Abstract
In this study, we show that black carbon (BC) mass concentrations measured by different techniques are consistent and traceable. First, we present the volatilities of 13 organic compounds passed through a heated inlet. These data were used to quantify the interference of organic aerosols on the BC measurement techniques. The masses of the refractory particles that incandesce (m*ref) were used to calibrate BC mass measured by a single-particle soot photometer (SP2), which uses laser-induced incandescence. This calibration was influenced little by refractory organics and agreed well with that of fullerene soot, which indicates the consistency of the standards. We estimated the interference of pyrolyzed refractory organics on the BC measured with a filter-based absorption photometer continuous soot monitoring system (COSMOS) with a heated inlet to be small in Asia. This was also confirmed by the stable mass absorption cross section (MAC) obtained by the high correlations between BC mass concentrations measured by COSMOS (M COSMOS) and those measured by the thermal-optical transmittance method (M TOT) (CitationKondo et al. 2009). M COSMOS was also compared with total BC mass concentrations measured with an SP2 (M SP2) in Tokyo in 2009. M COSMOS and M SP2 were highly correlated (r 2= 0.97) and agreed to within about 10% on average. These results demonstrate that M SP2, M COSMOS, and M TOT were nearly identical. Use of the masses of incandescing refractory BC particles for calibration of BC mass concentrations determined by different techniques gave consistent results.
1. INTRODUCTION
Black carbon (BC) aerosols have been extensively studied because they efficiently absorb solar visible radiation and contribute to the radiative forcing of the atmosphere on regional and global scales (IPCC 2007; CitationRamanathan et al. 2007). Elevated BC concentrations in source regions have deleterious impacts on human health (CitationLighty et al. 2000; CitationMcCreanor et al. 2007). Reliable measurements of BC mass concentrations are a prerequisite for improved evaluation of these effects. Various techniques have been used to measure BC mass concentrations in the field (CitationSchmid et al. 2001; CitationHitzenberger et al. 2006; CitationSlowik et al. 2007; CitationKanaya et al. 2008). Assessment of possible systematic differences between the measurements obtained by the various techniques is necessary, particularly with regard to their uncertainties and traceability to appropriate standards. To achieve this goal, we need to establish methods for absolute and systematic calibration of BC mass concentrations.
FIG. 1 Experimental setup for the measurement of the size distributions of organic aerosols with or without the heated inlet. Total numbers of particles were measured by a CPC after size selection by a DMA.
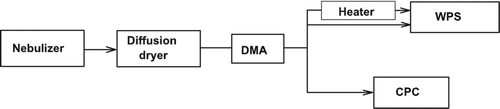
In recent years, a technique has been developed to measure the masses of individual BC particles by means of laser-induced incandescence (LII) (CitationGao et al. 2007; CitationSchwarz et al. 2006; CitationSchwarz et al. 2008; CitationMoteki and Kondo 2007, Citation2008, Citation2010; CitationMoteki et al. 2009). The measurement of BC by means of a single-particle soot photometer (SP2), which uses LII, has been shown to be unperturbed by internal mixing of BC. The technique is not influenced by the coexistence of other types of aerosols, including organic aerosols (OA). Therefore, we used this technique as a basis for measuring BC mass concentrations. We used ambient BC mass to calibrate our SP2 mass measurement.
The main focus of this article is to show the consistency of the different measurement techniques of ambient BC mass concentrations. The techniques used are the LII technique, the refractory mass method (RMM; Kondo et al. 2006), the thermal-optical transmittance (TOT) method (CitationBirch and Cary 1996; CitationLim and Turpin 2002; CitationKondo et al. 2006), and the filter-based photo-absorption technique, specifically the continuous soot monitoring system (COSMOS) (CitationMiyazaki et al. 2008; CitationKondo et al. 2009). The BC measurement techniques are summarized in . The symbols and acronyms used in this article are summarized in .
TABLE 1 Summary of the measurement techniques for BC mass concentration used in this study
TABLE 2 Summary of the variable symbols and acronyms used in this study
The physical and chemical properties of BC and coexisting aerosols influence the uncertainties of the BC measurements and the degree of the interferences strongly depends on measurement principle. First, the variabilities in the microphysical properties of BC, including size, morphology, and mixing state, can cause changes to the response to BC instruments (CitationKondo et al. 2009; CitationNakayama et al. 2010; CitationMoteki et al. 2010b).
Secondly, the coexistence of aerosols other than BC can cause significant uncertainties in the BC measurements, depending on their chemical properties. OA in particular is known to cause significant uncertainties, namely, uncertainties in the separation of BC and organic carbon (OC) in the TOT method (CitationBoparai et al. 2008), enhancement of absorption (lens effect) (absorption photometers except for COSMOS) (CitationSubramanian et al. 2007; CitationCappa et al. 2008; CitationLack et al. 2008), and charring of OA by heating the sample air (COSMOS).
In this study, we conducted detailed laboratory studies of the characteristics of vaporization and pyrolyzation of OA. Based on these data, we have assessed the effect of OA on ambient BC measurements. We show that BC mass concentrations measured by these techniques are consistent and traceable, as indicated by new measurements and our previously published results. The published results include detailed comparisons of the BC measurements by the TOT and filter-based photo-absorption techniques at six locations in Asia (urban and suburban Tokyo in Japan, Jeju Island in Korea, rural Guangzhou and Beijing in China, and Bangkok in Thailand). Here we investigate the relationships between the results obtained from the different techniques from the perspective of our current understanding. The effects of microphysical properties on these measurements are also taken into account.
TABLE 3 Ratios of the volumes of organic particles downstream of the heated (400°C) and unheated inlets (heated/unheated) for different mobility diameters
2. HEATED INLET
We start with the description of a heated inlet, because it was used for the RMM and COSMOS measurements and for the calibration of an SP2, as discussed in section 3. The heated inlet was made of a stainless steel tube with a 3/8-inch outer diameter and 0.049 inches thick. A 21-cm section of the inlet line was heated at 400°C, and the residence time of particles in this section was about 0.3 s for a sample flow rate of 0.7 STP liter min–1 (CitationKondo et al. 2009). Changes in the mass concentration of aerosols with diameters less than about 1 μm (PM1) were measured using an Aerodyne aerosol mass spectrometer (AMS) (CitationKondo et al. 2009). Almost all the mass of inorganic compounds and more than 90% of the mass of organic compounds detected by the AMS evaporated in the heated inlet. However, we need to characterize the volatilities of different organic species through the heated inlet more quantitatively for the interpretation of the BC data obtained by the different techniques.
For this purpose, we measured the volatilities of 13 different organic compounds using the experimental setup shown in . The temperature of the heated inlet was set to be 300° and 400°C. We tested three saccharides: levoglucosan (C6H10O5), glucose (C6H12O6), and sucrose (C12H22O11); five diacids: malonic acid (HOOC(CH2)(COOH)), succinic acid ((HOOC(C2H2)2COOH), adipic acid (HOOC(CH2)4COOH), pimelic acid (HOOC(CH2)5COOH), and azelaic acid (HOOC(CH2)7COOH); one aromatic acid: phthalic acid HOOC(C6H4)(COOH)); and four humic-like substances (HULIS): Suwannee River Humic Acid II (SRHA II), and Suwannee River Fulvic Acid I (SRFA I), Suwannee River Fulvic Acid II (SRFA II), and Pahokee Peat Fulvic Acid (PPFA), as summarized in , together with their molecular weights (MW). The HULIS were from the International Humic Substances Society.
FIG. 2 Changes in the number size distributions of organic aerosols with changes in the temperature of the inlet (300° and 400°C) for organic species.
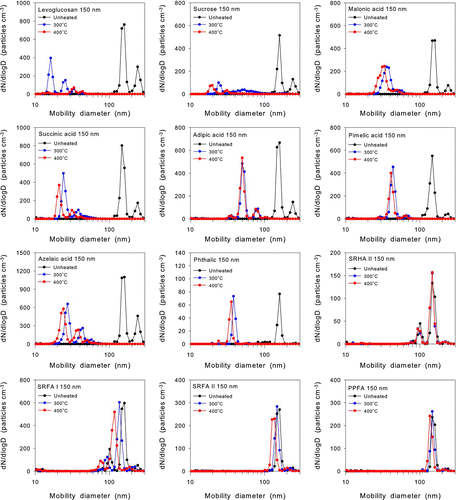
The organic compounds were dissolved in water without significant changes in water temperature to avoid possible changes in chemical characteristics. Aerosol particles generated by a nebulizer setup were dried using a diffusion dryer and then passed through a conductive elastic tube to a DMA (Model 3081, TSI Inc., MN, USA) for mobility-size selection. The flow of the nearly mono-modal organic particles was split into three flows (). One flow was used to measure the total aerosol particle concentration in the sample air with a condensation particle counter (CPC; Model 3022, TSI Inc., MN, USA). The size of organic particles selected by the DMA ranged from 100 to 200 nm, depending on the organic species.
