Abstract
A novel multifilter PM10–PM2.5 sampler (MFPPS) that enables the collection of four PM10 and four PM2.5 samples simultaneously has been developed and tested. The MFPPS uses a PM10 impactor as the inlet and operates at 33.4 L/min. After the inlet, the aerosol flow is divided half by a Y-type fitting. Half of the flow is directed into four PM10 filter cassettes, while the other half is directed into four PM2.5 filter cassettes after the aerosols are further classified by a PM2.5 impactor. An active flow control system consisting of two mass flow controllers (MFCs), one for PM10 and the other for PM2.5, is used to fix the total flow rate of 16.7 L/min for four PM10 or four PM2.5 channels based on the ambient pressure and temperature. To ensure flow rate uniformity through each of the four PM10 or four PM2.5 filter cassettes, an orifice is assembled behind each of the filter cassettes to increase the pressure drop, such that the flow rates of eight sampling lines are nearly equal using just two MFCs. The MFPPS was calibrated in the laboratory for particle collection efficiency curves first. Then, the ambient PM concentrations were compared with those of other two collocated FRM samplers, the dichotomous PM10 and the EPA WINS PM2.5 sampler in the field study. Calibration results showed the cutoff aerodynamic diameters of the PM10 and PM2.5 impactors were 9.8 ± 0.1 and 2.5 ± 0.05 μm, respectively. Field comparison results indicated PM10 and PM2.5 concentrations agreed well with the other two PM samplers.
INTRODUCTION
Many epidemiological studies pointed out that there were positive correlations between PM concentrations and increased human morbidity (Dockery et al. 1994; Anderson Citation2000; Kan et al. Citation2007). For better protection of public health, the U.S. Environmental Protect Agency (EPA) renewed the national ambient air quality standards (NAAQS) for particulate matter (PM) in 2006, in which the 24-h PM2.5 standard was tightened from the 1997 level of 65 to 35 μg/m3, and the annual PM2.5 standard was retained at 15 μg/m3. The 24-h PM10 standard was also retained at 150 μg/m3, while the annual PM10 standard was revoked due to a lack of evidence showing the association between the longterm exposure of coarse particle and health problems (U.S. EPA Citation2011).
For the determination of compliance with the NAAQS, the U.S. EPA has designed many FRMs for PM10 and PM2.5. A typical sampling system consists of a size-selective inlet, a denuder, a filter holder, a flow control system and a pump, in which an impactor or a cyclone is commonly used as the size-selective inlet. For impactors, solid particles may bounce from dry impaction surfaces readily. Lots of efforts have been made to resolve this problem, including oiled membrane substrates (Turner and Herring 1987), specially designed impaction surfaces (Tsai and Cheng Citation1995) and porous foam impaction substrates (Huang et al. Citation2005). Beside compliance monitoring, different components have been assembled to achieve specific research objectives (Watson and Chow Citation2001), including simultaneous sampling on multiple filters for different analyses, and multiple inlets in series or in parallel for sampling particles in different size fractions, etc. Examples are the Interagency Monitoring of Protected Visual Environments (IMPROVE) sampler, the Reference Ambient Aerosol Sampler (RAAS) (Watson and Chow Citation2001), and the Southern California Air Quality Study (SCAQS) sampler (AeroVironment Citation1987), etc.
Collocated samplers have been used to obtain multiple samples for different aerosol analyses. Monn and Becker (Citation1999) employed two collocated dichotomous samplers to collect PM2.5–10 and PM2.5 samples for the comparison of the ability of inhalable fine and coarse particle to cause cell injury and cytokine production in monocytes. Oanh et al. (Citation2009) used two collocated dichotomous samplers to collect PM ambient data for the receptor modeling of apportionment study. A Scanning Mobility Particle Sizer (SMPS, TSI, Model 3936), three dichotomous samplers (Andersen, Model SA-241), and three micro-orifice uniform deposit impactors (MOUDIs, MSP, Model 110) were collocated at a road side or in a highway tunnel in Taiwan to determine the PM mass and number concentrations simultaneously (Chen et al. Citation2010). Researchers have also used collocated samplers to study the differences in the performance of samplers. For example, Tsai and Perng (Citation1998) employed two collocated annular denuder systems to study the artifacts of ionic species in the FRM samplers. The effect of RH on the PM10 concentrations of the beta-gauge monitor was evaluated by two collocated high-volume PM10 FRM samplers in the ambient air (Chang et al. Citation2001). However, the use of collocated samplers increases the cost to purchase more samplers. Moreover, the measured concentrations among different samplers may not be comparable due to the differences in the shapes of collection efficiency curves and the cut-points of the sampling inlets.
