation-condensation aerosol generator in order to get a narrow particle number size distribution (CitationLiu and Lee 1975). After this procedure a desired monodisperse particle size fraction was selected using a Differential Mobility Analyzer (DMA, TSI Model 3080, St. Paul, Minnesota, USA). The narrow size distribution minimized the number of multiple-charged particles and kept the aerosol highly monodisperse.
Electric Detection
The collection efficiency curves were measured in two ways, using either electric detection or two condensation particle counters (CPCs). The electric measurement method has been described in detail by CitationHillamo and Kauppinen (1991) and CitationMaenhaut et al. (1996). Briefly, charged monodisperse test particles from the DMA were fed into the impactor stage and the current carried by these particles was measured simultaneously from the impaction plate and back-up filter using electrometers (Keithley 617 Programmable Electrometer, Cleveland, OH, USA). The quartz filter was attached to the metal impaction plate with a conductive tape. The collection efficiency was calculated as the ratio of impacted portion to the sum of impacted and penetrated portions. Since the impaction plate was electrically isolated from the rest of the impactor body by a plate made of polytetrafluoroethylene (PTFE), this method measured the real impaction efficiency and neglected the collection due to inter-stage losses.
The method enables a real-time measurement of the collection efficiency for a single particle diameter. The method is therefore quick, and if monodisperse particles are selected with the DMA, the particle size selection and data acquisition of the electrometers can be made using a computer. The disadvantage is that particles used in the measurement have a unipolar charge. This may result in undesirable collection of particles by mechanisms other than impaction, such as deposition due to the electric field caused by an image charge (CitationHillamo and Kauppinen 1991; CitationMarjamäki and Keskinen 2004).
Condensation Particle Counter Detection
In the second calibration system the detection of the particles was made using two CPCs (TSI Model 3010), one before and another after an impactor stage. For most of the stages the measurement was done well below the atmospheric pressure, but, as shown by CitationHermann and Wiedensohler (2001), the CPC can operate properly also under reduced pressures. In this work particle concentrations were measured down to 20 kPa without any leakages due to the low pressure. The only modification made for the CPC was the plugging of the butanol intake tube during the measurement. Compared with the electrical detection, the test aerosols were generated in a similar way, but were neutralized by using a Kr-85 radioactive source before the CPC detection. The concentrations were measured from the CPC directly or on the basis of detected pulses. The latter was made using a computer. Before the calibration, a measurement was made without an impaction plate in order to determine the factor used to normalize the concentration measurements before and after the impactor stage. The factor takes into account differences in the flow rate and absolute pressure upstream and downstream of the stage as well as losses in the CPC sampling lines and inside the impactor. In addition to the base line measured by filtrating the aerosols off, also the systematic difference between the two CPCs was measured on a daily basis by measuring parallel with two instruments. The difference of the CPCs was 6% at maximum and it was taken into account in calculating the collection efficiency. The measurement set-up is shown in .
The collection efficiency was calculated from the concentration data by assuming that the difference between the upstream and downstream concentrations (after applying the corrections explained before) was due to particle impaction. As a result, the collection efficiency was equal to the ratio of this difference to the upstream concentration. The measurement was made for both plain impaction plate and quartz fiber substrate. The former data are not shown because they were used only for checking out that the impactor configuration equaled that used in the electrical detection.
Field Measurements
Sites
Field measurements were carried out at an urban and rural site in Finland. The urban site was located in Helsinki, 65 meters from a busy road. Sampling at this site was carried out between January and March 2004. The sampling duration was about 17 hours. The rural site was located in a boreal forest in Hyytiälä (SMEAR II), representing a background area of southern Finland (CitationKulmala et al. 2001). In Hyytiälä the samples were collected during May 2004. Since particle mass concentrations are typically very low in Hyytiälä, the sampling duration ranged from two days to five days.
