Abstract
Most filtration studies have been conducted with spherical particles; however, many aerosol particles are agglomerates of small primary spheres. Filtration efficiency tests were conducted with silver NP agglomerates, with the agglomerate structure controlled by altering the temperature of a sintering furnace. The mobility diameter and mass of the silver NP agglomerates were measured using a differential mobility analyzer together with an aerosol particle mass analyzer. From these measurements, it was found that the fractal-like dimension, D fm, varied from 2.07 to 2.95 as the sintering temperatures was increased from ambient to 600°C. The agglomerates were essentially fully coalesced at 600°C allowing direct comparison of the filtration behavior of the agglomerate to that of a sphere with the same mobility diameter. Other agglomerate properties measured include the primary diameter, the agglomerate length and aspect ratio, and the dynamic shape factor.
Agglomerate filtration modeling with no adjustable parameters has been investigated in terms of diffusion, impaction, and interception. The model results agree qualitatively with the experimental results in the particle size range of 50 to 300 nm. The results indicated that the larger interception length of agglomerates is responsible for the smaller penetration through a fibrous filter in comparison to spherical particles with the same mobility diameters.
INTRODUCTION
There is increased interest in the filtration of nanoparticle (NP) agglomerates, which are made up of clusters or chains of nanosize spherules. Such particles are produced by high temperature processes leading to a solid particulate. Combustion is a primary source including diesel particulate and soot from building fires. Combustion is also used to make a variety of materials in agglomerate form including fumed silica, titanium dioxide, and carbon black. A variety of metallic agglomerates including silver, iron, and copper are produced by the vaporization of the metal and subsequent cooling in a non-oxidative environment.
These agglomerates are a health concern both from an occupational perspective in the production and handling of such particles and as an air pollutant concern from the exposure to agglomerates emitted by internal combustion engines, fires, and industrial combustors. It has been shown that particle surface area is the most important parameter in terms of nanoparticle toxicity (CitationOberdörster et al. 2005). Thus, agglomerates are also expected to be more toxic than spherical particles with the same mass.
One of the primary methods for reducing the exposure to such particles is the use of filtration. There has been a great deal of research on the performance of fibrous filters for spherical particles. Theories have been developed for the penetration of spherical particles through the filter including the effect of particle diffusion, interception, and impaction and these theories have been validated based on experiments on model filter systems. There is much less information on the filtration of NP agglomerates. CitationFu et al. (1990) studied the filtration of chain aggregate aerosols using a screen type filter. The iron oxide chain aggregates had mean lengths in the range of 1,500 nm to 3,200 nm. The primary sphere size ranged from 17 nm to 41 nm. The authors compared their results with predictions based on the Chan-Dahneke model (1981) for the friction coefficient for chain agglomerates. The only unknown parameter needed for predicting the single fiber collection efficiency was the interception parameter. This parameter was estimated by matching the theoretical single fiber efficiency with the experimental results. They found that interception was the dominant deposition mode and that the chain aggregates were preferentially aligned with the flow at the higher flow rates.
CitationLange et al. (1999) measured the filtration efficiency of fibrous filters for carbon agglomerates and for spherical particles as a function of the mobility diameter. They derived a filtration model based on the interception equivalent diameter of agglomerates, which is defined as the diameter of a spherical particle diameter having the same penetration as that of the agglomerate. They showed that the concept of equivalent diameters can be used to predict filter penetration for non-spherical particles if both the interception and mobility diameters are measured and inserted into models for spherical particles.
The focus of our study is the filtration of silver agglomerates. One novel feature is the ability to change the morphology of the agglomerates from an open structure with a fractal dimension less than two to a fully sintered structure with a spherical shape. This allows a direct comparison between the filtration efficiency of the agglomerate and that of a sphere with the same mobility diameter as the agglomerate and the same material density. Another new feature is the characterization of the structure of the agglomerate in terms of the fractal dimension, the maximum length and aspect ratio, and the dynamic shape factor. This information is not available from the previous studies. A third difference is our direct comparison between the measured penetration and the predicted penetration. In other studies, the interception parameter was estimated by a fitting procedure.