The standard configuration of the IMPROVE sampler consists of one PM10 and one PM2.5 channel which contain Teflon filters for gravimetric or proton-induced X-ray emission (PIXE) elemental analysis, and two PM2.5 channels for ion and carbon analyses. The SCAQS sampler has up to a dozen sampling lines that allow two total particulate, seven PM2.5, and three PM10 collections simultaneously. The RAAS utilizes two AIHL PM2.5 cyclones (John and Reischl Citation1980) followed by a manifold to collect six PM2.5 samples through the attached filter holders. For these three samplers, a critical orifice is used to control the volumetric flow rate of each of the sampling lines. The critical orifice provides essentially constant volumetric flow rate independent of ambient pressure changes and only varies slightly with the square root of ambient temperature. However, the pressure downstream of the critical orifice must be less than 0.528 times the upstream pressure to achieve the critical state, which leads to the requirement of a large vacuum pump.
The active volumetric flow control system, which fixes the actual sampling flow rate based on the ambient pressure and temperature, has been introduced in many recent commercial samplers for accurate ambient sampling. Examples are the Partisol Model 2300 speciation sampler from Thermo, Inc. and the Spiral Aerosol Speciation Sampler (SASS) from Met One, Inc, which allow four and five simultaneous sample collections, respectively. Both of these samplers have an independent mass flow controller (MFC) to control the flow rate of each of the sampling lines. Totally, these systems require four and five MFCs, respectively. More MFCs will be needed if there are more sampling lines.
In this study, a multifilter PM10–PM2.5 sampler was developed for the simultaneous collection of four PM10 and four PM2.5 samples using only two MFCs, one for PM10 and the other for PM2.5 sampling lines, in the active flow control system. The reason of choosing four samples for each PM was due to the need of various analyses, such as gravimetric, organic carbon (OC), elemental carbon (EC), water-soluble ions, and trace elements analyses of collected samples, such as in the recent study of Chen et al. (Citation2010) who used collocated samplers to obtain different PM samples. In the laboratory, both monodispersed liquid and polydispersed solid particles were used to calibrate the PM10 and PM2.5 impactors. After the laboratory calibration, field validation was conducted by sampling ambient aerosol particles with the two other collocated manual FRM samplers, the dichotomous PM10 sampler (Andersen Model SA-241) and EPA WINS PM2.5 sampler (Thermo Model 2000-FRM).
EXPERIMENTAL METHODS
Description of the Multifilter PM10–PM2.5 Sampler (MFPPS)
shows the schematic of the present MFPPS. The sampler consists of: (1) an annular inlet with a PM10 impactor; (2) a smooth home-made Y-type fitting with four PM10 filter holders, one PM2.5 impactor and four PM2.5 filter holders; (3) an orifice assembled behind each of the filter holders to maintain the flow rate uniformity through all four PM10 and PM2.5 filter holders; (4) ambient temperature and pressure sensors; and (5) an active flow control system with two MFCs, a LabView program (National Instrument) and a PC. Efforts were made to reduce particle wall loss in the sampler by iterating the designs to ensure smooth flow streamline in it. For example, the half-angle of the PM10 impactor nozzle was changed from the original 30o to current 15o, the downstream impactor chambers were changed from the original cylindrical to the current cone shape, a common hole at the bottom of the impactor chambers was used for the Y-type fitting (branching tube inside diameter: 2.3 cm, angle: 60o), and a common inlet was used for the 4 branching holes in each of the flow dividers.