The number size distributions of organic particles pre-selected by the first DMA were measured with a wide range particle spectrometer (WPS; Model 1000 XP, MSP Corporation, MN, USA) operated with a flow rate of about 1 liter min–1 (). The size range measured was between 10 and 500 nm. The number concentrations of the particles measured by the DMA + CPC and WPS with the unheated inlet agreed to within 5%.
shows size distributions as a function of mobility diameter with and without the inlet heated to 300° and 400°C for 12 organic compounds for particles with a mobility diameter of 150 nm, pre-selected by the DMA. Because the WPS system was equipped with its own charger for its internal DMA (second DMA), doubly charged particles by the first DMA were measured as particles larger than 150 nm by the WPS, as notably seen for some species, including sucrose and levoglucosan. The size distributions shown in are corrected for the combined effect of charging by the first and second DMA systems.
The remaining volume fractions downstream of the heated inlet are summarized in . At 400°C, the diameters of levoglucosan, adipic acid, pimelic acid, azelaic acid, and phthalic acid decreased by factors of about 4 to 10. More than 99% of their initial volumes were lost by the heating. In contrast, the changes in the sizes were much smaller for the HULIS, as shown in . Correspondingly the changes in their volumes were as low as about 20–60%. At 300°C, the changes in the volumes were about 10–30%. The observed low volatilities of the HULIS and the high volatilities of the other low-MW organics are generally consistent with those observed by the thermograms of these compounds collected on filters (CitationMiyazaki et al. 2007; CitationBoparai et al. 2008). We also discuss this point in detail in section 3.2.
3. BC MEASUREMENT TECHNIQUES
3.1. SP2 Method
We used an SP2 (CitationSchwarz et al. 2006, Citation2008; CitationGao et al. 2007; CitationMoteki and Kondo 2007, Citation2008, Citation2010; CitationShiraiwa et al. 2008) to measure the BC mass size distribution. Each BC particle sampled by the SP2 was irradiated by a laser beam at a wavelength of 1064 nm and thus heated to incandescence. The SP2 monitors LII signals in two distinct visible bands (λ= 300–550 nm and 580–710 nm). In addition, laser light scattered by individual particles was detected by two avalanche photodiodes to measure the sizes and number concentrations of light scattering particles.
BC particles can be identified by the ratio of LII intensities of the two visible bands, because the ratio is a proxy for the vaporization temperature of the particles. The vaporization temperatures of ambient BC were measured to be about 4000 K (CitationSchwarz et al. (2006). The BC mass for each particle (m SP2) was estimated from the LII peak intensity. We measured the LII peak intensities of monodisperse BC aerosols that were mass selected by an aerosol particle mass analyzer (APM; Model-302, KANOMAX, Inc., Japan) with a 400°C heated inlet (CitationMoteki and Kondo 2007). shows the experimental setup for the measurements of the ambient BC and other types of BC including fullerene soot particles. The BC particles, except for ambient BC, were aerosolized from a water suspension with a pneumatic nebulizer. The diameters of the coated BC particles (shell diameters, D p) were derived using the scattering signals for D p. A detailed description of the method to derive D p is given in the Appendix.
The dependence of the LII intensity on particle microphysical properties was formulated by CitationMoteki and Kondo (2010) and is summarized in the Appendix. The relationships between the peak LII intensity and the mass of BC particles were measured for fullerene soot (Alfa Aesar, USA), colloidal graphite (Alfa Aesar), Aquadag (Acheson, USA), glassy carbon (Tokai Carbon, Japan), glassy carbon (Alfa Aesar, USA), Aqua-Black 001 (Tokai Carbon, Japan), Aqua-Black 162 (Tokai Carbon), and ambient BC in Tokyo (CitationMoteki and Kondo 2010). The relationships were very similar for fullerene soot and ambient BC.
The density of fullerene soot was measured using a helium pycnometer (Gas Pycnometer: Model Accupyc 1340, Micromeritics, GA, USA). The volume of fullerene soot is measured by replacing its void space with helium. The density is determined with independent and simultaneous measurements of its mass, which was about 0.2 g. The measurements were made three times. Each measurement consisted of 10 measurement cycles, providing 10 data points. The average density measured in the three data sets was 1.718 ± 0.004 g cm–3. The estimate of the mass equivalent diameter of BC (D BC) is based on this value.
BC in ambient air was extracted by vaporization of the condensed compounds coating the BC particles by means of the heated inlet mentioned in section 2. The SP2 calibration using ambient BC was made for several hours on November 10, 2009. The purity of the extracted BC estimated from the scattering signals (Appendix) was very high, as discussed by CitationMoteki et al. (2010a). While the heated-inlet-APM output includes both BC and possibly HULIS, the Gaussian mean mass of the output should be the same for all particle types, if they are externally mixed. Since only incandescent, temperature-ratio-filtered BC particles are detected by the SP2 and used for the SP2
calibration, HULIS externally mixed with BC does not affect this calibration.
Now we evaluate errors due to HULIS internally mixed with BC. The median shell/core ratio (D p/D BC) of the extracted BC has been estimated to be 1.00–1.03 for D BC= 160–240 nm, indicating that the volume fraction of the coating materials was less than 9%. About 20–60% of the volume of the 4 kinds of HULIS was lost through the heated inlet (section 2. The density of the HULIS is 1.5 g cm–3 (section 3.4. Assuming these values as typical for refractory organics and assuming the density of BC to be 1.7 g cm–3, the error in extracting refractory BC mass is estimated to be less than about 10% (9 × (1.5/1.7) × 0.8 = 6%).
We also make another estimate. The median value of the shell/core ratio (D p/D BC) of ambient BC in Tokyo was about 1.1 in August and September 2009, as shown in section 4. This means that the ratios of the volume of coating materials to that of BC were about 0.3. It is very unlikely that all the coating compounds were refractory organic compounds, considering that the coating thickness of BC was correlated with the mass concentrations of PM1 inorganics and organics (CitationShiraiwa et al. 2007). PM1 organic mass fractions in Tokyo were about 0.4 in summer and winter (CitationKondo et al. 2010). The mass concentrations of the sum of PM1 aerosols (non-refractory aerosols + BC) agreed to within 10% with the independent gravimetric measurement by a Tapered Element Oscillating Microbalance (TEOM; Rupprecht and Patashnick Co., Albany, NY) instrument in summer (CitationKondo et al. 2007), indicating that less than 25% (= 10%/0.4) of the mass of organics was refractory. Therefore, the influence of refractory organic compounds on the extracted BC mass should have been less than about 10% (= 25 × 0.3 × (1.5/1.7) × 0.8 = 5%), assuming a density of HULIS of 1.5 g cm–3.
This extraction procedure means that the definition of the mass of ambient BC is the mass of incandescent aerosols refractory at 400°C (m*ref). The m*ref data for ambient BC were not interfered by other incandescing aerosols like metal particles, because the ratios of the LII in the two visible bands were always similar for laboratory BC particles. The uncertainty of m*ref is about 10%.
shows plots of the LII peak intensity versus m*ref and the mass of fullerene soot (m full) measured by CitationMoteki and Kondo (2010). We selected only ambient BC and fullerene soot among all the other types of BC for this plot in order to demonstrate clearly their similarity in the LII peak intensity–m*ref
relationships. The values of m full and m*ref ranged up to 350 femtograms (fg) and 230 fg, respectively. The LII peak intensity increased linearly with mass up to about 20 fg. At higher mass, the relationships deviated from linearity. These results agree with the theoretically predicted results, as discussed above.
We attributed the similarity of the LII response of fullerene soot and ambient BC to the similarity in their microphysical properties, namely their refractive indices and particle shapes (CitationMoteki et al. 2010a; CitationMoteki and Kondo 2010). We used the LII peak intensity–m*ref relationship for ambient BC to derive the BC size distributions throughout the present study. It should be stressed here that M SP2 calibrated by m full is nearly identical to M SP2 calibrated by m*ref. In this way, M SP2 is fully traceable to the calibration standards (m*ref). The D BC detection range of the SP2 used for this study was 70–920 nm.
3.2. Refractory Mass Method
The size distribution of the mass of the PM1 refractory particles (m ref) was measured with a scanning mobility particle sizer (SMPS; Model 3034, TSI Inc., MN, USA) combined with a 400°C heated inlet on the campus of the Research Center for Advanced Science and Technology (RCAST), the University of Tokyo, Tokyo, in the summer of 2004. Ambient air was introduced into a cyclone with a 50% effective cut-off aerodynamic diameter of 1 μm (PM1 cyclone) to exclude coarse particles. The observation site was located about 2 km from highways with large traffic volumes. BC at this location is strongly influenced by emissions from heavy-duty diesel vehicles (CitationKondo et al. 2006).
The SMPS is composed of a differential mobility analyzer (DMA; Model 3081, TSI Inc., MN, USA) and a CPC. The mobility diameter (D m) of the SMPS was converted to mass equivalent diameter (D me) with an APM. The DMA provides a slice of aerosol with a reduced range of D m from the ambient distribution. The APM then identifies the single mass per particle that best represents the mass distribution of the slice. The actual mass per particle varies widely with the change in D m, and this constitutes the M ref–D m and D me–D m relationships. The details of the method used to calibrate the DMA–APM system are given in CitationKondo et al. (2006).