NP AGGLOMERATES PENETRATION TEST
shows a schematic diagram of a NP agglomerate penetration test system. It consists of a NP agglomerate generator, a DMA (Differential Mobility Analyzer) for size classification, and a filter penetration measurement system. An electric furnace is used to generate silver nanoparticles from a pure silver powder source (99.999%, Johnson Mattney Electronics). The temperature within the flow tube is 1,150°C with a length of 89 cm and an I.D. of 1.43 cm. Compressed nitrogen gas is used as a carrier gas with a flow rate of 1.5 lpm. The residence time in the tube furnace based on ambient temperature is 5.7 s. The silver powder source located in the center of a heating tube is vaporized and condensed into silver nanoparticles. The average primary sphere size is 16.2 nm with a standard uncertainty (estimated standard deviation) of 3.1 nm. This value is similar to the values of 15 nm and 18.5 nm observed by CitationSchmidt-Ott (1988) and by CitationLall et al. (2006) who used slightly different operating conditions.
These primary particles stick upon collision to form NP agglomerates in an agglomeration chamber located just downstream of the primary particle generation furnace. The residence time of primary particles in the agglomeration chamber is 2 min with a carrier gas flowrate of 1.5 lpm. Downstream of this chamber, the NP agglomerates are sintered in a second tube furnace with sintering temperatures in the range from room temperature to 600°C. The dimensions of the sintering tube are identical to those of the tube in the first oven. As discussed below, the sintering temperature affects the agglomerate morphology.
The NP agglomerates having controlled morphology are next classified by mobility diameter using a DMA. Just before the DMA, the particles are bipolarly charged to the Boltzmann equilibrium charge distribution by passing the particles through a tube containing a Po-210 radioactive source (1.9 × 10−7 Bq, 0.5 mCi). Only charged particles with a narrow range of mobility can pass through the DMA. The particle size is controlled by adjusting the DMA voltage. The fractional penetration through test filters is measured for each mobility particle size from 50 to 300 nm, where the mobility size is the diameter of a sphere with the same drag force as the NP agglomerate. The neutralized silver nanoparticles (by another Po-210 neutralizer) then flow through the test filter and the number of particles upstream and downstream of the filter are measured by two CPCs (Condensation Particle Counter, TSI Model 3760). The NP agglomerates grow into micrometer-size droplets in the supersaturated environment in the CPC where they are counted based on light scattering.
Particle sampling is long enough to detect at least 100 counts downstream of the test filter. Each test is repeated on three separate days, each with a different filter. The standard uncertainty of the mean is 2.1% of the mean value or less for all the penetration measurements.
shows SEM images of NP agglomerates (mobility diameter of 50 and 200 nm) as a function of sintering temperature (room temperature, 200°C and 600°C). It is shown that NP agglomerate morphology can be changed to a nearly spherical shape at a sintering temperature of 600°C. A similar result was obtained by CitationSchmidt-Ott (1988). It also appears that the morphology of the 200 nm agglomerates is more compact at 200°C compared to room temperature though one must view the SEM images carefully since the magnification scale changes for these two images. The apparent increase in agglomerate size at 200°C for the 50 nm spheres is likely a result of the larger fraction of doubly charged agglomerates in this image. It is also apparent from that the morphology at room temperature is chain-like for the 50 nm agglomerates and has a branched chain structure at 200 nm. The chain-like behavior at 50 nm is not surprising because of the small number of primary spheres, about 4 to 8.
FIG. 2 SEM images of NP agglomerates (1st row: 50 nm, 2nd row: 200 nm) as a function of sintering temperature (1st column: room temperature, 2nd column: 200°C, 3rd column: 600°C).
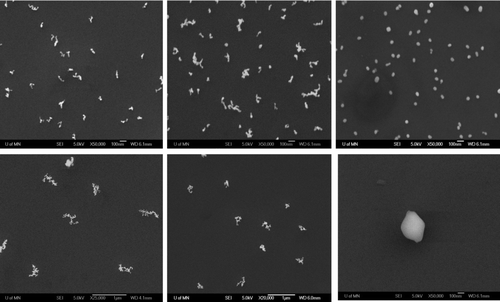
The NP agglomerate penetration results through a test filter (HE 1073, Hollingsworth and Vose Company) are shown in . The standard uncertainty in the measurement points, ± 2.1% of the value, is smaller than the data symbols. Filter HE1073 is a fiberglass filter with an effective fiber diameter of 1.9 μ m, solid fraction of 0.050, and thickness of 0.53 mm. This filter was selected in part because of the small variability in penetration for different filters of this type. A coefficient of variation (CV) of 2.2% was obtained for the penetration of 0.3 μ m dioctylphthalate aerosol at 5.3 cm/s based on measurements with six different filters. The mean penetration was 12.8% (CitationJapuntich 1991).
FIG. 3 NP agglomerate penetration as a function of sintering furnace temperature (test filter: HE 1073, face velocity: 5.3 cm/s, 1st furnace temperature: 1,150°C).