Sampling System and Analytical Methods
Instruments and analytical methods used for the field measurements have been described in detail by CitationSaarikoski et al. (2005). Briefly, the sampling system consisted of three annular denuders (URG-2000, 30 × 242 mm, Chapel Hill, NC) followed by the SDI and virtual impactor (VI; Loo and Cork 1988) with a cut-off diameter of 1.3 μ m. The denuders were coated with XAD-4 material according to CitationGundel et al. (1995) and no single denuder was used longer than 24 hours. In the VI two quartz filters (Whatman Q-MA, 47 mm) were used back-to-back and correspondingly, the substrates used in the SDI were cut from the same filter material. Prior to the sampling, the quartz filters and substrates were cleaned at 550°C for six hours. The VI samples collected by using quartz filters (VI-Q), as well as the SDI quartz filter substrates (SDI-Q), were analyzed for organic and elemental carbon (OC and EC) with a thermal-optical transmittance method by using a carbon analyzer developed by Sunset Laboratory Inc., Oregon. The thermal method had four temperature steps in a helium-phase (310, 480, 615, and 800°C) and four steps in a helium-oxygen phase (675, 750, 825, and 920°C). For the SDI, the charring of OC was corrected by the method of CitationViidanoja et al. (2002) with the help of parallel VI samples.
In Hyytiälä, a second set of SDI and VI was used for the a closure study. In this system the SDI was loaded with greased aluminum substrates (SDI-Al), whereas the VI had PTFE filters (VI-PTFE; Millipore Fluoropore, 3.0 μ m, 47 mm). No denuders were used prior to the SDI-Al and VI-PTFE. The SDI-Al and VI-PTFE samples were weighed before and after the sampling using a microbalance (MT5, Mettler-Toledo Inc. Hightstown, NJ) and analyzed for ions (methanesulfonate (MSA), chloride, nitrate, sulfate, oxalate, sodium, ammonium (NH4), potassium, magnesium, and calcium) by ion chromatography (CitationTeinilä et al. 2000). The sample types and performed analyses for the samples are listed in .
TABLE 2 List of sample types used in text. Same sampling set-up was used in the field measurements conducted in Helsinki (urban) and in Hyytiälä (rural)
RESULTS AND DISCUSSION
Calibration of SDI for Quartz
Collection Efficiency Curves
The collection efficiency curves measured by using the electric and CPC method are shown in . Of two detection methods, the electric one is technically easier. It can be made online for a single atomizer primary solution, and one calibration point is an average of several measurements during a short sampling time. As a result, the collection efficiency curves are almost continuous with only a minor statistical error. The drawback, as mentioned before, is that the particles are charged, which increases the collection efficiency of particles due to electric field caused by the image charge (CitationVirtanen et al. 2001). In order to exclude the potential image charge effect on the collection efficiency, stages 2–8 were calibrated with the CPCs using particles with a bipolar equilibrium charge distribution. Due to the low pressure, stage 1 was not calibrated with the CPC method. In general the agreement between the two calibration methods was good, even though the CPC method suffered from poor statistics especially when the number concentration was low (the ends of the curves, and stages 7 and 8). The best agreement between the two methods was achieved for stages 4, 5, and 6. As anticipated, the lowest stages (1–3) had slightly higher collection efficiencies with the electric method than with the CPCs, which was most likely caused by the image charge (). For stages 4–8 the image charge did not modify the collection characteristics very much because inertial forces were the dominating deposition mechanism for particles of these sizes.
FIG. 2 Collection efficiency curves for plain impaction plate (a) and for quartz substrates (b–c). Quartz substrates were calibrated using two detection methods, the electric detection (b) and the CPC detection (c).
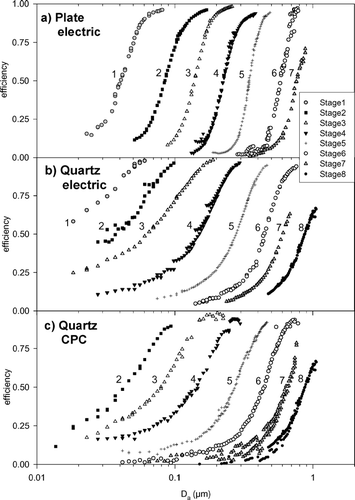
The cut-off diameters calculated from the collection efficiency curves are summarized in . For the quartz substrate the cut-off sizes measured with the two methods were not far from each other, but due to the increased collection caused by the image charge, the D50 values for stages 2 and 3 were slightly lower with the electric method than with the CPCs. In contrast, the difference between the quartz substrate and plate was significant ().
FIG. 3 D50 values measured for the plate plotted against those measured for quartz substrate (a) and the adiabatic velocity of individual stages against the square root of Stk50 (b). Adiabatic velocities are given in and Stk50 ½ values in . Note that stage 1 was calibrated only using the electric method.