Mono-modal size distributions were fitted to the data of mass size distribution of m ref(dm ref/dlogD me) for D m< 500 nm (D me< 300 nm). The mono-modal mass size distribution of m ref was integrated to obtain the total BC mass concentration in this mode (m ref). This procedure excluded dust, carbonate, and sea salt with D me> 300 nm from M ref. In fact, Na+ constituted a very minor (about 1%) fraction of PM1 aerosol mass (CitationTakegawa et al. 2005), showing that M ref was influenced little by sea salt particles.
The absolute accuracy of the APM depends on the accuracy of the estimate of the masses of polystyrene latex (PSL) particles used for calibration. The uncertainty in the masses of submicron PSL particles is about 5%, according to the manufacturer of the PSL. The accuracies of M ref and M ref are therefore about 5%. It should be noted here that M ref and M ref were measured gravimetrically and do not depend on the density of BC assumed to derive the D me–D m relationship.
3.3. TOT Method
The total mass of PM1 BC was also measured by a semi-continuous elemental carbon (EC)-organic carbon (OC) analyzer (Model RT3052, Sunset Lab., Oregon). These measurements were conducted simultaneously with the M ref measurements at RCAST. The BC mass concentrations (M TOT) were derived by the TOT method on the basis of the National Institute for Occupation Safety and Health protocol (CitationKondo et al. 2006; CitationHan et al. 2009). The accuracy of total carbon measurements is about 5%. The overall accuracy of the M TOT measurement was estimated to be 22% from the uncertainties associated with the sensitivity calibration, aerosol sampling, and temperature protocol.
The volatilities of organic compounds can be measured from thermograms of TOT. We relate the volatility data obtained from the TOT and those measured with the heated inlet in section 2 to assess the uncertainty of the COSMOS measurement. In the oven of the TOT, the OC collected on filters evolved in He gas during the four heating steps of 300°C (Step-1: 75 s duration), 450°C (Step-2: 60 s), 600°C (Step-3: 60 s), and 870°C or 750°C (Step-4: 150 s) were defined as OC1, OC2, OC3, and OC4. The OC released at 870°C in He/O2 mixtures (Step-5: 240 s) was defined as OC5. OC4 and OC5 agreed to within 10% for the different temperatures during Step-4, and we use the results for 870°C for discussion. During Step-5, BC was oxidized in the oven. Namely, OC5 represents the fraction of OC that is resistant to volatilization in the He mode (including carbon that undergoes charring) and requires the same conditions as BC evolution in the thermogram (CitationHuntzicker et al. 1982; Cadle et al. 1980).
For atmospheric sampling, OC5 cannot be estimated in the same way as described above, because BC coexists with OC. Instead, we define pyrolyzed carbon (PC) as the amount of carbon released between the start of Step-5 and the time when the laser transmittance through the filter recovered to the initial value. During this time, we measured the thermograms of SRHA II, SRFA I, SRFA II, and PPFA, as shown in . The transmittance of TOT largely decreased during Steps-3 and 4 and recovered during Step-5, indicating that these species were pyrolyzed mainly during Steps-3 and 4. The fractions of OC1-OC5 are summarized in , together with the values of levoglucosan, pimelic acid, and azelaic acid, shown in CitationMiyazaki et al. (2007). The thermograms of some other low-MW organics were also given by CitationMiyazaki et al. (2007).
There are some differences in the OC5 fraction for SRFA I measured by CitationMiyazaki et al. (2006) and the present work (36% versus 54%). The causes of this difference are not understood, although some difference in the samples used could be a possibility. We used the present value in estimating the performance of COSMOS because the same sample was used for this purpose. It should be noted here that the OC5 fractions (F OC5) exceeded 40% only for the HULIS, while the F OC5 of the other organic species were less than 6%. These high F OC5 values of the HULIS correspond quite well to their distinct low volatilities, discussed in section 2.
3.4. COSMOS Method
3.4.1. Measurement Principle
Concentrations of ambient BC have also been measured by means of photo-absorption photometry. This technique requires conversion of the measured absorption coefficient (b abs(λ)) at wavelength λ to BC mass concentration assuming a certain mass absorption cross section (σabs). However, coating of BC with relatively volatile compounds and the coexistence of light-scattering particles can contribute greatly to variation in σabs (CitationLack et al. 2008; CitationCappa et al. 2008; CitationKondo et al. 2009). This difficulty is overcome by removal of almost all the mass of volatile aerosol components before the BC particles are collected on filters. The volatiles are removed by means of a 400°C heated inlet operated with a residence time of about 0.3 s, as discussed in section 2.
TABLE 4 Summary of thermograms of organic species standards. The data for levoglucosan, pimelic acid, and azelaic acid were taken from CitationMiyazaki et al. (2007)
For the present study, we measured mass concentrations of BC with a filter-based absorption photometer COSMOS. Details of the basic performance of the COSMOS instrument have been reported elsewhere (CitationMiyazaki et al. 2008; CitationKondo et al. 2009). In brief, the instrument measures the absorption coefficient (b 0) at a wavelength of 565 nm from the change in transmission through a filter loaded with BC particles after removal of volatile compounds. Operationally, b 0 is determined with the COSMOS system by the following equation,
By means of laboratory experiments using size-selected nigrosin (C48N9H51) particles, we observed that the amplification of BC photo-absorption by multiple scattering increases with the decrease in D BC (CitationKondo et al. 2009; CitationNakayama et al. 2010). Large BC particles do not penetrate as deeply into the filter fibers as do smaller particles, and large particles are situated in locations where the radiation field is influenced by less-frequent multiple scattering. The decrease in the frequency of multiple scattering with the increase in D BC decreases the photo-absorption by BC, resulting in a need of smaller correction for multiple scattering. This size dependence was also confirmed quantitatively by theoretical calculations for spherical photo-absorbing particles on the basis of a radiative transfer model (CitationMoteki et al. 2010b). Here we used the following equation for f fil based on CitationNakayama et al. (2010)
One-minute averaged b abs was converted to BC mass concentration (M COSMOS) using σabs:
FIG. 6 Experimental setup for the measurement of the absorption coefficient of organic aerosols by means of two COSMOS instruments. COSMOS I was mounted downstream of a heater, and COSMOS II was operated without a heater. Total numbers of particles were measured by a CPC after size selection by a DMA.
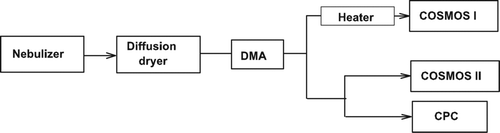
FIG. 7 Plots of equivalent mass concentrations of BC measured by COSMOS with an inlet heated to 300°C and 400°C and without a heated inlet versus the volume concentrations of organic species. The equivalent mass concentrations of BC were derived from the observed absorption coefficients by assuming a mass absorption cross section of 5.4 m2 g–1.
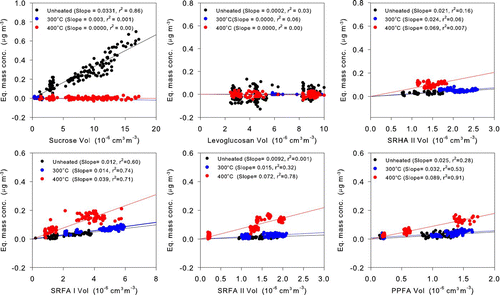
Values of b abs were measured simultaneously with M TOT at six different sites in Asia. The sampled BC particles were fresh and aged and emitted by combustion of fossil fuel and biomass, depending on the locations and seasons. At these locations, b abs was highly correlated (r 2= 0.85–0.93) with M TOT, with a 7% variability in the slope of the b abs–M TOT correlation, which is the mass absorption cross section, denoted as σabs(TOT) (CitationKondo et al. 2009). Even in air strongly impacted by burning of biomass (mainly rice straw) in Bangkok, σabs(TOT) did not deviate more than 10% from the average value of 5.9 m2 g–1. CitationKondo et al. (2009) derived σabs(TOT) = 10.4 m2 g–1 using B= 1.22 and E= 1.0. The set of parameters B= 1.397, E= 1.554, and σabs(TOT) = 5.9 m2 g–1 gives the same BC mass concentration for the same value of b 0. This is because only the ratio f fil/σabs affects M COSMOS, and the σabs was determined according to the change in f fil so that f fil/σabs is unchanged.
TABLE 5 Slopes of correlation plots of equivalent BC mass concentrations versus volume concentration measured by COSMOS I (heated inlet) and COSMOS II (without heated inlet)
3.4.2. Laboratory Experiments
Some fraction of the organic compounds collected on a quartz-fiber filter are known to be transformed into photo-absorbing carbon compounds at temperatures above 300°–400°C in oxygen-free and oxygen-rich environments, and this transformation causes an uncertainty in the value of M TOT (CitationNovakov and Corrigan 1995; CitationSchauer et al. 2003; CitationKirchstetter et al. 2004), depending on the detailed procedures during the heating. The errors in separating charred refractory organics from BC are considered to be the major cause of the uncertainties of TOT methods (CitationYu et al. 2002; CitationYang and Yu 2002; CitationHitzenberger et al. 2006; CitationChow et al. 2007; CitationBoparai et al. 2008; CitationHadley et al. 2008). If this process occurs in the present heating system for airborne particles, it may cause corresponding uncertainties in the BC values measured with the COSMOS instrument, although the mechanism of the interference is very different from that of TOT. We have evaluated this effect using the experimental setup shown in .