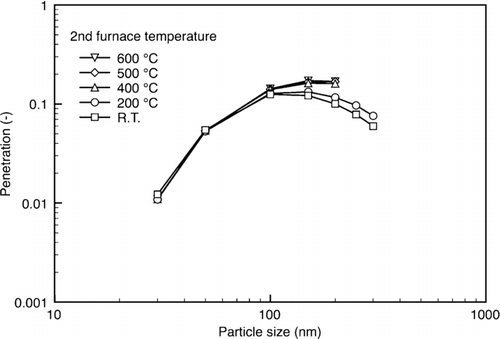
Branched chain agglomerates without sintering show lower penetration than sintered particles for particle sizes larger than 100 nm. The lower penetration of the agglomerates is expected because of the increased interception parameter and is consistent with the study by CitationLange et al. (1999). Additional discussion of the relative penetration will be given below after comparing the measured results to theory. First the characterization of the structural properties of the NP agglomerates is presented. This information is essential for comparing with theory.
STRUCTURAL PROPERTIES OF NP AGGLOMERATE
We have chosen silver agglomerates to study for the following reasons. First the generation and properties of silver agglomerates have been extensively studied (CitationLall et al. 2006; CitationScheibel and Porstendorfer 1983; CitationSchmidt-Ott 1988). Second, the morphology of silver agglomerates can be easily controlled from an open structure to a spherical shape through heating (CitationKu and Maynard 2006). Third, the high density of silver, 10.5 g/cm3, allows us to measure particle mass of relatively small agglomerates.
Our primary approach to the characterization of the agglomerates is by using a DMA and an APM (Aerosol Particle Mass Analyzer) in tandem. These are on-line instruments providing rapid measurements of the mobility diameter and the agglomerate mass. We use the dependence of the mass on mobility diameter as one of our primary characterizations of the agglomerate, and a secondary characteristic being the dynamic shape factor. The other measurements involve the use of the transmission electron microscope to measure the primary sphere size of the agglomerates, the length of the agglomerates, and their widths.
shows the schematic diagram of DMA-APM system to measure mobility diameter and mass of NP agglomerates in real time. The technique involves first selecting particles of a known electrical mobility with a DMA fabricated at the University of Minnesota based on the design of CitationLiu and Pui (1974) and then measuring their mass using a Kanomax aerosol particle mass analyzer, which is based on the design of CitationEhara et al. (1996).
The APM consists of two concentric cylindrical electrodes rotating together at the same controlled speed (∼ 3,000 rpm). A voltage is applied to the inner electrode with the outer electrode grounded. Particles are introduced into the small gap between two electrodes where they experience centrifugal and electrostatic forces. The APM transmits particles of a known mass independent of particle shape or composition. The relationship between the mass of the particle and the rotation frequency ω, voltage V, and radii of the inner and outer electrodes r 1 and r 2 is given by (CitationEhara et al. 1996):
The APM output number concentration versus mass, derived from the voltage and other operating condition of the APM, is shown in for 199 nm silver NP agglomerates. This response curve is not the same as the size distribution function. The APM output number concentration at voltage V 1, N o (V 1), is a convolution of the mass distribution exiting the DMA, G(m), and the APM transfer function, T(m,V 1):
FIG. 5 Mass distribution of 199 nm NP agglomerates measured by DMA-APM system (1st furnace temperature: 1,150°C, 2nd furnace temperature: ambient).
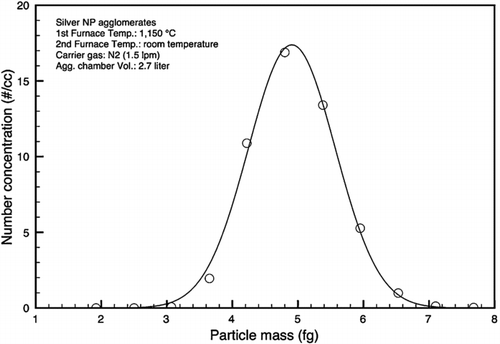
The mass distribution is obtained based on measurements of the APM output number concentration versus voltage using a gradient search optimization to minimize the Chi-squared (χ2) function (CitationEmery 2007). This approach also allows the calculation of the standard uncertainty in the mass based on the fitting procedure. The average uncertainty is 3.4% of the measured mass (see ). It is also found that the error in estimating the peak in the mass distribution from a Gaussian fit of N o (m(V 1)) is on average 0.8% for the range of sizes considered.