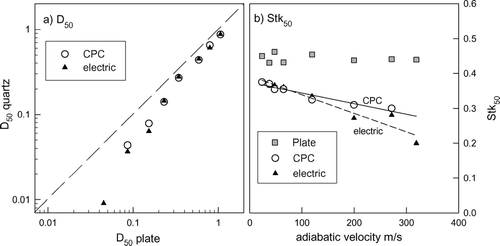
TABLE 3 Parameters characterizing the collection efficiency curves. D50 values, (Stk)½, and slopes were calculated by fitting a sigmoidal curve to the calibration data points shown in . The uncertainty of the D50 was estimated on the basis of repeated tests and the uncertainty of instruments
In addition to the D50 values, also the shape of the collection efficiency curve changed when the flat substrate was replaced by porous quartz substrate. The collection efficiency curves for the quartz were not as steep as the corresponding curves for the plate (). Again, the difference between the curves was its largest for stages 2–4 (stage 1 not determined for quartz), as indicated in by the ratio of particle aerodynamic diameters corresponding to 84% and 50% collection efficiencies. For stages 5 and 6 the steepness of the quartz curve was closer to the curve for the plate. The increased collection efficiency of the quartz can be explained by filtration. The jet penetrates the porous substrate, and particles will be collected in addition to impaction by interception, and for the smallest particle sizes also by diffusion, inside the quartz material. From it can be seen that the difference between the plate and quartz increased with decreasing particle size. In addition to the smaller particle size, this feature is obviously related to the higher jet velocity (see ). For the porous sinter impaction plate used in the Electrical low-pressure impactor (ELPI), most of the change in the collection efficiency was found to be caused by the surface roughness (CitationMarjamäki and Keskinen 2004). The effect of the surface roughness for the quartz substrate is not easy to assess because of the substrate deformation under the jet for the highest velocities (for the SDI, stages 1–5).
shows the square root of the particle Stokes number with 50% collection efficiency (Stk50 ½) as a function of the adiabatic jet velocity. As presented also in , Stk50 ½ was quite constant for the plain plate but decreased clearly for quartz fiber substrate when the jet velocity increased (stage number decreased). The systematic decrease of Stk50 ½ with increasing velocity is obviously due to the deeper penetration of particles at higher jet velocities and subsequent filtration by the quartz fiber substrate material. This effect strengthens when going to the lower impactor stages because the particle sizes are smaller and subsequently diffusional deposition higher.
Since only stages 2–8 were calibrated for the quartz using CPCs and stages 1–8 using the electric method, the remaining D50values were calculated by fitting linear curves for the data of the and by calculating the corresponding Stk50 ½ values for the known adiabatic velocities of individual stages (). For the CPC method the D50 value equal to 0.015 μ m was obtained for stage 1. For stages 9, 10, 11, and 12, the cut-off sizes were equal to 1.3, 2.0, 3.3, and 6.7 μ m, respectively, being the same for the CPC and electric methods.
Effect of Mass Loading
For stage 4, collection efficiency curve was measured for the particle loaded quartz substrate as well. Stage 4 was chosen for this test because it collects over size range where the mass of atmospheric aerosol particles is concentrated and because its jet velocity is high enough to penetrate the quartz substrate. The test procedure involved three steps. First, the collection efficiency of stage 4 was measured using a clean quartz substrate. The same substrate was let to collect atmospheric particles over the night in the lab. The quartz substrate (stage 4) was roughly estimated to have collected 20–30 μ g of particulate mass from the laboratory air. Second, the efficiency curve was measured again for the loaded substrate. The collection efficiency curves for clean quartz, loaded quartz and plain impaction plate are shown in . The loading of the substrate did not significantly alter the D50 value but the shape of the collection efficiency curve changed slightly. The lower part of the curve descended, which means that the collection of small particles was more efficient for the unloaded (clean) quartz substrate than for the loaded substrate. This finding is in line with the suggestion that impaction is not solely responsible for the collection when the quartz substrate is used. As the loading increases, the penetration of the jet is smaller, and the collection efficiency by filtering decreases, which decreases the overall collection efficiency. In contrast to quartz, the loading has not been found to have effect on porous sinter plates (CitationMarjamäki and Keskinen, 2004) or on porous foam substrates (CitationHuang et al. 2005).
Field Measurements
Effect of Cut-Off Diameters on the Size Distributions
Ions. In order to test the validity of the new cut-off sizes for quartz, two SDIs used in parallel in the field (SDI-Q and SDI-Al) were both analyzed for ions. Sampling was carried out in Hyytiälä and the sampling duration was five days (May 11–16, 2004). Contrary to other samplings done in Helsinki and Hyytiälä, no denuders were used prior to the SDI-Q. Of all the ions determined, methanesulfonate and ammonium were the only compounds that had sufficient concentrations in the SDIs but no significant blanks in the quartz substrates. Because of that, MSA and ammonium were used for comparison of size distributions.