Aerosols are sampled into the MFPPS at 33.4 L/min via an annular slot inlet designed to have good inlet efficiency, which is the product of the transmission and aspiration efficiencies calculated based on the equations in Tsai et al. (Citation2008) and Witschger et al. (1997), respectively. The inlet efficiency is greater than 98.4 % for particles smaller than 10 μm in aerodynamic diameter (d pa) at the wind speed of 3 m/s. The inlet efficiency is reduced to 90.6 and 82.3% when d pa = 15 and 20 μm, respectively. After the inlet, a PM10 impactor is used to remove particles greater than 10 μm in d pa. Aerosol flow is then divided into two streams of equal flow rate of 16.7 L/min by a smooth home-made Y-type fitting, one stream is led to four PM10 filter cassettes behind a flow splitter, and the other is introduced into a PM2.5 impactor. After the PM2.5 impactor, the aerosol stream is divided by another flow splitter into four PM2.5 filter holders. An active flow control system consists of two MFCs, one for four PM10 channels and the other for four PM2.5 channels, was used to control the total actual sampling flow rate of both PM10 and PM2.5 channels at 16.7 L/min using the feedback signals of ambient pressure and temperature. The standard (or mass) flow rate of the MFC is adjusted automatically by the LabView program based on the following equation:
Since only one MFC was used to control the total flow rate through all four PM10 or PM2.5 filter cassettes, the flow rate through each of the filter cassettes could be different from other sampling lines due to the differences in the pressure drop of the filters. To overcome this nonuniform flow rate problem, an orifice with the diameter of 1.1 mm was installed after each of the filter holders to increase the pressure drop to a high enough value such that the flow rate differences in the sampling lines were less than 2%. In this study, the pressure drop increased by the orifice was about 40 cm H2O (or 3.92 kpa), which was about three times that of the Teflon filters but much lower than that of the critical orifices.
The inertial impactors were designed based on the impaction theory (Huang and Tsai Citation2001). When the flow Reynolds number based on the nozzle diameter is greater than 1500, the dimensionless is equal to 0.49, which
is defined as:
Laboratory Calibration
In this study, monodisperse oleic acid (OA) tagged with uranine dye wase used to determine the liquid particle collection efficiency of the PM10 and PM2.5 impactors and particle wall losses. The test procedure was described in Tsai and Cheng (Citation1995) and Tsai et al. (Citation2008). The liquid OA particles were generated by the vibrating orifice monodisperse aerosol generator (VOMAG, TSI Model 3450). As shown in , the aerosols were introduced into the sampler by a specially designed duct, which was based on the design of John and Wang (Citation1991) where the transition between the duct and the sampler inlet was smooth and had a constant cross-sectional area so that the average flow velocity was maintained at 3 m/s. The generated aerosols were neutralized by the TSI 3054 Kr-85 charge neutralizer before entering the mixing chamber. The monodispersity and steadiness of these aerosols were checked by the Aerodynamic Particle Sizer (APS, TSI Model 3321). Glass fiber filters were used to collect particles impacted on the impaction plate and the downstream of the impactor. At the end of each experiment period of 5–30 min, cotton swabs were used to recover the deposited particles at the inner wall of the sampling system. The glass fiber filters and cotton swabs were soaked in separate 0.001 N NaOH solution and ultrasonicated for 30 min, allowing the fluorescent material to dissolve in the solutions. The fluorescent concentrations of fluorescent in the glass fiber filters and cotton swabs were measured by the fluorometer (Turner Designs Model 10-AU-005, Cincinnati, OH, USA) separately. The particle wall loss, L wall, and the collection efficiency of the impactors, E imp, were determined as:
After the liquid particle tests, polydispersed solid KCl particles generated by the ultrasonic atomizing nozzle system (Model 8700, Sonotek Inc.) were used to test if particle bounce occurred from the substrates with different amounts of coated oil. The experimental setup is shown in . All generated particles were introduced into a mixing chamber to remove the excess moisture and further neutralized by the TSI 3054 Kr-85 charge neutralizer. The APS was used to measure the upstream and downstream aerosol distributions to determine the solid particle collection efficiency for the PM10 and PM2.5 impactors. For each experiment, the size distributions were measured for 3-min upstream, 3-min downstream, and then 3-min upstream again to ensure the steadiness of aerosol concentration. The KCl concentrations at the inlet of the impactors ranged from 37 to 568 #/cm3 for particles from 0.58 to 15 μm in d pa (maximum concentration was found at d pa = 3.3 μm). Each test was repeated at least 3 times. The solid particle collection efficiency η s (%) was determined as:
TABLE 1 Pressure drop and actual flow rate in each sampling channel, with and without an orifice (dia. = 1.1 mm) after the filter cassette.