TABLE 1 Mean and the standard deviation for the mass, length, and width
CitationEhara et al. (1996) report a standard uncertainty of 6.5% for the mass of 300 nm polystyrene spheres measured with the APM. In our uncertainty analysis given below, we assume a mass standard uncertainty of 7% including the uncertainty associated with the fitting method discussed above. CitationKinney et al. (1991) reported a standard uncertainty of 3.0% in 100 nm polystyrene spheres when using a DMA which had been calibrated for flow and voltage. We assume a slightly larger uncertainty of 4% in our study which includes mobility diameters from 50 nm to 300 nm.
Using the tandem DMA-APM, the masses of the agglomerates were determined as a function of the mobility diameter over a range from about 50 nm up to 300 nm. Data sets were obtained for sintering temperatures ranging from room temperature up to 600°C as shown in . The results for the mean mass and the uncertainty in the mass measurement from the nonlinear fitting are given in .
FIG. 6 NP agglomerate mass versus mobility diameter for various sintering temperatures (1st furnace temperature: 1,150°C, residence time in agglomeration chamber: 2 min).
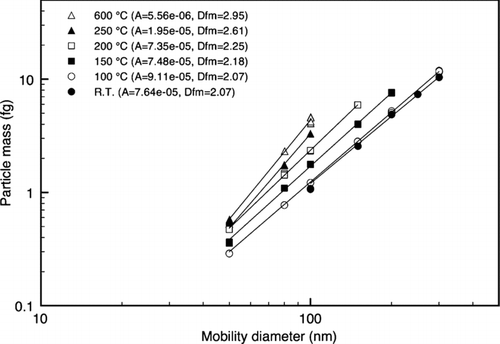
The following power law expression is used for fitting the data:
The quantity D fm is an exponent characterizing the power law dependence of mass on a hydrodynamic length scale rather than a geometric length scale, which is used in defining a fractal dimension. The exponent D fm is not a fractal dimension though it is often presented in aerosol literature as a fractal dimension. We use this same terminology as used by CitationPark et al. (2004), recognizing that D fm is analogous to a fractal dimension and that the subscript m signifies mobility. The power law fits to the data are shown in along with the values of D fm as a function of the sintering temperature. The standard uncertainty in D fm is estimated by adjusting the mass of the agglomerate with d m equal to 300 nm by 7% and then computing the power law expression consistent with this mass and the mass of the agglomerate with d m equal 50 to nm. The change in D fm is ± 0.038. Next the value of d m is adjusted by 4% for the 300 nm sphere and the resulting effect on D fm is ± 0.046. The combined standard uncertainty resulting from both effects is estimated as the square root of the sum of squares with a value of ± 0.060.
The value of D fm at room temperature and for sintering at 100°C, 2.07, is less than the value of 2.18 obtained by CitationSchmidt-Ott (1988) in his study of silver agglomerates over the mobility size range from 20 nm to 90 nm. The current study is for larger agglomerates with mobility size from 50 nm to 300 nm. The Schmidt-Ott results are within 2 standard uncertainties of the mean of the current study.
The particles are collected on a TEM grid by an electrostatic sampler designed for collection of nanosize particles (TSI Model 3089). Sample collection of about 10 minutes is adequate for concentrations above 1,000 part/cm3. For the largest agglomerates with a size of 300 nm and a concentration of about 30 part/cm3, 2 ∼ 5 agglomerates per field of view at 10,000× are collected in two hours.
As mentioned above, TEM analysis is also used to characterize the silver agglomerates. An electron microscope (FEI Tecnai T12) was used for measuring the primary sphere size and the length and width of the agglomerates. The nominal magnification of × 50,000 is used with a resolution of about 0.3 nm per pixel. The primary particles clearly distinguished in the agglomerates are used for analysis. shows a high magnification TEM of the primary spheres. A total of 124 spheres were analyzed. The size distribution is plotted in . The mean particle size is 16.2 nm with a standard uncertainty of 3.1 nm. This is based on the best fit Gaussian to the data. CitationLall et al. (2006) obtained a primary sphere diameter of 18.5 nm with a 3.5 nm standard uncertainty.
FIG. 7 TEM images of NP agglomerates as a function of sintering temperature (a) 50 nm at R.T., (b) 100 nm at R.T., (c) 50 nm at 200°C, (d) 100 nm at 200°C, (e) 50 nm at 600°C, and (f) 100 nm at 600°C.