By summing up all the stages in the SDI, the SDI-Q had more MSA and ammonium than the SDI-Al with the SDI-Al to SDI-Q ratio of 0.79 for both MSA and ammonium. The reason for this difference could be the better collection efficiency of quartz substrates (less particle bouncing; CitationFujitani et al. 2006). The size distributions of MSA and ammonium calculated using the original and the new D50 values are shown in . In case of the SDI-Al, the D50 values re-calibrated in this study (; plate) were used, however, the values for stages 9–12 were obtained from the earlier calibration of the SDI (CitationMaenhaut et al. 1996).
FIG. 5 Size distributions of MSA (a) and ammonium (b) analyzed from aluminum (SDI-Al) and quartz substrates (SDI-Q). Original D50 values and new D50 values. Measurements were performed in Hyytiälä May 11–16, 2004.
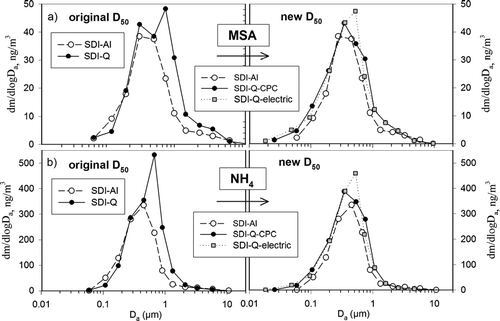
By using the original D50 values for both the SDI-Q and SDI-Al, the size distributions were rather different (, left side). For MSA the SDI-Q gave two modes in the accumulation size range peaking at stages 4 and 6, whereas the SDI-Al gave only one mode with the maximum at stage 4. The size distribution of ammonium from the SDI-Q had a sharp maximum at stage 6, but no such peak could be seen in the distribution obtained from the SDI-Al. For both MSA and ammonium in the size range of 0.1–0.2 μ m (stages 2 and 3 for ammonium and stage 2 for MSA), the concentrations were lower for the SDI-Q than for the SDI-Al.
On the right side of the new D50 values were used for the SDI-Q while the SDI-Al was the same as in the left side. Results derived from the electric and CPC method are shown separately. It can be seen that with the new D50 values for the SDI-Q, the size distributions of the SDI-Q and the SDI-Al were much more similar. For MSA two accumulation modes displayed by the SDI-Q were replaced by one mode when the new values were used. In case of ammonium the accumulation mode of the SDI-Q shifted to smaller particle sizes and the sharp maximum at stage 6 disappeared.
The size distributions calculated using the D50 values obtained by the electric method differed slightly from those obtained by the CPCs. With the D50 values from the electric method the maximum of the mode (MSA and ammonium) was at the larger particle size than that calculated with the D50 values from the CPC method. Also, the mode was narrower with the electric D50 values, although both the SDI-Qs gave broader mode than the SDI-Al. For particles with the diameter approximately in the range 0.05–0.06 μ m (stage 1 for the SDI-Al and stage 2 for the SDI-Q), the concentrations of MSA and ammonium were a bit larger for the SDI-Q (CPC and electric) than for the SDI-Al. The differences between the electric and CPC distributions resulted from the uncertainties in the calibrations, especially from the D50 values measured for stage 7 ().
OC and EC. The new D50 values for the SDI-Q were applied for OC and EC measurements carried out at urban and rural sites (Helsinki and Hyytiälä, respectively). Concurrently with the SDI-Q a virtual impactor (VI-Q) was used. Denuders were used prior to the SDI and VI in order to remove gas-phase organic compounds which may adsorb on quartz substrates (CitationViidanoja et al. 2002). Compared with the VI, the SDI had lower OC and EC concentrations. On average, the ratio of SDI-Q (sum of stages 1–12) to VI-Q (sum of fine and coarse) was 0.78 and 0.81 for OC and 0.66 and 0.78 for EC in Helsinki and Hyytiälä, respectively. The systematic difference between the SDI-Q and VI-Q might be due to the bouncing and inter-stage losses of particles in the SDI, as well as due to analytical uncertainties caused by the small OC and EC concentrations in the size-segregated samples of the SDI.