Field Comparison Tests
In the field sampling study, the MFPPS and other two reference FRM samplers including dichotomous PM10 and EPA WINS PM2.5 sampler were collocated in the campus of National Chian Tung University to conduct the 24-h comparison tests. Twelve tests were taken from August 2009 to January 2010, during which the daily average temperature ranged from 10 to 33°C and the wind speed ranged from 2 to 5 m/s. A bug screen similar to that of dichotomous PM10 sampler was installed in the present sampler to protect any unwanted debris for the field test. Sampling inlets of these samplers were all fixed at the same height of 1.5 m. Teflon filters (Teflo R2PL037, Pall Corp., New York, USA) were used for all samplers, which were weighed before and after sampling after they were conditioned for at least 24 h in an environment conditioning room where the relative humidity and temperature were kept at 40 ± 2% and 21 ± 1°C, respectively. The electrostatic charge of the filters was eliminated by an ionizing air blower (Model CSD-0911, MELSEI, Japan) before the filters were weighed by a microbalance (Model CP2P-F, Sartorius, Germany). The precision of weighing was determined to be 2 μg by repeated weighing for at least five times.
RESULTS AND DISCUSSION
Flow Rate Uniformity
With the Teflon filters placed in the filter cassettes, the pressure drop and flow rate through the sampling lines were measured separately with and without the orifices in the filter cassettes. First, the pressure drop through each sampling line was measured individually with a magnehelic pressure gage (Dwyer Instrument, Michigan City, IN) at a fixed standard flow rate of 4.17 L/min controlled by the MFC. Then, the individual flow rate through each channel was measured with a bubble flow calibrator (Gilibrator-2, Sensidyne Inc., USA) upstream of the filter cassette at a total flow rate of 16.7 L/min through all four PM10 or PM2.5 sampling lines controlled by the downstream MFC. It was noted that a small pressure drop about 2 cm H2O was created by the flow calibrator which caused a lower measured flow rate in each individual sampling line than the set value of 4.17 L/min, since the flow rates through the other three lines without the upstream bubble flow calibrator were increased. The measured pressure drop and flow rate are summarized in . The data show that when the orifices are not used, the maximum relative difference in the pressure drop created by the Teflon filters is, 10.3%, which is large due to the differences in the filters. The flow rates between sampling lines are shown to be nonuniform with a maximum relatively difference of 9.1%. However, after the orifices are assembled behind the filter cassettes, the pressure drop in each sampling line is increased by nearly the same amount of about 40 cm H2O, which reduces the relatively differences in the pressure drop to less than 1.9%. Since the pressure drop differences are reduced, the flow rate uniformity between four sampling channels is achieved with a relatively difference of less than 1.7% using just one MFC through four PM10 or PM2.5 sampling filter cassettes.
Calibration Results
Major and other minor particle wall losses in the MFPPS are shown in and b, respectively. shows that the total particle wall loss, including minor losses, is less than 4.0 % for particle smaller than 5 μm in d pa. It gradually increases to 14% when d pa = 10 μm and 17% when d pa = 14 μm. Major particle loss occurred in the PM10 chamber and the Y-type fitting when d pa = 8 and 10 μm, respectively. However, the wall losses decreased for particle larger than 8 and 10 μm at the PM10 chamber and the Y-type fitting, respectively. This is because these two parts are downstream of the PM10 impactor, where most of the particles larger than 10 μm have been impacted already. For d pa larger than 10 μm, the major wall loss occurred in the annular slot inlet and the PM10 cone-shaped nozzle. As shown in , other minor losses were found at the PM10 and PM2.5 flow splitter and the PM2.5 impactor wall and chamber, and were less than 1.2 %. The wall losses were measured by using liquid OA particles. The losses are expected to be smaller for solid particles because of solid particle bounce (Marple et al. Citation1991).