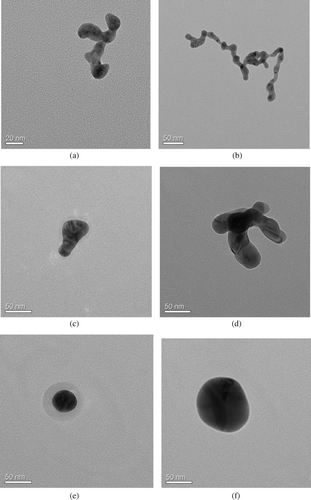
FIG. 8 Primary particle size distribution (1st furnace temperature: 1,150°C, 2nd furnace temperature: ambient, residence time in agglomeration chamber: 2 min).
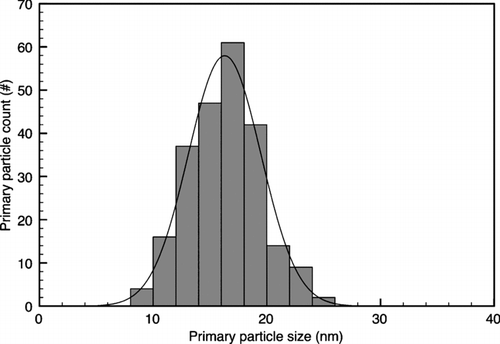
The other measurements from the TEM images are the maximum projected length and the maximum projected width in a direction orthogonal to the length. These quantities were measured for mobility diameters in the range 50 nm to 200 nm for room temperature agglomerates and for agglomerates sintered at 200°C. The length distribution plots for mobility sizes of 50 nm, 100 nm, and 200 nm are shown in . contains the mean values and standard uncertainties for each case. It is seen that a much smaller number of agglomerates are collected for the larger sizes.
FIG. 9 Maximum length distributions of NP agglomerates for various mobility diameters (1st furnace temperature: 1,150°C, 2nd furnace temperature: ambient, residence time in agglomeration chamber: 2 min).
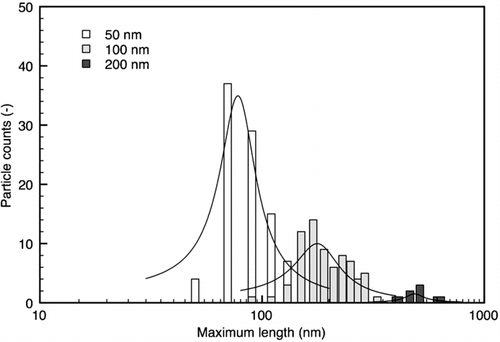
The maximum projected length is plotted versus the mobility diameter in . While the data are limited to three points for each temperature, it is seen that the results are consistent with a power law dependence given by:
Another quantity of interest is the aspect ratio β defined as the maximum projected length of the agglomerate divided by maximum projected width. The distribution of β for NP agglomerates without sintering are shown in to include a symmetrical distribution around a value of 1.65 plus a small fraction of agglomerates with much larger values of β in the range 2.7 to 5. As shown in , the aspect ratio is insensitive to the mobility diameter and is similar for both the NP agglomerates that were not sintered and the ones sintered at 200°C. If one includes the small fraction of agglomerates with large aspect rations, the mean aspect ratio is about 1.75 with a standard uncertainty of about 0.50.
FIG. 11 Aspect ratio analysis of NP agglomerate (1st furnace temperature: 1,150°C, 2nd furnace temperature: ambient, mean value: 1.65).
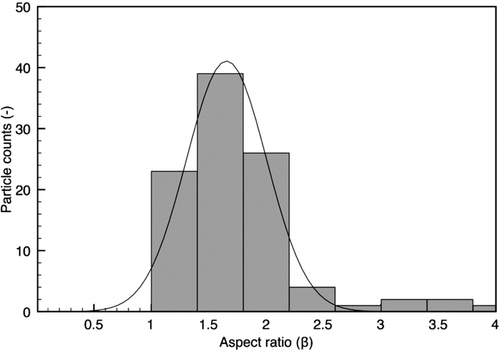
It is of interest to express Equation (Equation3) in terms of L to obtain an estimate of the fractal dimension of the agglomerates. This can be done by making use of Equation (Equation4).
The expression on the right-hand side is the relationship between the mass and the characteristic geometric length scale for a mass fractal. Thus, exponent D fm /γ is the fractal dimension and is found to have a value of approximately 1.77 at room temperature. In the study by CitationLall et al. (2006), fractal dimensions of 1.38 and 1.63 were obtained based on the structure of two individual agglomerates by TEM analysis. CitationKu and Maynard (2006) obtained a projected surface fractal dimension of 1.53 and 1.56 for two individual agglomerates. There is a large uncertainty in our measurements of about 10% because of the small number of mobility sizes included, 3, and the small number of agglomerates sized for the largest mobility size, 8. One also expects the other studies to give a slightly smaller fractal dimension, since they are analyzing 2-D projections of the agglomerates.