The mass size distributions for OC and EC calculated with the original and new D50 values, and derived from the CPC and electric method, are shown in . For the stages that were not calibrated in this study, the D50 values were calculated as described in the previous sections. The size distributions of OC and EC changed significantly when using the new D50 values. First, the concentrations of small particles (0.03–0.2 μ m) increased, which was observed for both OC and EC measured in Hyytiälä and Helsinki. Second, in most of the cases two sharp maximums in the accumulation size range (0.1–1 μ m) were replaced by a single maximum that was located between the “old” ones (). However, in some cases two peaks in the accumulation size range remained, especially when the D50 values from the CPC method were used ( and ). If the size distribution calculated with the original D50 values had only one accumulation mode, the maximum usually shifted to smaller particle sizes (). By comparing the CPC and electric methods, the electric method gave an additional mode in the particle size of 0.05 μ m. This mode was found not only for OC in Helsinki and Hyytiälä but also for EC in Helsinki.
FIG. 6 Mass size distributions of OC (a, c) and EC (b, d) calculated using new and original D50 values. Measurements were done in Helsinki (a, b) January 19–20, 2004, and in Hyytiälä (c, d) May 26–28, 2004.
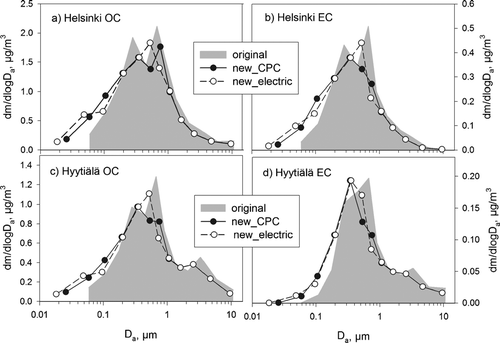
Mass closure. Concurrently with the SDI and VI dedicated to measure size distributions and concentrations for carbonaceous particles (SDI-Q and VI-Q), another set of the SDI and VI (SDI-Al and VI-PTFE) was used for mass and ions in Hyytiälä (). In general, the size distribution of OC was different (both CPC and electric methods) from that of mass and ions. Comparison of the size distributions of OC and sulfate (the latter was the most prominent inorganic ion in Hyytiälä) showed that OC had a broader accumulation mode than sulfate (not shown) resulting from the larger amount of OC at sizes < 0.5 μ m. Aerosol Mass Spectrometer measurements have shown that this fraction of OC can be attributed to hydrocarbon-like organic aerosol in urban area (CitationZhang et al. 2005) but in remote sites most of OC has found to be oxygenated (CitationZhang et al. 2007).
Although the D50 values for the aluminum and quartz were different, the mass closure was constructed by subtracting analyzed inorganic ions from the gravimetric mass and by comparing the remaining mass with the measured OC and EC concentrations calculated using the D50 values from the electric method (). The white area between OC and ions (unanalyzed) consists mainly of oxygen and hydrogen atoms in organic compounds not analyzed in this study (the difference between OC and particulate organic matter, POM). The accumulation mode of total carbon (TC; OC+EC) fitted well to the area that remained when the inorganic ions were subtracted from mass. However, the right side of the accumulation mode was steeper for unanalyzed mass than for TC. Also, in the particle size range of 0.02–0.1 μ m there was a mode for OC that was not found for mass. That mode might be due to gaseous OC evaporated from particles collected in the upper stages of the SDI and subsequently collected on stages 1 and 2 by adsorption. As discussed previously, the penetration of the flow through the quartz substrate was significantly larger at lower stages due to larger velocities. The same amount of OC should have been evaporated from the SDI-Al, but the use of denuders prior to the SDI-Q probably accelerated the evaporation of OC from particles by changing the gas-to-particle distribution of organic components (CitationZhang and McMurry 1991; CitationTurpin et al. 2000). It should also be noted that the new D50 values for the SDI-Q gave slightly larger concentrations for the particle size 0.05–0.06 μ m than the SDI-Al (). However, the difference in the D50 values was not able to explain the mode at 0.02–0.1 μ m for OC, neither did the uncertainty of thermal-optical method used for the OC analysis.
FIG. 7 Mass closure measured in Hyytiälä May 7–9, 2004. Note different D50 values for the SDI-Al (mass and inorganic ions) and for the SDI-Q (OC and EC). D50 values from the electric method were used for the SDI-Q. Unanalyzed fraction (white area) consists mainly of hydrogen and oxygen atoms in organic compounds in fine fraction (< 1μ m), and elements in coarse fraction (> 1 μ m, e.g., metal oxides).