The experiments were carried out for the same organic species as discussed in section 2. For reference, PSL particles were also used. Aerosol particles generated by a nebulizer setup were dried using a diffusion dryer and then passed through a conductive elastic tube to a DMA for mobility-size selection. The flow of the nearly mono-modal organic particles was routed to a CPC, COSMOS I (with a heated inlet), and COSMOS II (without a heated inlet). The size of organic particles selected by the DMA ranged mainly from 100 to 200 nm, depending on the organic species.
The b 0(λ) measured by COSMOS I and COSMOS II were converted to equivalent BC mass concentrations in μg m–3 to quantify interpretation of the results. We used σabs= 5.9 m2 g–1 for the conversion. shows the correlations between equivalent BC mass concentrations and the volume concentrations of the generated organic aerosols (sucrose, levoglucosan, SRHA II, SRFA I, SRFA II, and PPFA) that were directed into the two COSMOS instruments. The volume concentrations (× 10–6 cm3 m–3) were estimated from the diameters and number concentrations of the organic aerosols. lists the slopes of correlation plots of equivalent M COSMOS versus volume concentrations.
Although COSMOS II showed significant response to sucrose, COSMOS I showed no detectable response. The COSMOS I slopes of low-MW organics, including sucrose, levoglucosan, glucose, adipic acid, and phthalic acid, did not exceed 0.0001 μg m–3/10–6 cm3 m–3 at either 300°C or 400°C, consistent with their high volatilities through the heated inlet. The HULIS showed significant absorption for both the unheated and heated inlet. The increased absorption with the inlet heated at 400°C suggests that some fraction of the HULIS pyrolyzed, leading to larger σabs. The reduction in particle size can also lead to enhanced absorption by COSMOS, as discussed in the previous section. However, this effect is considered to be small in this case, considering that the responses of SRHA II and SRFA to the COSMOS with the unheated inlet did not show significant differences for different mobility diameters between 100 and 200 nm.
For a quantitative analysis, we relate the response of the COSMOS to the volatility shown in the thermograms of the organic compounds. The OC4 fractions of pimelic acid and azelaic acid were as high as 25% and 58%, respectively (). However, the F OC5 values were as low as 6%. These organic species caused no detectable absorption in the COSMOS with the heated inlet. During Step-4, carbon is released by the evaporation of pyrolyzed carbon or by thermally dissociation of organic compounds. Some fraction of BC also evaporates when the Step-4 temperature is 870°C (CitationSubramanian et al. 2006).
These results indicate that only the carbon released during Step-5 is relevant to the absorption to COSMOS. This carbon was pyrolyzed mainly during Steps-3 and 4, as discussed in section 3.3. This relation is used to assess the interference of refractory organics on M COSMOS. First, we derive the sensitivities of the equivalent M COSMOS to the HULIS mass. The slopes of the equivalent M COSMOS–HULIS volume correlation at room temperature, 300°C, and 400°C are summarized in , together with the net increase in the slopes due to heating. The densities of SRFA and PPHA were measured to be about 1.5 g cm–3 (CitationDinar et al. 2006). We derived the ratios of the equivalent M COSMOS to the total HULIS mass (R 400 (total mass)) from the slopes of the equivalent M COSMOS–volume concentration and the HULIS densities. The slopes ranged from 0.039 (SRFA I) to 0.089 (PPFA) μg m–3/(10–6 cm3 m–3) at the heated inlet temperature of 400°C. The R 400 (total mass) values are calculated to be 0.025 (SRFA I)–0.059 (PPFA) μg/μg.
The mass fractions of carbon in these compounds were measured to be about 0.50 (CitationDinar et al. 2006). Based on this value, the ratios of the equivalent M COSMOS to the HULIS carbon mass (R 400(total-C)) are 2 ×R 400 (total mass) and are between 0.050 and 0.12 μg/μg. Namely, 5–12% of the carbon mass of these species was measured as equivalent M COSMOS, assuming that the absorption was entirely due to the charred HULIS.
At 300°C, the ratios (R 300(total-C)) were 2–4%, with a R 300 (total-C)/R 400 (total-C) ratio of about 1/3. The net increases in the absorption at 300°C were even lower (about 10 times) than those at 400°C. These results indicate that dominant fractions of the HULIS passed through the inlet without having been pyrolyzed at 400°C and that the fractions increased at 300°C.
It is useful to derive the [equivalent M COSMOS]/[OC5] ratios using F OC5. They are given as R 400 (OC5) =R 400 (total-C)/F OC5 and R 300 (OC5) =R 300 (total-C)/F OC5, and their values are summarized in . The average R 400 (OC5) and R 300 (OC5) values were 0.17 and 0.05.
TABLE 6 R 300 (OC5) and R 400 (OC5) of HULIS
TABLE 7 PC/BC ratios at different locations in Asia
The insensitivity of COSMOS to the low-MW species that produce high OC1-OC4 signals is due to their high volatilities in the heated inlet under high O2 concentration (). The R 400 (OC5) value as low as 0.17 is mainly due to the short residence time (<1 s) in the heated inlet. This is in contrast to the much longer time (>60 s) OC is exposed at 740°–870°C in He (Step-4) in the oven of the TOT instrument. Even at 300°C in the COSMOS inlet, more than 94% of the volumes of low-MW organic species evaporated (). However, only 10–30% of the volumes of the HULIS were lost through the heated inlet at this temperature, leading to a R 300(OC5)/R 400 (OC5) ratio of about 1/3.
3.4.3. Estimate of Interference of Refractory Organics on M COSMOS
It is possible to estimate the effect of charring of refractory organics in the heated inlet using R 300 (OC5) and R 400 (OC5). The use of this R 400 (OC5) includes an assumption that the σabs of charred refractory organics is the same as that of the HULIS used for the laboratory experiment. We obtained thermograms of the TOT instrument at the five locations in Asia, adopting the same temperature protocols as used for the laboratory experiments. The average PC/M TOT ratios were derived from the thermograms, as summarized in . We consider PC to be equivalent to OC5, as discussed in section 3.3. The average PC/M TOT ratios were as low as 0.04-0.08 in Tokyo, Jeju Island, and Bangkok during non-biomass burning periods, and they were as high as 0.23–0.28 in rural Guangzhou and Beijing and also in Bangkok during biomass burning periods. Simultaneous COSMOS/PSAP measurements were made with an inlet heated at 400°C. The estimated interference due to refractory organics is as low as 0.5–4.2%, as summarized in . If the temperature is lowered to 300°C, the interference will be less than 1.7%.
M COSMOS and M TOT were highly (r 2> 0.92) correlated, and the derived σabs(TOT) was stable to within 7% at these sites (CitationKondo et al. 2009). M TOT is directly influenced by the uncertainties in the estimate of PC, while only about 17% of PC contributes to the errors in M COSMOS. Therefore the high M COSMOS−M TOT correlation and little dependence of σabs(TOT) on the PC/M TOT ratios indicate the reliability of the PC estimate. This in turn indicates that σabs(TOT) represents BC mass concentrations disturbed little by refractory organics at these sites.
It has been reported that large amounts of organics are emitted from biomass burning (e.g., Mayol-Bracero et al. 2002). As an example, we use data obtained in Amazonia to assess the possible interference of organics on M COSMOS for this case (Mayol-Bracero et al. 2002), assuming that the properties of HULIS in the Amazonian rain forest are similar to those of SRFA and SRHA. The average concentrations of BC, OC, and water-soluble organic (WSOC) in the fine mode were 7, 35, and 23 μgC m–3 over the Amazon Basin in October 1999. Mayol-Bracero et al. (2002) roughly estimated that up to 50–60% of WSOC may be composed of HULIS. It is unlikely that other identified organic species produce high OC5 signals. The interference of the HULIS on M COSMOS with the inlet heated at 300°C and 400°C is crudely estimated to be about 0.3–0.4 μgC m–3 and 0.8–1.2 μgC m–3, respectively, assuming the same OC5/OC ratios as obtained by our laboratory experiments. This corresponds to errors in M COSMOS of about 4–6% and 11–17%, respectively.
4. COMPARISON OF BC MEASUREMENTS
4.1. RMM versus TOT
The observed M ref values were highly correlated (r 2= 0.88) with the M TOT values in the M TOT range between 0.5 and 7 μg m–3 in Tokyo. The obtained relation is expressed as
This high correlation and a linear regression slope near unity demonstrate that M TOT and M ref were nearly identical. It should be noted that the M ref and M TOT values were obtained every 1 h for 22 days under different meteorological conditions. M ref should be influenced by refractory organics more sensitively than M TOT, considering that significant fractions of the HULIS remained even at 400°C. The remaining mass concentrations of refractory organics was estimated from the average PC/M TOT= 0.047 (). The remaining mass relative to M TOT was calculated to be about 0.12 by using the average F OC5, mass fractions of carbon in HULIS (∼0.5), and the fractions of volumes lost by heating (). This suggests that M ref may have been overestimated by the same amount. If M ref in Equation (Equation5) is interpreted to represent the real BC mass concentrations, the factor of 0.96 becomes 1.08.