and b show the calibrated collection efficiency of the PM10 and PM2.5 impactors by using liquid particles, respectively, with and without considering wall losses. The figures indicate that without considering wall losses, the cutoff aerodynamic diameters of the PM10 and PM2.5 impactor are 10.3 ± 0.05 and 2.5 ± 0.05 μm, respectively, which agree well with the theoretical cutpoints of 9.92 and 2.47, respectively, calculated by Equation (2). When the wall losses are included, the collection efficiency curve of the PM10 impactor shifts slightly to the left of that without considering the wall losses, and the d pa is decreased from 10.3 ± 0.05 to 9.8 ± 0.1 μm, which is only 2 % smaller than the designed value. For the PM2.5 impactor, however, there is no obvious difference between the collection efficiency curves with and without considering the wall losses, and the cutoff aerodynamic diameters are nearly the same. This is because the total wall loss is small for particles smaller than 5 μm, so that the effect of wall losses on the separation characteristic is not observed. The collection efficiency curves of the PM10 impactor (considering the wall losses) and PM2.5 impactor are close to those of the hi-vol PM10 sampler (McFarland et al. Citation1984), the dichotomous PM10 and EPA WINS PM2.5 sampler (Peters et al. Citation2001), respectively.
FIG. 5 Solid particle collection efficiency for the (a) PM10 impactor and (b) PM2.5 impactor with various amounts of coated oil on the impaction substrate.
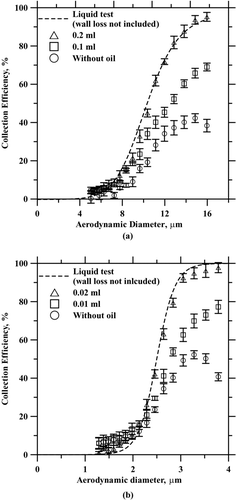
FIG. 6 Comparison of (a) PM10 concentrations and (b) PM2.5 concentrations between MFPPS, dichotomous PM10 and EPA WINS PM2.5 samplers.
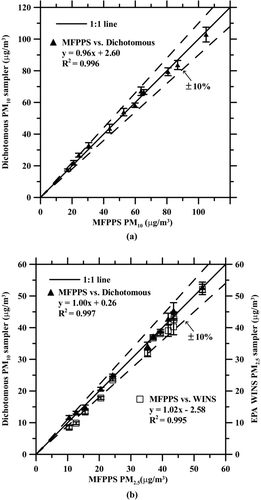
The above calibration results indicate that the traditional impactor theory can be used to design the multifilter PM10 sampling system successfully when particle wall losses are moderate in the PM10 sampling system. For the multifilter PM2.5 sampling system, since the wall losses in the system are small, the impactor theory can be used with good confidence.
and b show the solid particle collection efficiency of the PM10 and PM2.5 impactors, respectively, with various quantities of oil coated on the impaction substrates. Figures show that particle bounce occurs from the dry substrates, the maximum collection efficiencies are only 42% and 53% for PM10 and PM2.5 impactor, respectively. These results were also observed in previous studies (Rao and Whitby Citation1978; Cheng and Yeh Citation1979; Turner and Hering Citation1987; Tsai and Cheng Citation1995). Solid particle bounce still occurs when insufficient coated oil of 0.1 ml and 0.01 ml is used for the PM10 and PM2.5 impactor, respectively. The collection efficiency curves are less sharp due to the increased influence of surface roughness at these lower quantities of coated oil (Peters et al. Citation2001). When the coated oil is increased to 0.2 and 0.02 ml for the PM10 and PM2.5 impactors, respectively, solid particle bounce is seen to be eliminated and the collection efficiency curves become sharp. The corresponding cutoff aerodynamic diameters are 10.2 ± 0.2 and 2.5 ± 0.05 μm for PM10 and PM2.5 impactors, respectively, which is in good agreement with those of the liquid particles.