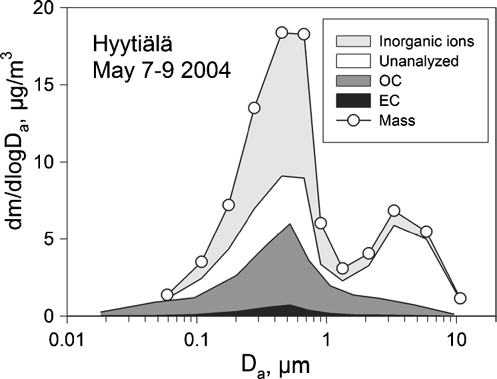
In case of large particles (> 1 μ m), the unanalyzed mass was made of components not analyzed in this study such as crustal metal oxides. The lack of a significant coarse mode for OC might be due to the denuders prior to the SDI. This study was focusing on the submicrometer size range, so the penetration of coarse particles through the denuders was not tested.
CONCLUSIONS AND IMPLICATIONS
According to the results obtained in this study, the collection efficiency of quartz substrate is significantly different from that of aluminum in the SDI. In addition to the change in the D50 values, also the shapes of the efficiency curves are different. This can be explained by collection mechanisms other than inertial impaction. One viable candidate for the excess collection is filtration because high velocity jets penetrate the porous quartz filter surface. The loading (∼ 16 hours) of quartz does not alter the D50 value but decreases the collection efficiency of particles smaller than D50.
Laboratory calibration gave smaller D50 values for the quartz than for the plain impaction plate. As a result the accumulation mode of OC and EC shifted toward smaller particle sizes and was broader than that based on the original D50 values. Also, an additional sub-0.1 μ m mode was obtained for OC whereas the same mode was obtained for EC only in urban environment. The size distributions of OC and EC differed from those of sulfate in the rural site, Hyytiälä, suggesting different sources and formation mechanisms. However, in order to provide reliable size distributions for OC and EC, the split between OC and EC in the thermal-optical analysis needs to be verified because the sample deposits of the SDI are not uniform and the optical detection of charring is difficult. The investigation of the split point was outside the scope of this study. Furthermore, only the new D50 values were used for quartz, which excludes completely the fact that the shape of the collection efficiency curve was far away from ideal. The less steep collection efficiency curves for the quartz lead to an increased overlap between stages. In order to include the shape of the curve in size distribution data, the use of more refined data processing (e.g., an inversion code) is needed.
Strictly speaking, the results of this study are valid only for the SDI. However, for low pressure impactors having velocities and stage pressures similar to those in the SDI (e.g., ELPI, BLPI), the change in particle collection characteristics from flat substrates (e.g., polycarbonate, aluminum) to quartz is most likely very similar. For the MOUDI the cut-off sizes for quartz substrate are not easy to estimate on the basis of this study, since the deposit area under the jet is smaller as are also the jet velocities. In the MOUDI the use of quartz substrates instead of thin films is critical also because of the extremely small jet-to-plate distance.
Acknowledgments
Financial support from the Finnish Funding Agency for Technology and Innovation (Grant no. 40531/04, and the LIPIKA project), the Academy of Finland (Contract no. 201131), and the Maj and Tor Nessling Foundation is gratefully acknowledged.
Notes
a Ratio of particle aerodynamic diameter corresponding 84% and 50% collection efficiency.
b Maenhaut et al. 1996.
c Estimated.
REFERENCES
- Birch , M. E. and Cary , R. A. 1996 . Black Carbon-based Method for Monitoring Occupational Exposure to Particulate Diesel Exhaust . Aerosol Sci. Technol. , 25 : 221 – 241 .
- Berner , A. and Lürzer , C. 1980 . Mass Size Distributions of Traffic Aerosols at Vienna . J. Phys. Chem. , 84 : 2079 – 2083 .
- Carvalho , A. , Pio , C. and Santos , C. 2003 . Water-soluble Hydroxylated Organic Compounds in German and Finnish Aerosols . Atmos. Environ , 37 : 1775 – 1783 .
- Chow , J. C. , Watson , J. G. , Pritchett , L. C. , Pierson , W. R. , Frazier , C. A. and Purcell , R. G. 1993 . The DRI Thermal/Optical Reflectance Carbon Analysis System: Description, Evaluation and Applications in U.S. Air Quality Studies . Atmos. Environ. , 27A : 1185 – 1201 .