The key quantity in characterizing the dynamics of a non-spherical particle is its dynamic shape factor κ. This quantity is equal to the ratio of the friction coefficient of the agglomerate to the friction coefficient of a sphere with the same condensed phase volume as the agglomerate. This quantity has been measured for a variety of agglomerates including small silver agglomerates by CitationSchmidt (1988), diesel exhaust agglomerates by CitationPark et al. (2004), PSL agglomerates by CitationZelenyuk et al. (2006), and carbonaceous smoke particle agglomerates and magnesium oxide smoke particle agglomerates by CitationWu and Colbeck (1996). It has been shown by CitationKasper (1982) and by CitationKelly and McMurry (1992) that κ can be expressed as function of the mobility diameter, d m , and the volume equivalent sphere diameter, which is calculated from the particle's true density and the individual particle mass measured by the APM, d ve .
The Cunningham slip correction factors are for spherical particles with diameters d m (d ve ).
The standard uncertainty in the measurement of the dynamic shape factor, u(κ), can be computed from the uncertainty in d m and m using the law of propagation of uncertainty:
Using Equations (6) and (8), one obtains the following expression for the relative uncertainty in κ:
The relative uncertainty for d m is 0.04 and for m is 0.07. The value of u r (κ) ranges from 0.07 to 0.09.
MODELING OF PARTICLE PENETRATION THROUGH A FILTER
The standard model for spherical particle penetration through a fibrous filter is modified for application to agglomerates. The three properties for the agglomerates needed for the modified model are the mobility diameter, the mass, and the interception particle size. We first give an explicit expression for the standard model for spherical particle penetration and then show the changes needed for a model of NP agglomerate penetration.
The mechanisms for particle deposition considered include diffusion, interception, impaction, and an effect for enhanced interception for a diffusing particle. The expression for the penetration efficiency through a fibrous filter with fiber diameter d f , volume fraction of fiber α, filter thickness t, and total single fiber collection efficiency E Σ is given by (CitationBrown 1993):
where
The following expression, which is consistent with an extensive data base (CitationKirsch and Stechkina 1978), is used for the single-fiber collection efficiency for diffusion:
The Peclet number, Pe, is defined by
The friction coefficient is related to the mobility diameter by the following expression:
This expression is used for both spherical and non-spherical particles.
The expression used for the interception efficiency is given by CitationBrown (1993):
This expression was derived for a spherical particle with diameter d s , for which the interception parameter R was equal to the ratio d s /d f . For agglomerates, we define the dimensionless interception parameter based on the maximum projected length of the agglomerate, L, which can be calculated from Equation (Equation4).
This choice of R 1 is based on the particle rotation time being fast compared to the transit time past the fiber.
In the size region where the collection efficiency is not totally dominated by diffusion, the interaction term E DR between diffusion and interception is as large as the interception efficiency. The interaction terms accounts for the enhanced interception from the particle diffusion motion as it moves in the vicinity of the fiber (CitationHinds 1999).
Because of their increased inertia, the trajectories of larger particles do not follow the gas streamlines. This leads to inertial deposition. The deposition efficiency E i is given by the following expression (CitationYeh and Liu 1974):
The constant J is computed by analysis of the particle trajectory for low Stokes flow:
The Stokes number Stk is a measure of the particle inertia:
The relaxation time of the agglomerates is computed based on the measured mass of the agglomerate and the mobility diameter. The resulting expression for the Stokes number is given by:
COMPARISON OF MODEL PREDICTIONS WITH EXPERIMENTAL RESULTS
As shown in , the penetration predicted by the model is lower than the experimental value for particle sizes below 100 nm, for which the diffusion mechanism is dominant. A similar result was observed by CitationWang et al. (2007), who compared the penetration of spherical silver nanoparticles through standard filter media to the penetration computed using the equation E = 2Pe −2/3. CitationWang et al. (2007) developed an empirical equation E = 0.84Pe −0.43 based on experimental data by CitationKim et al. (2007), which also gives good agreement with the experimental data in the present study. The standard filters used in CitationKim et al. (2007) are the same as those in the present study. We believe that inhomogeneity and random orientations of fibers in the standard filters are important reasons for the discrepancy between the experimental result and the models based on single-fiber efficiency theories.