Field Comparison Tests
The comparison of PM10 and PM2.5 concentrations measured by the MFPPS with those of the other two collocated FRM samplers is shown in and b. The data points with error bars represent the average ± 1 standard deviation calculated from the concentrations of four sampling channels. , shows that the MFPPS PM10 concentrations agree well with those the dichotomous PM10 sampler with a good correlation coefficient (R 2) of 0.996. Similar results are illustrated in which shows that the PM2.5 mass concentrations obtained by the MFPPS agree well with those obtained by both of the dichotomous PM10 and EPA WINS PM2.5 samplers with R 2 of 0.997 and 0.995, respectively. Data points in these figures almost fall on the 1 to 1 line with the difference of less than 10 %. The ANOVA tests show no significant differences (p > .05) for the 12 samples taken for both PM10 and PM2.5 concentrations. Furthermore, with the orifices in the filter cassettes, the concentration differences between four sampling lines are found to be small with the relative standard deviation of less than 4 and 3% for PM10 and PM2.5 channels, respectively.
CONCLUSIONS
This study presents the development, calibration, and field validation of a novel MFPPS, which enables the collection of four PM10 and four PM2.5 samples simultaneously. The sampler operates at a total flow rate of 33.4 L/min by an active flow control system. A proper designed orifice was assembled behind each of the filter cassettes to reduce the differences in the pressure drop of the sampling lines, which makes the flow rate uniform at 4.17 L/min for each sampling line using just one MFC for four PM10 or PM2.5 sampling lines. The pressure drop increased by the orifices is much smaller than that of the critical orifices used in many multifilter samplers.
Both liquid particles and solid particles were used to calibrate the MFPPS. Results showed the cutoff aerodynamic diameters of the PM10 and PM2.5 impactors were very close to the designed values of 10 and 2.5 μm, respectively, with the consideration of particle wall losses. This study shows that the traditional impactor theory can be used to design the multifilter PM10 sampling system successfully when particle wall losses are moderate in the PM10 sampling system. For the multifilter PM2.5 sampling system, since the wall losses in the system are small, the impactor theory can be used with good confidence.
Similar collection efficiency curves for liquid and solid particles indicate that there was no solid particles bounce occurred on the impaction substrates coated with sufficient amount of oil. In the field comparison study, the MFPPS, dichotomos PM10 and EPA WINS PM2.5 sampler were collocated for 24-h ambient aerosol sampling. Test results showed that the PM10 and PM2.5 concentrations measured by all sampling channels of the MFPPS were uniform and agreed well with those measured by the other two FRM samplers.
In the future, different filters and sampling components can be assembled in different sampling lines for different chemical analyses, and for the study of sampling artifacts of OCs and ionic species in the PM10 and PM2.5 filter samples simultaneously.
Acknowledgments
The authors would like to express their gratitude to the Taiwan National Science Council for financial support under contract number NSC 95-2221-E-009-MY3 and NSC 99-2221-E-009-038-MY3.
REFERENCES
- AeroVironment . 1987 . Design and Testing of the SCAQS Sampler for the SCAQS Study. Report # AV-FR-86/649 , Monrovia, CA : AeroVironment .
- Anderson , H. R. 2000 . Differential Epidemiology of Ambient Aerosols . Phil. Trans. Royal Soc. London. Series A, Math. & Phys. Sci. , 1775 : 2771 – 2785 .
- Chang , C. T. , Tsai , C. J. , Lee , C. T. , Chang , S. Y. , Cheng , M. T. and Chein , H. M. 2001 . Differences in PM10 Concentrations Measured by β-gauge Monitor and Hi-Vol Sampler . Atmos. Environ. , 35 : 5741 – 5748 .
- Chen , S. C. , Tsai , C. J. , Huang , C. Y. , Chen , H. D. , Chen , S. J. Lin , C. C. 2010 . Chemical Mass Closure and Chemical Characteristic of Ambient Ultrafine Particles and Other PM Fractions . Aerosol Sci. Technol. , 44 : 713 – 723 .
- Cheng , Y. S. and Yeh , H. C. 1979 . Particle Bounce in Cascade Impactors . Environ. Sci. Technol. , 13 : 1392 – 1396 .
- Dockery , D. W. and Pope , C. A. 1994 . Acute Respiratory Effects of Particulate Air Pollution . Annu. Rev. Public Health. , 15 : 107 – 132 .
- Huang , C. H. , Chang , C. S. , Chang , S. H. , Tsai , C. J. , Shih , T. S. and Tang , D. T. 2005 . Use of Porous Form as the Substrate of an Impactor for Respirable Aerosol Sampling . J. Aerosol Sci. , 36 : 1373 – 1386 .
