Abstract
The development of unit-dose, inhalable, antibiotic microparticles for use in primary and combined therapy approaches to treating tuberculosis (TB), multi-drug-resistant (MDR-TB), and extensively drug-resistant TB is explored using the gentle drying process of Carbon-dioxide Assisted Nebulization with a Bubble Dryer (CAN-BD). The microparticles produced using this method contain capreomycin, kanamycin, and isoniazid, respectively, imbedded in L-leucine. Antibiotics were developed into inhalable antibiotic formulations for their utility in both first line and second line antibiotic treatment regimens. Capreomycin and kanamycin are typically administered by injection making them desirable candidates for the development of a needle-free delivery system that addresses the Grand Challenges in Global Health Initiative #3. In response to this challenge, unit-dose packaging that preserves powder properties by protecting them from moisture, oxidants, and UV exposure, and a low cost “active” dry powder inhaler, the PuffHaler, were developed and used as a prototype device, in addition to the Aerolizer, to disperse the microparticle antibiotic formulations. Antibiotic formulations show yields above 50% in small-scale powder production by CAN-BD. Capreomycin and kanamycin show improved powder yields in scale up experiments. The particle properties were characterized using scanning electron microscopy, Karl Fischer moisture analysis, Anderson Cascade Impaction studies, and X-ray diffraction. The inhalable antibiotic formulations are within a respirable size range (1–5 μm), and have less than 3% residual moisture. Unit-dose dry powder antibiotics have the potential to provide easy-to-use, stable products with improved safety profiles.
INTRODUCTION
TB treatment has many challenges, which include antibiotic product contamination, difficulties in reaching target populations, wastage, the potential for needle-stick injuries, difficulties associated with sharps disposal, and the challenge of reaching high bacterial burden areas of the lung that are cutoff from a blood supply by the architecture of granulomas (Horsburgh et al. Citation2000; Mitragotri Citation2005; Shakoor et al. Citation1997; Shin et al. Citation2004; Taylor et al. Citation1995; Varmus et al. Citation2003). Many of these challenges may be overcome with the use of a pulmonary delivery strategy that incorporates unit-dose packaging. CAN-BD was chosen as the method for producing inhalable antibiotic microparticles because of its track record of success in producing a variety of dry, active powders of vaccines, and small molecule pharmaceuticals in a size range suitable for pulmonary delivery including Edmonston-Zagreb (EZ) measles vaccine, hepatitis B vaccine, and polyclonal IgG (Cape et al. Citation2008). CAN-BD processed microparticles have powder properties (size, moisture content, stability, and potency) that were developed specifically for pulmonary delivery. Preliminary explorations of making antibiotic dry powders using CAN-BD have been conducted (Cape et al. Citation2008; Sievers et al. Citation2006; Sievers et al. Citation2007). This work builds on those earlier results, with improved inhalable particle characteristics obtained by further exploration of formulation and processing conditions as well as the use of a new DPI technology including a specific reservoir material improvement.
CAN-BD is a unique blend of supercritical/near-critical microparticle forming techniques and spray drying. The CAN-BD process for creating microparticle vaccines and biopharmaceuticals is advantageous because the dense gas is not used to precipitate the solute of interest, but rather it is used to nebulize the liquid antibiotic solution into small microdroplets. Unlike many supercritical processes that require the solvent and supercritical or near-critical fluid to be miscible (Jovanovic et al. Citation2004), CAN-BD is not limited by this requirement, as organic solvents are not necessary to increase the solubility of the dense gas in the antibiotic or biopharmaceutical solution of interest (Cape et al. Citation2008; Sievers et al. Citation2007). The solute can be dissolved in a biologically stabilizing solution such as water with buffers, stabilizers, and excipients. Due to the relatively low processing temperatures used in CAN-BD, less decomposition of thermally labile drugs is expected.