For mobility diameters greater than 100 nm, the penetration decreases markedly for the room temperature agglomerates compared with the nearly spherical agglomerates sintered at 600°C. The trend observed for the agglomerated “sintered” at room temperature is qualitatively similar to that observed by Lange et al. (1996) for carbon agglomerates. For the mobility diameter of 200 nm, the penetration is a factor of 1.7 lower for the room temperature agglomerates; and its effective interception diameter, estimated as the maximum projected length, is a factor of 2.5 times larger than the mobility diameter. The model predicts a slightly larger decrease, a factor of 2.0 lower for the room temperature agglomerate. As seen for the efficiency factors in , interception is the dominant contributor for these agglomerates. Even with the high density, the impaction mechanism is predicted to contribute only 3% to the overall collection efficiency for the RT agglomerate with d m ; for 300 nm spheres the predicted efficiency is about 27%.
TABLE 2 Efficiency factors for the deposition for each mechanism
In the penetration is plotted versus the agglomerate mass to provide a different perspective on the results. There are fewer points than in , because of the difficulty in measuring masses corresponding to mobility sizes less than 50 nm and because of limited mass data for the larger agglomerate sizes. For a fixed mass in the mass range less than 2 fg, the more compact particles (higher sintering temperature) have a larger diffusion coefficient and lower penetration. As the mass increases, there is a crossover in the penetration with the lowest density agglomerates having the lowest penetration as a result of the much larger interception cross section. The decrease in penetration for the lower density agglomerates compared to the higher density is more striking as a function of mass than as a function of the mobility diameter. For example, at a mass of about 10 fg, the ratio of penetration at room temperature to a sintering temperature of 200°C is 0.46 compared to a ratio of 0.75 for mobility diameter of 200 nm.
The model predictions are consistent with the experimental observations, but they do not provide quantitative agreement with the experiment. It would be desirable to repeat these measurements using a more ideal filter, such as a stainless steel screen mesh, to eliminate the anomalous filtration behavior in the limit of the diffusion dominated collection efficiency.
We are not able to determine the interception equivalent diameter by the method of CitationLange et al. (1999) because of the limited size range of the fully sintered spheres data. There may also be difficulty using their method for such high density particles, 1.05 × 104 kg/m3 for larger particles, because of interference from deposition via impaction.
CONCLUSIONS
Our study shows that NP agglomerate particles have lower penetration through filters due to their larger interception length in comparison to spherical particles with the same mobility diameters. The largest decrease in penetration for the agglomerate was a factor of 1.7 for a mobility diameter of 200 nm. By sintering the agglomerates to a spherical shape, we are able to obtain a direct comparison of NP agglomerate filtration to compact spheres of the same material. The NP agglomerates were characterized in terms of their dynamic shape factor and fractal-like dimension D fm by using a tandem DMA/APM measurement system. The value of D fm increased from about 2.07 to almost 3.0 as the sintering temperature was increased from ambient temperature to 600°C. Model calculations for the penetration through a fibrous filter with no adjustable parameters provide agreement with the experimental trends. Additional study is needed using a more idealized filter such as a screen mesh to avoid the anomalous filtration efficiency in the diffusion region observed with the fiber glass filter.
The authors thank the support of members of the Center for Filtration Research: 3M Corporation, Cummins Filtration Inc., Donaldson Company, Inc., E. I. du Pont de Nemours and Company, Samsung Semiconductor Inc., Shigematsu Works CO., LTD, TSI Inc., and W. L. Gore & Associates and the affiliate member National Institute for Occupational Safety and Health (NIOSH).
REFERENCES
- Brown , R. C. 1993 . Air Filtration: An Integrated Approach to the Theory and Applications of Fibrous Filters , New York : Pergamon Press .
- Chan , P. F. and Dahneke , B. 1981 . Free Molecule Drag on Straight Chains of Uniform Spheres . J. Appl. Phys. , 52 : 3106 – 3110 .
- Ehara , K. , Hagwood , C. and Coakley , K. J. 1996 . Novel Method to Classify Aerosol Particles According to Their Mass-to-Charge Ratio-Aerosol Particle Mass Analyze . J. Aerosol Sci. , 27 : 217 – 234 .
- Emery , M. S. 2007 . “ Theoretical Analysis of Data from DMA-Apm System ” . In Mechanical Engineering , Minneapolis, , USA : University of Minnesota .
- Fu , T. H. , Cheng , M. T. and Shaw , D. T. 1990 . Filtration of Chain Agglomerate Aerosols by Model Screen Filter . Aerosol Sci. & Technol. , 13 : 151 – 161 .
- Hinds , W. C. 1999 . Aerosol Technol.: Properties, Behavior, and Measurement of Airborne Particles , New York : John Wiley & Sons .