- Huang , C. H. and Tsai , C. J. 2001 . Effect of Gravity on Particle Collection Efficiency of Inertial Impactors . J. Aerosol Sci. , 32 : 375 – 387 .
- John , W. and Reischl , G. 1980 . A Cyclone for Size-selective Sampling of Ambient Air . JAPCA , 30 : 872 – 876 .
- John , W. and Wang , H. C. 1991 . Laboratory Testing Method for PM-10 Samplers: Lowered Effectiveness from Particle Loading . Aerosol Sci. Technol. , 14 : 93 – 101 .
- Kan , H. , London , S. J. , Chen , G. , Zhang , Y. , Song , G. Zhao , N. 2007 . Differentiating the Effects of Coarse and Fine Particles on Daily Mortality in Shanghai, China . Environ. Int. , 33 : 376 – 384 .
- Marple , V. A. , Rubow , K. L. and Behm , S. M. 1991 . A Microorifice Uniform Deposit Impactor (MOUDI): Description, Calibration and Use . Aerosol Sci. Technol. , 14 : 434 – 446 .
- McFarland , A. R. , Ortiz , C. A. and Bertch , R. W. 1984 . A 10μm Cutpoint Size Selective Inlet for Hi-Vol Sampler . JAPCA. , 34 : 544 – 547 .
- Monn , C. and Becker , S. 1999 . Cytotoxicity and Induction of Proinflammatory Cytokines From Human Monocytes Exposed to Fine (PM2.5) and Coarse Particles (PM10–2.5) in Outdoor and Indoor Air . Toxicol. Appl. Pharmacol. , 155 ( 3 ) : 245 – 252 .
- Oanh , N. T. K. , Pongkiatkul , P. , Upadhyay , N. and Hopke , P. P. 2009 . Designing Ambient Particulate Matter Monitoring Program for Source Apportionment Study by Receptor Modeling . Atmos. Environ. , 43 : 3334 – 3344 .
- Peters , T. M. , Vanderpool , R. W. and Wiener , R. W. 2001 . Design and Calibration of the EPA PM2.5 Well Impactor Ninety-Six (WINS) . Aerosol Sci. Technol. , 34 : 389 – 397 .
- Rao , A. K. and Whitby , K. T. 1978 . Non-ideal Collection Characteristics of Inertial Impactors—II. Cascade Impactors . J. Aerosol Sci. , 9 : 87 – 100 .
- Tsai , C. J. and Cheng , Y. H. 1995 . Solid Particle Collection Characteristics on Impaction Surfaces of Different Designs . Aerosol Sci. Technol. , 23 : 96 – 106 .
- Tsai , C. J. , Chang , C. S. , Chen , S. C. , Chen , P. , Shih , T. S. Pui , D. Y. H. 2008 . Laboratory and Field Tests of a Novel Three- Stage Personal Dust Sampler for Sampling Three Dust Fractions Simultaneously . Aerosol Sci. Technol. , 42 : 85 – 95 .
- Tsai , C. J. and Perng , S. N. 1998 . Artifacts of Ionic Species for hi-vol PM10 and PM10 Dichotomous Samplers . Atmos. Environ. , 32 : 1605 – 1613 .
- Turner , J. R. and Hering , S. V. 1987 . Greased and Oiled Substrates as Bounce-free Impaction Surfaces . J. Aerosol Sci. , 18 : 215 – 224 .
- U.S. EPA . 2011 . National Ambient Air Quality Standards (NAAQS) . http://www.epa.gov/air/criteria.html (accessed March 1, 2011)
- Watson , J. G. and Chow , J. C. 2001 . “ Ambient Air Sampling ” . In Aerosol Measurement: Principle, Techniques and Applications , 2nd ed. , Edited by: Brown , P. A. and Willeke , K. 821 – 824 . Hoboken, NJ : Wiley-Interscience .
- Witschger , O. , Fabries , J. F. and Görner , P. 1997 . Particle Entry Efficiency of an Annular Slot Aerosol Sampler . J. Aerosol Sci. , 28 : S679 – S680 .