The needle-free pulmonary delivery strategy was developed in response to the Grand Challenges in Global Health Initiative #3 (Varmus et al. Citation2003). This initiative was designed to create new technologies for the administration of vaccines; however, this work expands the application of needle-free pulmonary devices to inhalable antibiotics and TB treatment. Traditional syringe and needle administration is associated with increased risk of infections due to human immunodeficiency virus (HIV), hepatitis B (HBV), and C (HCV) virus resulting from needle-stick injuries, inappropriate re-use of needles or syringes, and the challenges of proper needle disposal (Giudice and Campbell Citation2006; Mitragotri Citation2005). Needle-free delivery methods have the potential to improve drug delivery safety, while unit-dose packaging may reduce antibiotic wastage, and microbial contamination. The potential therapeutic advantages of inhalable TB treatment are based on the success of aerosol antibiotic drug delivery in cystic fibrosis patients, the pulmonary route of infection of Mycobacterium tuberculosis (Mtb), and the large population of HIV and TB coinfected individuals (Borsje et al. Citation2000; Pandey and Khuller Citation2005; World Health Organization Citation2011).
The current best-case scenario for drug susceptible TB is a 6 to 9 month first-line drug regimen. As first-line drugs are delivered orally, therapeutic drug concentrations can only be reached in parts of the body with adequate blood circulation. Bacteria harbored in granulomas often lack a strong blood supply due to loss of vascularization. Thus, oral drugs may not penetrate the granulomas, resulting in subtherapeutic doses of antibiotics to this subpopulation of Mtb (Muttil et al. Citation2009). By depositing antibiotics in the deep lung, one may be able to reach TB lesions that lack a strong blood supply, and may also increase the targeted dose to the lung airspace and tissue while reducing systemic side effects and, potentially, the length of treatment. Using inhalable antibiotics in combination with more traditional treatment may provide a two-pronged attack strategy, from inhalation as well as the blood side, for MDR-TB. Failure to sterilize all bacterial populations often results in the development of resistant strains (Nuermberger et al. Citation2004; Tyagi et al. Citation2005; Wolf et al. Citation2007). Increasing the targeted dose in the lung airspace and targeting bacteria in protective microenvironments such as those in granulomas may prevent the development of MDR-TB by ensuring that threshold doses of antibiotics reach bacteria populations that are difficult to target by traditional methods through only the blood (parenteral or oral delivery). New drugs or new methods for delivering existing therapies that shorten the duration of current treatment would greatly improve compliance and thus help prevent the emergence of resistant Mtb.
To illustrate the broad applicability of creating inhalable antibiotics via CAN-BD we chose to investigate a first-line antibiotic, isoniazid (INH), and two second-line antibiotics, capreomycin sulfate and kanamycin sulfate. The antibiotic microparticles are targeted for delivery to the deep lung by optimizing the formulations and particle processing conditions to produce particles with a large fine particle fraction (FPF) below 3.3 μm, the ideal aerodynamic size for reaching the alveolar space and poorly vascularized lesions and granulomas that harbor treatment-elusive bacilli in protective microenvironments. As these protected bacilli can transition through different metabolic states INH is particularly valuable as an inhalable antibiotic because it is active against metabolically dynamic and semidormant bacteria (Muttil et al. Citation2009). Furthermore, at therapeutic levels INH, a small molecule prodrug, is bactericidal against both intracellular and extracellular Mtb organisms (Chanwong et al. Citation2007). While INH is available orally it is an essential component of first line antibiotic treatment courses for TB and the oral delivery method may result in subtherapeutic antibiotic delivery to granulomas with a dwindling blood supply (Girling Citation1989; Hiyama et al. Citation2000). Inhaled INH may reduce treatment times, thereby improving compliance and reducing the development of resistant strains, by providing higher levels of antibiotics to isolated bacterial populations.
Both capreomycin sulfate and kanamycin sulfate are considerably larger molecules that are often used in injection form due to poor absorption through the gastrointestinal tract (Helms et al. Citation2006). Due to the common injection route of these second-line drugs, inhalable antibiotic options would likely improve safety and eliminate complications associated with disposal of needles, while maintaining absorption.