4.2. COSMOS versus SP2
4.2.1. Correlation between COSMOS and SP2 Measurements
Simultaneous measurements of BC were obtained with the SP2 and the COSMOS instrument for 9 days at RCAST between August 26 and September 12, 2009. A PM2.5-cyclone was used to exclude coarse particles. The weather was either mostly sunny or cloudy with little precipitation during the measurement period.
The sensitivity of the SP2 was found to be stable to within about 10% for particle mass measurement by means of calibration before and after the ambient measurements. The instrument was calibrated with fullerene soot. The lognormal size distribution was fitted to the mass size distributions of BC (dM SP2/dlogD BC). For comparison with the COSMOS measurements, we have integrated the mass size distribution dM SP2/dlogD BC to derive the total BC mass concentration over the entire BC size range (m SP2).
FIG. 8 Daily averaged number and mass size distributions of black carbon measured by SP2 in Tokyo in August and September 2009.
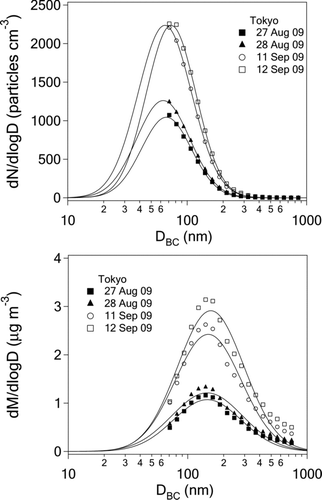
FIG. 9 Frequency distribution of the CMD and MMD of the lognormal size distributions fitted to 1-min averaged BC mass size distributions measured by SP2.
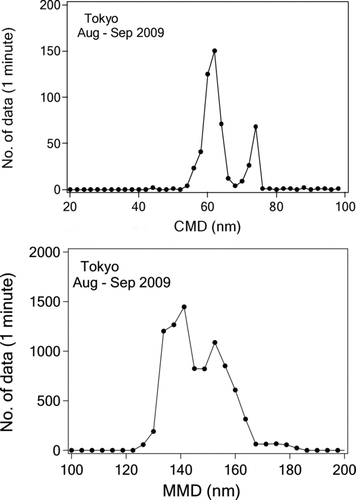
shows examples of the 1-day averaged number size distribution (dN/dlogD BC) and dM SP2/dlogD BC values measured between August 27 and September 11, 2009, along with lognormal fitted curves. In most cases, the count median diameter (CMD) was smaller than 70 nm. The BC mass for particles with diameters less than 70 nm was estimated assuming the lognormal mass size distributions (lower panel of ). On average, about 9–13% of the total BC mass concentration was in the size range below 70 nm for MMD = 130–185 nm.
shows the frequency distribution of the CMD and MMD for lognormal size distributions with a 1-minute resolution. CMD was derived stably only for a small fraction (about 10%) of the data. The mean CMD was 64 ± 6 nm, with a mean σgc of 1.66 ± 0.12. The majority of MMD were in the range of 130–170 nm, and the mean MMD value was 146 ± 12 nm, with a mean σgm of 1.82 ± 0.14.
shows the median shell/core ratios (D p/D BC) for different ranges of D BC. The median D p/D BC ratios were about 1.1, indicating that the BC particles were thinly coated. The possible effect of charring in extracting m*ref is considered to be small from these results, as discussed in section 2.2.
FIG. 10 Frequency distribution of the shell/core (D p/D BC) ratios of BC particles with different D BC size ranges.
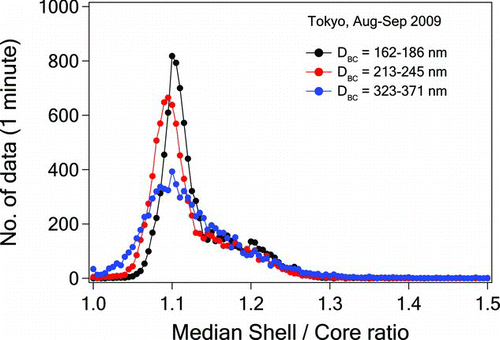
FIG. 11 Time series plots of BC mass concentrations measured by SP2 and COSMOS, their ratios, and MMD in Tokyo from September 10 to September 12, 2009. The mass concentration of BC was derived from the observed absorption coefficients by assuming a mass absorption cross section of 5.4 m2 g–1.
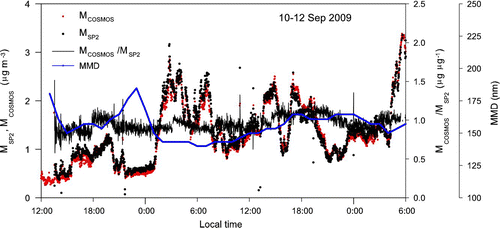
We estimated the error in M SP2 due to the uncertainty of the unmeasured BC is smaller than 5% by changing the parameters of the lognormal fits. We estimated the overall uncertainty of M SP2 due to the uncertainties of the calibration and the estimate of the unmeasured BC to be about 11%.
shows the time series of M COSMOS, M SP2, M COSMOS/M SP2 ratios, and MMD averaged for 1 min for the data obtained from September 10–12. The MMD ranged between 135 and 170 nm, and M COSMOS and M SP2 tracked each other well in this range.
Here we used σabs= 5.4 m2 g–1, which was obtained by the comparison of COSMOS and TOT measurements in Tokyo (CitationKondo et al. 2009) and updated using the new f fil.
shows the correlation between M COSMOS and M SP2 (1-min and 1-h averages) obtained during the observation period. The data were highly correlated (r 2= 0.96 and 0.97) at BC mass concentrations lower than 3–4 μg m–3. The high correlation indicates that interference from refractory organic aerosols to M COSMOS was small, considering that M SP2 is not influenced by the coexistence of organic aerosols.
FIG. 12 Correlation plots of BC mass concentrations measured by SP2 and those measured by COSMOS in 2009 using 1-min average data (left panel) and 1-h average data (right panel). Mass concentration of BC by COSMOS was derived from the absorption coefficients by assuming a mass absorption cross section of 5.4 m2 g–1. The least squares fitted line is denoted as the solid line.
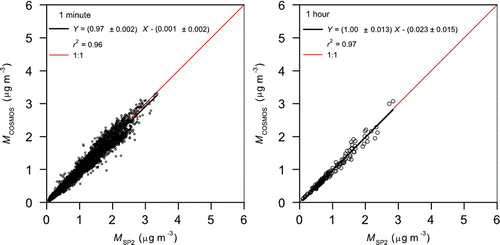
The average relationships of M COSMOS with M SP2 are expressed as follows.
We used bivariate regression analysis incorporating the errors in both variables. The high r 2 and small offset indicate that BC observed by SP2 (by incandescence) and COSMOS (by absorption) were similar. The value of σabs derived from the correlation between b abs and M SP2 was σabs(SP2) = 5.5 ± 0.6 m2 g–1. The σabs(TOT) = 5.4 ± 0.4 m2 g–1 agreed very well with σabs(SP2). This agreement means that M TOT agreed with M SP2 to the same degree on average. The small difference of the σabs(SP2) and σabs(TOT) is well within the uncertainties of the TOT, SP2, and COSMOS measurements.
It is expected that M COSMOS, M TOT, and M SP2 agree well in Asian regions free from strong influence of biomass burning, considering that σabs(TOT) was stable to within 10% in the 6 Asian locations. We also expect SP2 calibration with m*ref or fullerene soot to be valid in these regions.
4.2.2. Size Dependence of M COSMOS
We expect M COSMOS to have predicted and well-defined uncertainty despite its overall good agreement with M SP2. M COSMOS/M SP2 may vary with BC size distribution owing to the size dependence of σabs. For monodisperse BC, σabs depends on D BC for two reasons. First, the mass absorption cross section for airborne BC particles (σ*abs) depends on D BC. The value of σ*abs is reported to peak at a D BC of 150–200 nm (CitationBond and Bergstrom 2006) for typical refractive indices of BC particles. However, ambient BC particles are nonspherical (CitationPark et al. 2004), and reliable estimates of σ*abs for nonspherical BC particles are very limited. Second, the decrease in the frequency of multiple scattering with the increase in D BC decreases the photo-absorption by BC, resulting in a need of smaller correction for multiple scattering. The need for a smaller correction means that f fil given by Equation (Equation3) should decrease with increasing D BC. If the MMD of dm SP2/dlogD BC increased from a standard value, the f fil used for the present study would be overestimated, and the overestimation would lead to an underestimation of M COSMOS.
FIG. 13 M COSMOS/M SP2 ratio versus the MMD of BC. The solid black line is the least squares fitted line, and the red squares denote the mean M COSMOS/M SP2 ratios for 10-nm MMD bins.