- Fujitani , Y. , Hasegawa , S. , Fushimi , A. , Kondo , Y. , Tanabe , K. , Kobayashi , S. and Kobayashi , T. 2006 . Collection Characteristics of Low-pressure Impactors with Various Impaction Substrate Materials . Atmos. Environ. , 40 : 3221 – 3229 .
- Gundel , L. A. , Lee , V. C. , Mahanama , K. R. R. , Stevens , R. K. and Daisey , J. M. 1995 . Direct Determination of the Phase Distributions of Semi-volatile Polycyclic Aromatic Hydrocarbons Using Annular Denuders . Atmos. Environ. , 29 : 1719 – 1733 .
- Hasegava , S. , Hirabayashi , M. , Kobayashi , S. , Morigychi , Y. , Kondo , Y. , Tanabe , K. and Wakamatsu , S. 2004 . Size Distribution and Characterization of Ultrafine Particles in Roadside Atmosphere . J. Environ. Sci. Health , A39 : 2671 – 2690 .
- Hermann , M. and Wiedensohler , A. 2001 . Counting Efficiency of Condensation Particle Counters at Low-pressures with Illustrative Data from the Upper Troposphere . J. Aerosol Sci. , 32 : 975 – 991 .
- Hering , S. V. , Flagan , R. C. and Friedlander , S. K. 1978 . Design and Evaluation of New Low-pressure Impactor. I . Environ. Sci. Technol. , 12 : 667 – 673 .
- Hering , S. V. 1987 . Calibration of QCM Impactor for Stratospheric Sampling . Aerosol Sci. Technol. , 7 : 257 – 274 .
- Hillamo , R. E. and Kauppinen , E. I. 1991 . On the Performance of the Berner Low Pressure Impactor . Aerosol Sci. Technol. , 14 : 33 – 47 .
- Hinds , W. C. 1999 . Aerosol Technology; Properties, Behaviour and Measurement of Airborne Particles. , 124 New York : John Wiley & Johns .
- Huang , C.-H. , Tsai , C.-J. and Shih , T.-S. 2001 . Particle Collection Efficiency of an Inertial Impactor with Porous Metal Substrates . J. Aerosol Sci. , 32 : 1035 – 1044 .
- Huang , C.-H. , Chang , C.-S. , Chang , S.-H. , Tsai , C.-J. , Shih , T.-S. and Tang , D.-T. 2005 . Use of Porous Foam as the Substrate of an Impactor for Respirable Aerosol Sampling . J. Aerosol Sci. , 36 : 1373 – 1386 .
- Jaffrezo , J.-L. , Aymoz , G. and Cozic , J. 2005 . Size Distribution of EC and OC in the Aerosol of Alpine Valleys during Summer and Winter . Atmos. Chem. Phys. , 5 : 2915 – 2925 .
- Kavouras , I. G. and Koutrakis , P. 2001 . Use of Polyurethane Foam as the Impaction Substrate/Collection Medium in Conventional Inertial Impactors . Aerosol Sci. Technol. , 34 : 46 – 56 .
- Kulmala , M. , Hämeri , K. , Aalto , P. P. , Mäkelä , J. M. , Pirjola , L. , Nilsson , E. D. , Buzorius , G. , Rannik , Ü. , Dal Maso , M. , Seidl , W. , Hoffmann , T. , Janson , R. , Hansson , H.-C. , Viisanen , Y. , Laaksonen , A. and O'Dowd , C. D. 2001 . Overview of the International Project on Biogenic Aerosol Formation in the Boreal Forest (BIOFOR) . Tellus , 53B : 324 – 343 .
- Liu , B. Y. H. and Lee , K. W. 1975 . An Aerosol Generator of High Stability . Am. Indus. Hyg. Assoc. J. , 36 : 861 – 865 .
- Loo , B. W. and Cork , C. P. 1988 . Development of High Efficiency Virtual Impactor . Aerosol Sci. Technol. , 9 : 167 – 170 .
- Maenhaut , W. , Hillamo , R. , Mäkelä , T. , Jaffrezo , J.-L. , Bergin , M. H. and Davidson , C. I. 1996 . A New Cascade Impactor for Aerosol Sampling with Subsequent PIXE Analysis . Nucl. Inst. Meth. Phys. Res. B , 109/110 : 482 – 487 .