MATERIALS AND METHODS
The CAN-BD process and equipment schematic is shown in . CAN-BD has been described previously in more detail (Cape et al. Citation2008). In this process, the antibiotic is dissolved in water. Both the antibiotic solution and the nebulization fluid are pumped through 1/16 in outer diameter (OD) stainless steel tubing into the inlets of a tee (Alltech 0.25 mm bore stainless steel tee (p/n 30771)) at 83 bars. A pair of either Isco screw piston pumps for the liquid CO2 or conventional HPLC pumps for the drug solution were used. Once in the tee, the solution or suspension and the liquid, near critical, CO2 are mixed intimately. The mixture of dense gas and drug solution flows from the tee through a 75 μm inner diameter, 10 cm-long, fused silica restrictor (Polymicro TSP075375) and forms a wet aerosol plume upon the rapid expansion of the compressed CO2 into a glass drying chamber maintained at or near atmospheric pressure. Particles are formed when the decompressed plume containing microdroplets and hypothesized microbubbles is rapidly dried by warm nitrogen (60°C) flowing through the glass-drying chamber at 30 L/min (Sievers et al. Citation2001). Particles are collected downstream of the drying chamber with a single 0.45 μm dead-end filter to achieve collection of smaller amounts of powder. This method was employed for the small powder runs used to produce inhalable antibiotic formulations for all of the PuffHaler and Aerolizer results described in this article.
Alternatively, a double filter apparatus that has two 0.45 μm filters on either side of a glass chamber can be used for larger batches of powder. The top filter is back-pulsed at regularly timed intervals to release powder onto the bottom filter, where the powder, optionally, undergoes brief additional drying with warm nitrogen flowing at 30 mL/min through the bottom filter at the end of the primary processing. This alternative method was used in the scale up procedure for creating larger amounts of kanamycin and capreomycin powders.
Capreomycin sulfate, kanamycin sulfate, and isoniazid were chosen for development into inhalable antibiotics due to structural diversity, significance in first and second line anti-TB drug regimens, and, in the case of kanamycin and capreomycin, the needle-based delivery method. The structural diversity of the antibiotics is evident from their molecular weights, which are 752.76 g/mol, 582.58 g/mol, and 137.14 g/mol for capreomycin, kanamycin, and isoniazid, respectively. All three antibiotics and L-leucine were purchased from Sigma-Aldrich, St Louis, MO. The formulations studied in this article: capreomycin (5% total dissolved solids consisting of 80% capreomycin sulfate and 20% L-leucine), kanamycin (5.69% total dissolved solids consisting of 80% kanamycin sulfate and 20% L-leucine), and isoniazid (5% total dissolved solids consisting of 80% isoniazid and 20% L-leucine). The increase to 5.69% total dissolved solids for the kanamycin formulation was due to the higher solubility of kanamycin.
Particle shape, geometry, and surface morphology are examined using a Scanning Electron Microscope. Scanning electron microscopy (SEM) was performed on TB drug powders with a JEOL model #JSM-7401 (FESEM) operating at either 3 or 5 kV with a filament current of about 0.5 mA (The images within this article have the operating voltage printed on the image). Powder samples were deposited on carbon conductive double-sided tape, and then most of each sample was tapped off to leave a thin layer of microparticles. Samples were coated with a gold layer using a sputter coater operated for 30 s at a sputtering current of 40 mA.
The moisture content of the powders was determined by extraction of the water into methanol and measuring the concentration by the Karl Fischer coulometric titration method (Denver Instruments, Model 260, and 275KF). The protocol in USP Chapter <921> was followed and tests were conducted at humidity below 15% RH.