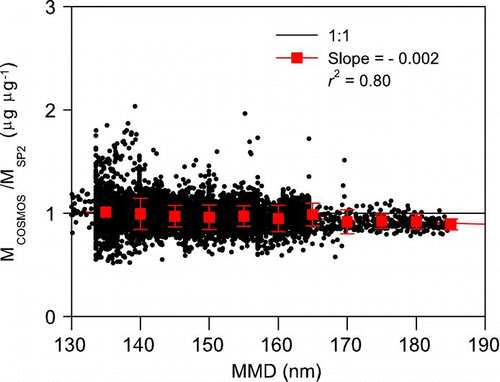
To investigate this size dependence, we plotted M COSMOS/M SP2 versus MMD (). The MMD was determined by the size distributions obtained by the SP2. The ratios decreased with MMD at an average slope of –0.0014/nm; on average, the ratios increased by about 7% as MMD increased from 130 to 180 nm. We estimate that f fil decreased by about 20% as MMD increased from 130 to 150 nm, on the basis of laboratory experiments and theoretical calculations (CitationKondo et al. 2009; CitationNakayama et al. 2010; CitationMoteki et al. 2010b). This decrease in MMD resulted in the underestimation of M COSMOS by the same amount. The estimate of the decrease in the M COSMOS/M SP2 ratio by CitationNakayama et al. (2010) includes the size dependence of σ*abs for typical refractive indices of BC based on the assumption of spherical particle shape. The fact that the MMD dependence predicted by the laboratory experiments was significantly larger than the observed dependence may be due to the difference in the size dependence of σ*abs assumed for the prediction.
5. DISCUSSION AND RECOMMENDATIONS
The refractory particles extracted by means of a heated inlet at 400°C provided a common reference for BC mass concentrations for all the instruments used in the present study. However, the responses of the different types of optical BC instruments may depend on the microphysical properties of BC (refractive index and shape). The peak LII intensity–m*ref relationship depends on these parameters (CitationMoteki and Kondo 2010). We used the m*ref of ambient BC particles that incandesce in urban air for this study. Considering the good agreement of the peak LII intensity–m*ref and peak LII intensity–m full relationships, either fullerene soot or thermally extracted BC should be used for calibration of an SP2. Thinly coated BC has to be chosen for thermal extraction to minimize the interference of refractory organics.
M COSMOS obtained with an inlet heated to 400°C correlated highly with M TOT and M SP2, with very good agreement between σabs (SP2) and σabs (TOT). The interference by charring of refractory organics on M COSMOS was estimated to be less than 5% based on laboratory experiments combined with thermograms of TOT. It was also shown that the degree of the interference is reduced by a factor of about 3 by lowering the inlet temperature down to 300°C without causing interference from low-MW organics. We recommend the use of COSMOS at 300°C if the same configuration and operational conditions of the heated inlet are adopted.
If the filter material (Pallflex E70-2075W, Pall, USA) used in a photo-absorption photometer with a heated inlet is the same as that used for a COSMOS and particle soot absorption photometer (PSAP; Radiance Research, WA, USA), the constants used for the present study can be used as is; that is, σabs= 5.5 m2 g–1 combined with Equations (Equation1)–(Equation4) can be used. If the filter materials are different, σabs can be determined by comparison of b abs with COSMOS measurements or with other standards, including a well-calibrated SP2 instrument.
As discussed in section 3.4. R 400 (total mass) was as low as 0.03–0.06. This ratio decreased by 1/3 at 300°C. By contrast, the HULIS mass concentration contributes to M ref more sensitively, and the sensitivity is larger at 300°C. It is likely that the good correlation between the M ref and M TOT values holds only at locations where the mass concentrations of refractory organics relative to M TOT are small, such as in Tokyo.
6. SUMMARY AND CONCLUSIONS
Coexistence of OA causes significant uncertainties in BC measurements, depending on the techniques used. In order to assess the effects of OA, we measured the volatilities of 13 organic species with different MW through a heated inlet. The low-MW organics were evaporated almost completely. Only about 10–30% and 20–60% of the volumes of the HULIS were lost at 300°C and 400°C, respectively.
For the most reliable BC mass concentration measurements, we used the SP2, which is free of interference from aerosols other than BC. For calibration of the SP2, we extracted particles that survived evaporation after passing through an inlet heated to 400°C. The refractory particles that incandesced (m*ref) were used to calibrate the LII peak intensity of the SP2. The uncertainty of m*ref is estimated to be about 10%. Fullerene soot showed microphysical properties (refractive index and shape) similar to those of ambient BC. The peak LII intensity was similar for the mass of fullerene soot (m full) that was the same as m*ref; this result is consistent with the similarity in the microphysical properties of fullerene soot and ambient BC. Practically, m full can be used as a primary standard. However, confidence in the absolute calibration of SP2 increases if both m full and m*ref are used and compared under various conditions, considering the limited measurements made thus far.
The HULIS underwent partial charring, causing additional absorption in the COSMOS. Only about 5–12% of the carbon atoms contained in HULIS contributed to the equivalent M COSMOS with an inlet heated at 400°C. This interference is reduced by about a factor of 3 at 300°C. The charred carbon mass was found to be directly related with the OC5 signal of the TOT. The [equivalent M COSMOS]/[OC5] ratios were about 0.17 and 0.05, respectively, for the inlet temperatures of 400°C and 300°C. The average errors due to charring of refractory organics on M COSMOS were estimated to be smaller than 5% in Asia. We recommend setting the inlet temperature at 300°C for COSMOS measurements.
The values of b abs were measured simultaneously with M SP2 for 9 days in Tokyo; the values were highly correlated (r 2= 0.97). The high correlation between M COSMOS and M SP2 is understood considering little influence of the coexistence of organic aerosols on these measurements. The derived σabs(SP2) agreed with σabs (TOT) to within 2%. This means that the masses of PM1 refractory aerosols that incandesce were very close to M TOT.
The value of σabs(SP2) varied by only 7% as MMD increased from 130 to 180 nm and changed little with aging. It should be noted that these results are restricted to Tokyo and similar aerosol mixtures that are not strongly impacted by refractory organic compounds such as those expected from biomass burning.
These results demonstrate that M SP2, M TOT, and M COSMOS are nearly identical to a first approximation in Asia in the absence of pronounced effects of forest fires and dust particles. Throughout these studies, the COSMOS measurements were stable and traceable to the calibrated SP2 measurements to within about 10%.
APPENDIX
A1. Method to Calculate Coating Thickness
The shape of the BC particles in the atmosphere is known to be fractal. Even if these BC particles are coated with volatile compounds, their structure is not concentric shell-core in a strict sense. Despite the difference in the detailed structures, coating thickness or shell/core ratio is a useful conceptual parameter to quantify the mixing state of BC. For the present study, we obtained the shell/core ratio by deriving the time-dependent scattering cross sections of BC particles from the scattering waveforms from an SP2 (CitationMoteki and Kondo 2008). We derived the scattering cross section of coated BC measured at the leading edge of the laser beam (before evaporation) and that measured at the onset of incandescence (after evaporation of coatings) using the light-scattering algorithm for concentric spheres (CitationAden and Kerker 1951; CitationBohren and Huffman 1983). The shell/core ratio derived by the new algorithm depends little on the assumed refractive indices. Further, the morphological dependence of light scattering and thermal emission has little affect on the shell/core ratios. This is because both the diameters of the core and shell are scaled by the light-scattering intensity measured by the same detection method. If the core diameter is estimated from the L-II peak signal, the uncertainty of the derived shell/core ratios will be larger.
A2. Dependence of the LII Intensity on the Microphysical Properties of Black Carbon
The time (t)-dependent intensity of the incandescence signal Si (t) measured by the SP2 is given as
C emit of an arbitrary body for radiation is equivalent to the photo-absorption cross section (CitationMoteki et al. 2009). The photo-absorption cross section of BC (C abs(D BC, λ)), where D BC is the mass equivalent diameter of BC, depends on N, the particle shape, and the mixing state (CitationMoteki and Kondo 2010; CitationShiraiwa et al. 2009). The size parameter x (= kD BC/2) is defined to categorize C abs(D BC, λ) at large and small values of D BC relative to λ. Here k= 2π/λ is the wavenumber. For x< ∼1, the necessary conditions for the Rayleigh–Gans approximation are generally satisfied, and C abs (D BC, λ) is given by
This expression indicates that C abs is proportional to volume V (or mass), Im[(N 2 –1)/(N 2+ 1)], and 1/λ. It does not depend on shape. For x> ∼1, generally the Rayleigh–Gans approximation does not hold, and C abs depends on particle shape. These size and shape dependencies are the cause of the observed nonlinearity in the correlation between the LII peak intensity and M SP2 at large D BC values (CitationMoteki and Kondo 2010).
Acknowledgments
The authors thank T. Ishigai for his support of the laboratory experiments. D. W. Fahey, J. P. Schwarz, and M. Kuwata provided very useful comments on the manuscript. This work was supported by the Ministry of Education, Culture, Sports, Science, and Technology (MEXT), the strategic international cooperative program of the Japan Science and Technology Agency (JST), and the global environment research fund of the Japanese Ministry of the Environment (B-083). This study was conducted as a part of the Mega-Cities: Asia Task under the framework of the International Global Atmospheric Chemistry (IGAC) project.
REFERENCES
- Aden , A. L. and Kerker , M. 1951 . Scattering of Electromagnetic Waves from Two Concentric Spheres . J. Appl. Phys. , 22 : 1242 – 1246 .