- Marjamäki , M. and Keskinen , J. 2004 . Effect of Impaction Plate Roughness and Porosity on Collection Efficiency . J. Aerosol Sci. , 35 : 301 – 308 .
- Marple , V. A. and Liu , B. Y. H. 1974 . Characteristics of Laminar Jet Impactors . Environ. Sci. Technol , 8 : 648 – 654 .
- Marple , V. A. , Rubow , K. L. and Behm , S. M. 1991 . A Microorifice Uniform Deposit Impactor (MOUDI): Description, Calibration and Use . Aerosol Sci. Technol , 14 : 434 – 446 .
- Marple , V. A. and Olson , B. A. 1999 . A Micro-Orifice Impactor with Cut-Sizes Down to 10 Nanometers for Diesel Exhaust Sampling Final Report for Grant Number G1145242, G1155242/#2783 Generic Technology Center for Respirable Dust
- Rader , D. J. and Marple , V. A. 1985 . Effect of Ultra-Stokesian Drag and Particle Interception on Impaction Characteristics . Aerosol Sci. Technol , 4 : 141 – 156 .
- Saarikoski , S. , Mäkelä , T. , Hillamo , R. , Aalto , P. P. , Kerminen , V.-M. and Kulmala , M. 2005 . Physico-chemical Characterization and Mass Closure of Size-segregated Atmospheric Aerosols in Hyytiälä, Finland . Boreal Env. Res , 10 : 385 – 400 .
- Sillanpää , M. , Hillamo , R. , Mäkelä , T. , Pennanen , A. S. and Salonen , R. O. 2003 . Field and Laboratory Tests of a High Volume Cascade Impactor . J. Aerosol Sci. , 34 : 485 – 500 .
- Teinilä , K. , Kerminen , V.-M. and Hillamo , R. 2000 . A Study of Size-Segregated Aerosol Chemistry in the Antarctic Atmosphere . J. Geophys. Res. , 105 : 3893 – 3904 .
- Turpin , B. J. , Saxena , P. and Andrews , E. 2000 . Measuring and Simulating Pariculate Organics in the Atmosphere: Problems and Prospects . Atmos. Environ , 34 : 2983 – 3013 .
- Viidanoja , J. , Kerminen , V.-M. and Hillamo , R. 2002 . Measuring the Size Distribution of Atmospheric Organic and Black Carbon Using Impactor Sampling Coupled with Thermal Carbon Analysis: Method Development and Uncertainties . Aerosol Sci. Technol. , 36 : 1 – 10 .
- Virtanen , A. , Marjamäki , M. , Ristimäki , J. and Keskinen , J. 2001 . Fine Particle Losses in Electrical Low-pressure Impactor . J. Aerosol Sci. , 32 : 389 – 401 .
- Winklmayer , W. , Wang , H.-C. and John , W. 1990 . Adaptation of the Twomey Algorithm to the Inversion of Cascade Impactor data . Aerosol Sci. Technol. , 13 : 322 – 331 .
- Wolfenbarger , J. K. and Seinfeld , J. H. 1990 . Inversion of Aerosol Size Distribution Data . J. Aerosol Sci. , 21 : 227 – 247 .
- Yttri , K. E. , Dye , C. , Siørdal , L. H. and Braathen , O.-A. 2005 . Quantification of Monosaccharide Anhydrides by Liquid Chromatography Combined with Mass Spectrometry: Application to Aerosol Samples from an Urban and a Suburban Site Influenced by Small-scale Wood Burning . J. Air & Waste Manage. Assoc. , 55 : 1169 – 1177 .
- Zhang , X. and McMurry , P. H. 1991 . Theoretical Analysis of Evaporative Losses of Adsorbed and Absorbed Species during Atmospheric Aerosol Sampling . Environ. Sci. Technol. , 25 : 456 – 459 .
- Zhang , Q. , Worsnop , D. R. , Canagaratna , M. R. and Jimenez , J. L. 2005 . Hydrocarbon-like and Oxygenated Organic Aerosols in Pittsburgh: Insights into Sources and Processes of Organic Aerosol . Atmos. Chem. Phys. , 5 : 3289 – 3311 .
- Zhang , Q. , Jimenez , J. L. , Canagaratna , M. R. , Allan , J. D. Coe , H. 2007 . Ubiquity and Dominance of Oxygenated Species in Organic Aerosol in Anthropogenically-influenced Northern Hemisphere Midlatitudes . Geophys. Res. Lett , 34 : L13801