Crystallinity of the powders was assessed using powder X-ray diffraction (pXRD) scanning from 5° to 45° at a wavelength of 1.54 Angstroms on a Scintag PAD-5 X-ray diffractometer in continuous-scan mode. The step size was 0.02 and the scan rate was 2° per minute. The Diffraction Management System for NT (DMSNT) was used to search and match peaks from the isoniazid formulation diffractogram to the isoniazid standard in the International Centre for Diffraction Data (ICDD).
To provide an effective alternative to traditional syringe-based vaccines and biopharmaceuticals, one option is to develop inhalable vaccine and antibiotic powders with fine particle fractions suitable for pulmonary delivery, having low moisture, high stability, and high potency. Pulmonary delivery of biopharmaceuticals requires specific fine particle fractions and high dispersibility. The aerodynamic particle requirements for pulmonary delivery are particles in the 1 to 5 μm range, preferably with a 1 to 3 μm range for delivering particles to the alveolar or deep lung region (Crowder et al. Citation2002). Aerodynamic particle size distribution, particle shape, and powder dispersion characteristics dictate the performance of inhalation devices (Bosquillon et al. Citation2001; Crowder et al. Citation2002). To meet regulatory requirements, measurements of aerodynamic particle size distribution and deposition are usually done using an Andersen Cascade Impactor (ACI) and a Dosage Unit Sampling Apparatus (DUSA) (USP Chapter <601>). ACI experimental results provide fine particle fractions (FPF). Particles with aerodynamic diameters equal to or larger than the specified cutoff diameter of a given ACI stage impact and collect upon the plate for that stage. Therefore, the FPF is the fraction of particle mass contained in particles having aerodynamic diameters smaller than the specified cutoff diameter of a particular ACI stage. In this work, the fractions are defined in terms of the total powder mass loaded into the PuffHaler or Aerolizer. The FPF measured is an indication of where the powder is likely to deposit in the lung upon inhalation, and is affected by dispersion of the powder. Results from a DUSA provide emitted dose (ED); ED is the mass percentage of particles leaving the dry powder inhaler (DPI) system and available for inhalation.
Fine particle fractions were determined using a modification of the USP Chapter <601>, in which an abbreviated set-up of the Andersen Cascade Impactor (Westech, Marietta, GA) was employed (Mitchell et al. Citation2010). The abbreviated setup of the ACI at 28.3 L/min consisted of stages 0, 1, 3, and F, with cutoffs of 9 μm, 5.8 μm, and 3.3 μm, respectively. The abbreviated setup of the ACI at 60 L/min consisted of stages 0, 1, 2, and F, with cutoffs of 6.5 μm, 4.4 μm, and 3.3 μm, respectively. Emitted doses were determined according to USP Chapter <601> using a DUSA. Sample preparation and tests were conducted in a glove box purged with dry nitrogen gas to maintain relative humidity below 15%. Ten-milligram aliquots of antibiotic formulations were dispensed volumetrically by the Omnidose TT (Harro Hoefliger GmbH, Almersbach, Germany) into aluminum-foil polymeric laminate blisters and thermally sealed. For testing with the Aerolizer (manufactured by Novartis Pharma AG for Schering Corp, Kenilworth, NJ), gelatin capsules were loaded with antibiotic powder. The mass of the powder in blisters or capsules was then gravimetrically verified. Blisters remained sealed until peeling back the lid immediately before use. The powders were then dispersed into the reservoir before being sampled through a mask by the ACI or DUSA. In these procedures, the powder aerosol in the reservoir is drawn into the ACI or DUSA at 28.3 L/min or 60 L/min. In the ACI procedure, particles are separated by aerodynamic diameter on sequential stages and gravimetrically analyzed to quantitate the FPF (Rebits et al. Citation2007). Capreomycin, isoniazid, and kanamycin were processed at the University of Colorado, Boulder, and packaged into foil laminate blister packs at Aktiv-Dry LLC. The antibiotic microparticles were analyzed at Aktiv-Dry LLC for FPF and ED.