- Birch , M. E. and Cary , R. A. 1996 . Elemental Carbon-Based Method for Monitoring Occupational Exposures to Particulate Diesel Exhaust . Aerosol Sci. Technol. , 25 : 221 – 241 .
- Bohren , C. F. and Huffman , D. R. 1983 . “ Absorptio ” . 530 New York : and Scattering of Light by Small Particles. John Wiley & Sons .
- Bond , T. C. and Bergstrom , R. W. 2006 . Light Absorption by Carbonaceous Particles: An Investigative Review . Aerosol Sci. Technol. , 40 : 27 – 67 .
- Bond , T. C. , Anderson , T. L. and Campbell , D. 1999 . Calibration and Intercomparison of Filter-Based Measurements of Visible Light Absorption by Aerosols . Aerosol Sci. Technol. , 30 : 582 – 600 .
- Boparai , P. , Lee , J. and Bond , T. C. 2008 . Revisiting Thermal-Optical Analyses of Carbonaceous Aerosol Using a Physical Model . Aerosol Sci. Technol. , 40 : 930 – 948 .
- Cadle , S. H. and Groblicki , P. J. 1982 . “ An Evaluation of Methods for the Determination of Organic and Elemental Carbon in Particulate Samples ” . In Particulate Carbon-Atmospheric Life Cycle , Edited by: Wolf , G. T. and Klimisch , R. L. 79 – 85 . New York : Plenum Press .
- Cappa , C. D. , Lack , D. A. , Burkholder , J. B. and Ravishankara , A. R. 2008 . Bias in Filter-Based Aerosol Light Absorption Measurements Due to Organic Aerosol Loading: Evidence from Laboratory Measurements . Aerosol Sci. Technol. , 42 : 1022 – 1032 .
- Chow , J. C. , Yu , J. Z. , Watson , J. G. , Ho , S. S. H. , Bohannan , T. L. , Hays , M. and Fung , K. K. 2007 . The Application of Thermal Methods for Determining Chemical Composition of Carbonaceous Aerosols: A Review . J. Environ. Sci. Health Part A. , 42 : 1521 – 1541 .
- Dinar , E. , Mentel , T. F. and Rudich , Y. 2006 . The Density of Humic Acids and Humic Like Substances (HULIS) from Fresh and Aged Wood Burning and Pollution Aerosol Particles . Atmos. Chem. Phys , 6 : 5213 – 5224 .
- Gao , R. S. , Schwarz , J. P. , Kelly , K. K. , Fahey , D. W. , Watts , L. A. and Thompson , T. L. 2007 . A Novel Method for Estimating Light-Scattering Properties of Soot Aerosols Using a Modified Single-Particle Soot Photometer . Aerosol Sci. Technol. , 4 : 125 – 135 .
- Hadley , O. , Corrigan , C. E. and Kirchstettee , T. W. 2008 . Modified Thermal-Optical Analysis Using Spectral Absorption Selectivity to Distinguish Black Carbon from Pyrolized Organic Carbon . Environ. Sci. Technol. , 42 : 8459 – 8464 .
- Han , S. , Kondo , Y. , Takegawa , N. , Miyazaki , Y. , Oshima , N. , Hu , M. , Lin , P. , Deng , Z. , Zhao , Y. and Sugimoto , N. 2009 . Temporal Variation of Elemental Carbon in Beijing . J. Geophys. Res. , 114 ( D23202 ) doi:10.1029/2009JD012027
- Hitzenberger , R. , Petzold , A. , Bauer , H. , Ctyroky , P. , Pouresmaeil , P. , Laskus , L. and Puxbaum , H. 2006 . Intercomparison of Thermal and Optical Measurement Method for Elemental Carbon and Black Carbon at an Urban Location . Environ. Sci. Technol. , 40 : 6377 – 6383 .
- Huntzicker , J. J. , Johnson , R. L. , Shah , J. J. and Cary , R. A. 1982 . “ Analysis of Organic and Elemental Carbon in Ambient Aerosols by a Thermal-Optical Method ” . In Particulate Carbon-Atmospheric Life Cycle , Edited by: Wolf , G. T. and Klimisch , R. L. 79 – 85 . New York : Plenum Press .
- IPCC . 2007 . Climate Change 2007: The Physical Science Basis. Contribution of Working Group I to the 4th Assessment Report of the IPCC , Cambridge , , UK : Cambridge University Press .
- Kanaya , Y. , Komazaki , Y. , Pochanart , P. , Liu , Y. , Akimoto , H. , Gao , J. , Wang , T. and Wang , Z. 2008 . Mass Concentrations of Black Carbon Measured by Four Instruments in the Middle of Central East China in June 2006 . Atmos. Chem. Phys , 8 : 7637 – 7649 .
- Kirchstetter , T. W. , Novakov , T. and Hobbs , P. V. 2004 . Evidence That the Spectral Dependence of Light Absorption by Aerosols is Affected by Organic Carbon . J. Geophys. Res. , 109 ( D21208 ) doi:10.1029/2004JD004999
- Kondo , Y. , Komazaki , Y. , Miyazaki , Y. , Moteki , N. , Takegawa , N. , Kodama , D. , Deguchi , S. , Nogami , M. , Fukuda , M. , Miyakawa , T. , Morino , Y. , Koike , M. , Sakurai , H. and Ehara , K. 2006 . Temporal Variations of Elemental Carbon in Tokyo . J. Geophys. Res. , 111 ( D12205 ) doi:10.1029/2005JD006257
- Kondo , Y. , Miyazaki , Y. , Takegawa , N. , Miyakawa , T. , Weber , R. J. , Jimenez , J. L. , Zhang , Q. and Worsnop , D. R. 2007 . Oxygenated and Water-Soluble Organic Aerosols in Tokyo . J. Geophys. Res. , 112 ( D01203 ) doi:10.1029/2006JD007056
- Kondo , Y. , Sahu , L. , Kuwata , M. , Miyazaki , Y. , Takegawa , N. , Moteki , N. , Imaru , J. , Han , S. , Nakayama , T. , Hu , M. , Kim , Y. J. and Kita , K. 2009 . Stabilization of the Mass Absorption Cross Section of Black Carbon for Filter-Based Absorption Photometry by the Use of a Heated Inlet . Aerosol Sci. Technol. , 43 : 741 – 756 .
- Kondo , Y. , Takegawa , N. , Miyakawa , T. , Koike , M. , Miyazaki , Y. , Kanaya , Y. , Mochida , M. , Kuwata , M. , Morino , Y. and Shiraiwa , M. 2010 . Formation and Transport of Aerosols in Tokyo in Relation to Their Physical and Chemical Properties: A Review . J. Meteorol. Soc. Jpn. , 88 : 597 – 624 .
- Lack , D. A. , Cappa , C. D. , Covert , D. S. , Baynard , T. , Massoli , P. , Sierau , B. , Bates , T. S. , Quinn , P. K. , Lovejoy , E. R. and Ravishankara , A. R. 2008 . Bias in Filter-Based Aerosol Light Absorption Measurements Due to Organic Aerosol Loading: Evidence from Ambient Measurements . Aerosol Sci. Technol. , 42 : 1033 – 1041 .
- Lighty , J. S. , Veranth , J. M. and Sarofim , A. F. 2000 . Combustion Aerosols: Factors Governing Their Size and Composition and Implications to Human Health . J. Air Waste Manag. Assoc. , 50 : 1565 – 1618 .
- Lack , D. A. , Cappa , C. D. , Covert , D. S. , Baynard , T. , Massoli , P. , Sierau , B. , Bates , T. S. , Quinn , P. K. , Lovejoy , E. R. and Ravishankara , A. R. 2008 . Bias in Filter-Based Aerosol Light Absorption Measurements Due to Organic Aerosol Loading: Evidence from Ambient Measurements . Aerosol Sci. Technol. , 42 : 1033 – 1041 .
- Lim , H. J. and Turpin , B. J. 2002 . Origin of Primary and Secondary Organic Aerosol in Atlanta: Results of Time-Resolved Measurements During the Atlanta Supersite Experiment . Environ. Sci. Technol. , 36 : 4489 – 4496 .
- Mayol-Braccero , O. L. , Guyon , P. , Graham , B. , Roberts , G. , Andrea , M. O. , Decesari , S. , Facchini , M. C. , Fuzzi , S. and Artaxo , P. 2002 . Water-Soluble Organic Compounds in Biomass Burning Aerosols over Amazonia. Part 2: Apportionment of the Chemical Composition and Importance of the Polyacidic Fraction . J. Geophys. Res. , 107 ( D208091 ) doi:10.1029/2001JD000522
- McCreanor , J. , Cullinan , P. , Nieuwenhuijsen , M. J. , Stewart-Evans , J. , Milliarou , E. , Jarup , L. , Harrington , R. , Svartengren , M. , Han , I. -K. , Ohman-Strickland , O. , Chung , K. F. and Zhang , J. 2007 . Respiratory Effects of Exposure to Diesel Traffic in Persons with Asthma . N. Engl. J. Med. , 357 : 2348 – 2358 .