- Japuntich , D. A. 1991 . Particle Clogging of Fibrous Filters , Loughborough, , U.K. : Loughborough University of Technology .
- Kasper , G. 1982 . Dynamics and Measurements of Smokes I: Size Characterization of Nonspherical Particles . Aerosol Sci. & Technol. , 1 : 187 – 199 .
- Kelly , W. P. and McMurry , P. H. 1992 . Measurement of Particle Density by Inertial Classificationof Differential Mobility Analyzer-Generated Monodisperse . Aerosol Sci. & Technol. , 17 : 199 – 212 .
- Kim , J. H. , Mulholland , G. W. , Kukuck , S. R. and Pui , D. Y. H. 2005 . Slip Correction Measurements of Certified PSL Nanoparticles Using a Differential Mobility Analyzer (Nano-DMA) for Knudsen Number from 0.5 to 83 . J. Res. Natl. Inst. Standard Technol. , 110 : 31 – 54 .
- Kim , S. C. , Harrington , M. and Pui , D. Y. H. 2007 . Experimental Study of Nanoparticle Penetration through Commercial Filter Media . J. Nanoparticle Res. , 9 : 117 – 125 .
- Kinney , P. D. , Pui , D. Y. H. , Mulholland , G. W. and Nelson , P. B. 1991 . Use of the Electrostatic Classification Method to Size 0.1 μm Srm Particles-a Feasibility Study . J. Res. Natl. Inst. Standard Technol. , 96 : 147 – 176 .
- Kirsch , A. A. and Stechkina , I. B. 1978 . The Theory of Aerosol Filtration with Fibrous Filters , New York : John Wiley &Sons .
- Ku , B. K. and Maynard , A. D. 2006 . Generation and Investigation of Airborne Silver Nanoparticles with Specific Size and Morphology by Homogeneous Nucleation, Coagulation and Sintering . J. Aerosol Sci. , 37 : 452 – 470 .
- Lall , A. A. , Seipenbusch , M. , Rong , W. and Friedlander , S. K. 2006 . On-Line Measurement of Ultrafine Aggregate Surface Area and Volume Distributions by Electrical Mobility Analysis: ?. Comparison of Measurements and Theory . J. Aerosol Sci. , 37 : 272 – 282 .
- Lange , R. , Fissan , H. and Schmidt-Ott , A. 1999 . Predicting the Collection Efficiency of Agglomerates in Fibrous Filter . Particle & Particle Systems Characterization , 16 : 60 – 65 .
- Liu , B. Y. H. and Pui , D. Y. H. 1974 . A Submicron Aerosol Standard and the Primary, Absolute Calibration of the Condensation Nuclei Counter . J. Colloid Interface Sci. , 47 : 155 – 171 .
- Oberdörster , G. , Oberdörster , E. and Oberdörster , J. 2005 . Invited Review: Nanotoxicology: An Emerging Discipline Evolving from Studies of Ultrafine Particles . Environ. Health Perspect. , 113 : 823 – 839 .
- Park , K. , Kittelson , D. B. and McMurry , P. H. 2004 . Structural Properties of Diesel Exhaust Particles Measured by Transmission Electron Microscopy (TEM): Relationships to Particle Mass and Mobility . Aerosol Sci. & Technol. , 38 : 881
- Scheibel , H. G. and Porstendorfer , J. 1983 . Generation of Monodisperse Ag- and NaCl- Aerosols with Particle Diameters between 2 and 300 Nm . J. Aerosol Sci. , 14 : 113 – 126 .
- Schmidt-Ott , A. 1988 . New Approaches to In Situ Characterization of Ultrafine Agglomerates . J. Aerosol Sci. , 19 : 553 – 563 .
- Wang , J. , Chen , D. R. and Pui , D. Y. H. 2007 . Modeling of Filtration Efficiency of Nanoparticles in Standard Filter Media . J. Nanoparticle Res. , 9 : 109 – 115 .
- Wu , Z. and Colbeck , I. 1996 . Studies of the Dynamic Shape Factor of Aerosol Agglomerates . Europhysics Letters , 33 : 719 – 724 .
- Yeh , H. C. and Liu , B. Y. H. 1974 . Aerosol Filtration by Fibrous Filters . J. Aerosol Sci. , 5 : 191 – 217 .
- Zelenyuk , A. N. , Cai , Y. and Imre , D. G. 2006 . From Agglomerates of Spheres to Irregularly Shaped Particles: Determination of Dynamic Shape Factors from Measurements of Mobility and Vacuum Aerodynamic Diameters . Aerosol Sci. & Technol. , 40 : 197 – 217 .