- Miyazaki , Y. , Kondo , Y. , Takegawa , N. , Komazaki , Y. , Fukuda , M. , Kawamura , K. , Mochida , M. , Okuzawa , K. and Weber , R. J. 2006 . Time-resolved Measurements of Water-Soluble Organic Carbon in Tokyo . J. Geophys. Res. , 111 ( D23206 ) doi:10.1029/2006JD007125
- Miyazaki , Y. , Kondo , Y. , Han , S. , Koike , M. , Kodama , D. , Komazaki , Y. , Tanimoto , H. and Matsueda , H. 2007 . Chemical Characteristics of Water-soluble Organic Carbon in the Asian Outflow . J. Geophys. Res. , 112 ( D22S30 ) doi:10.1029/2007JD009116
- Miyazaki , Y. , Kondo , Y. , Sahu , L. K. , Imaru , J. , Fukushima , N. and Kanno , A. 2008 . Performance of a Newly Designed Continuous Soot Monitoring System (COSMOS) . J. Environ. Monit. , 10 : 1195 – 1201 .
- Moteki , N. and Kondo , Y. 2007 . Effects of Mixing State on Black Carbon Measurement by Laser Induced Incandescence . Aerosol Sci. Technol. , 41 : 398 – 417 .
- Moteki , N. and Kondo , Y. 2008 . Method to Measure Time-Dependent Scattering Cross Sections of Particles Evaporating in a Laser Beam . J. Aerosol Sci. , 39 : 348 – 364 .
- Moteki , N. , Kondo , Y. , Takegawa , N. and Nakamura , S. 2009 . Directional Dependence of Thermal Emission from Nonspherical Carbon Particles . J. Aerosol Sci. , 40 : 790 – 801 .
- Moteki , N. and Kondo , Y. 2010 . Dependence of Laser Induced Incandescence on Physical Properties of Black Carbon Aerosols: Measurements and Theoretical Interpretation . Aerosol Sci. Technol. , 44 : 663 – 675 .
- Moteki , N. , Kondo , Y. and Nakamura , S. 2010a . Method to Measure Refractive Indices of Small Nonspherical Particles: Application to Black Carbon Particles . J. Aerosol Sci. , 41 : 513 – 521 .
- Moteki , N. , Kondo , Y. , Nakayama , T. , Kita , K. , Sahu , L. K. , Ishigai , T. , Kinase , T. and Matsumi , Y. 2010b . Radiative Transfer Modeling of Filter-Based Measurements of Light Absorption by Particles: Importance of Particle Size Dependent Penetration Depth . J. Aerosol Sci. , 41 : 401 – 412 .
- Novakov , T. and Corrigan , C. E. 1995 . Thermal Characterization of Biomass Smoke Particles . Mikrochim Acta. , 119 : 157 – 166 .
- Nakayama , T. , Kondo , Y. , Moteki , N. , Sahu , L. K. , Kinase , T. , Kita , K. and Matsumi , Y. 2010 . Size-Dependent Correction Factors for Absorption Measurements Using Filter-Based Photometers: PSAP and COSMOS . J. Aerosol Sci , 41 : 333 – 343 .
- Park , K. , Kittelson , D. B. and McMurry , P. H. 2004 . Structural Properties of Diesel Exhaust Particles Measured by Transmission Electron Microscopy (TEM): Relationships to Particle Mass and Mobility . Aerosol Sci. Technol. , 38 : 881 – 889 .
- Ramanathan , V. , Ramana , M. V. , Roberts , G. , Kim , D. , Corrigan , C. , Chung , C. and Winker , D. 2007 . Warming Trends in Asia Amplified by Brown Cloud Solar Absorption . Nature. , 448 : 575 – 579 .
- Schauer , J. J. , Maader , B. T. , Deminter , J. T. , Heidemann , G. , Bae , M. S. , Seinfeld , J. H. , Flagan , R. C. , Cary , R. A. , Smith , D. , Huebert , B. J. , Bertran , T. , Howell , S. , Kline , J. T. , Quinn , P. , Bates , T. , Turpin , B. , Lim , H. J. , Yu , J. Z. , Yang , H. and Keywood , M. D. 2003 . ACE-Asia Intercomparison of a Thermal-Optical Method for the Determination of Particle-Phase Organic and Elemental Carbon . Environ. Sci. Technol , 37 : 993 – 1001 .
- Schmid , H. , Laskus , L. , Abraham , H. J. , Baltensperger , U. , Lavanchy , V. , Bizjak , M. , Burba , P. , Cachier , H. , Crow , D. , Chow , J. , Gnauk , T. , Even , A. , Brink , H. M. T. , Giesen , K. -P. , Hitzenberger , R. , Huegelin , C. , Maenhaut , W. , Pio , C. , Carvalho , A. , Putaud , J. -P. , Toom-Sauntry , D. and Puxbaum , H. 2001 . Results of the “Carbon Conference” International Aerosol Carbon Round Robin Test Stage I . Atmos. Environ. , 35 : 2111 – 2121 .
- Schwarz , J. P. , Gao , R. S. , Fahey , D. W. , Thomson , D. S. , Watts , L. A. , Wilson , J. C. , Reeves , J. M. , Darbeheshti , M. , Baumgardner , D. G. , Kok , G. L. , Chung , S. H. , Schulz , M. , Hendricks , J. , Lauer , A. , Kärcher , B. , Slowil , J. G. , Rosenlof , K. H. , Thompson , T. L. , Langford , A. O. , Loewenstein , M. and Aikin , K. C. 2006 . Single-Particle Measurement of Mid Latitude Black Carbon and Light-Scattering Aerosols from the Boundary Layer to the Lower Stratosphere . J. Geophys. Res. , 111 ( D16207 ) doi:10.1029/2006JD007076
- Schwarz , J. P. , Gao , R. S. , Spackman , J. R. , Watts , L. A. , Thomson , D. S. , Fahey , D. W. , Ryerson , T. B. , Peischl , J. , Holloway , J. S. , Trainer , M. , Frost , G. J. , Baynard , T. , Lack , D. A. , de Gouw , J. A. , Warneke , C. and Del Negro , L. A. 2008 . Measurement of the Mixing State, Mass, and Optical Size of Individual Black Carbon Particles in Urban and Biomass Burning Emissions . Geophys. Res. Lett. , 35 ( L13810 ) doi:10.1029/2008GL033968
- Shiraiwa , M. , Kondo , Y. , Moteki , N. , Takegawa , N. , Miyazaki , Y. and Blake , D. R. 2007 . Evolution of Mixing State of Black Carbon in Polluted Air from Tokyo . Geophys. Res. Lett. , 34 ( L16803 ) doi:10.1029/2007GL029819
- Shiraiwa , M. , Kondo , Y. , Moteki , N. , Takegawa , N. , Sahu , L. K. , Takami , A. , Hatakeyama , S. , Yonemura , S. and Blake , D. R. 2008 . Radiative Impact of Mixing State of Black Carbon Aerosol in Asian Outflow . J. Geophys. Res. , 113 ( D24210 ) doi:1029/2008JD10546
- Shiraiwa , M. , Kondo , Y. , Iwamoto , T. and Kita , K. 2009 . Amplification of Light Absorption of Black Carbon by Organic Coating . Aerosol Sci. Technol. , 44 : 46 – 54 .
- Slowik , J. G. , Cross , E. , Han , J. -H. , Davidovits , P. , Onasch , T. B. , Jayne , J. T. , Williams , L. R. , Canagaranata , M. R. , Worsnop , D. R. , Chakrabarty , R. K. , Mossmüller , H. , Arnott , W. P. , Schwarz , J. P. , Gao , R. -S. , Fahey , D. W. , Kok , G. L. and Petzold , A. 2007 . An Inter-Comparison of Instruments Measuring Black Carbon Content of Soot Particles . Aerosol Sci. Technol. , 41 ( 3 ) : 295 – 314 .
- Subramanian , R. , Khlystov , A. Y. and Robinson , A. L. 2006 . Effect of Peak Inert-Mode Temperature on Elemental Carbon Measured Using Thermal-Optical Analysis . Aerosol Sci. Technol. , 40 : 763 – 780 .
- Subramanian , R. , Roden , C. A. , Boparai , P. and Bond , T. 2007 . Yellow Beads and Missing Particles: Trouble Ahead for Filter-Based Absorption Measurements . Aerosol Sci. Technol. , 41 : 630 – 637 .
- Takegawa , N. , Miyazaki , Y. , Kondo , Y. , Komazaki , Y. , Miyakawa , T. , Jimenez , J. L. , Jayne , J. T. , Worsnop , D. R. , Allan , J. D. and Weber , R. J. 2005 . Characterization of an Aerodyne Aerosol Mass Spectrometer (AMS): Intercomparison with Other Aerosol Instruments . Aerosol Sci. Technol , 39 : 760 – 770 .
- Yang , H. and Yu , J. Z. 2002 . Uncertainties in Charring Correction in the Analysis of Elemental and Organic Carbon in Atmospheric Particles by Thermal/Optical Methods . Environ. Sci. Technol. , 36 : 5199 – 5204 .
- Yu , J. Z. , Xu , J. and Yang , H. 2002 . Charring Characteristics of Atmospheric Organic Particulate Matter in Thermal Analysis . Environ. Sci. Technol. , 36 : 754 – 761 .