The ED was gravimetrically determined by analyzing a single filter in the DUSA. When particles are dispersed from the foil laminate blister pack in the inhaler, a portion of the particles flows with the gas stream, while some remain adhered to the walls of the blister pack and on the walls of the other inhaler parts. The DUSA filter captures only what is actually emitted from the reservoir.
Lastly, the dry powder dispersion and emitted dose characteristics have been studied by administration of antibiotic powder using low cost dry powder inhalers (e.g., the PuffHaler, and the Aerolizer). The new PuffHaler, described in , is an active DPI, with a disposable mask suitable for use with infants or test animal subjects such as cotton rats (Kisich et al. Citation2011), and rhesus macaques (Lin et al. Citation2011).
The Aerolizer is a well-established, commercial passive DPI, which requires the subject to inhale on command to generate powder dispersion (Hindle and Byron Citation1995; Stelmach et al. Citation2009; Traini et al. Citation2010; Vianna et al. Citation2009). Both the Aerolizer and the PuffHaler provide alternatives, for different age groups, to traditional subcutaneous injections or oral administration. The results from these two test devices illustrate the importance of the inhalation device in aerosolization and delivery efficacy.
PuffHaler reservoirs were manufactured from POLYBATCH VLA 55 UK antistatic polyethylene film bags purchased from Uline (Pleasant Prairie, WI). These antistatic bags were formed into PuffHaler reservoirs with the desired dimensions and volume by following a folding and cutting protocol and using a heat sealer to introduce new permanent seams to hold the desired reservoir configuration.
PuffHaler reservoir materials were tested using placebo powder, which contains many of the excipients used in a variety of the inhalable powders developed by our group. Specifically, the placebo consists of 50 g/L of myo-inositol (Sigma-Aldrich, St Louis, MO), in addition to six other constituents: 25 g/L of hydrolyzed gelatin (E-Merck, Germany), 16 g/L of L-arginine-HCl (Sigma-Aldrich, St Louis, MO), 1.0 g/L of L-alanine (Sigma-Aldrich, St Louis, MO), 2.1 g/L of histidine (Sigma-Aldrich, St Louis, MO), 3.5 g/L of TC lactalbumin hydrolysate (Becton Dickinson, NJ), 3.0 g/L of tricine buffer (Sigma-Aldrich, St Louis, MO). The placebo powder was made, as described earlier, using the alternative CAN-BD procedure with a double filter apparatus.
RESULTS AND DISCUSSION
To help combat TB, a disease that kills 1.3 million people a year, we are developing unit-dose, inhalable, antibiotic microparticles for use as a primary and combined therapy approach to treating TB and multiple drug resistant TB (MDR-TB) (Namgyal Citation2006). Lack of patient compliance during lengthy treatment regimens is a major contributing factor in the development of MDR-TB (Horsburgh et al. Citation2000; Shin et al. Citation2004). In developing countries, because inhalable dry powders can be administered by health care workers with minimal training and weigh less than combined antibiotics and water-for-injections, they are transport friendly and may reach more remote patients, making compliance more probable. Moreover, as CAN-BD antibiotic powders are also needle-free, inhaled use improves safety for both administrators and patients by eliminating needle-sticks and blood-borne infections from needle re-use. These antibiotic powders have fine particle fractions from the PuffHaler as great as 21.6% less than 3.3 μm aerodynamic diameter, which are ideal for alveolar deposition.
The antibiotics capreomycin, kanamycin, and isoniazid were formulated with 5%, 5.69%, and 5% total dissolved solids, respectively. The 5%–5.69% TDS consisted of 80% antibiotic with 20% L-leucine. The inhalable antibiotic powders were all made on a small batch CAN-BD system and had yields of 53.7% for the capreomycin formulation, 70.4% for the kanamycin formulation and 51.2% for the isoniazid formulation. Both capreomycin and kanamycin antibiotic formulations have been made as large batch powders and show yields of 87.8% and 97.0%, respectively. The large batch yield data are based on single scale up experiments.
Minimal inhibitory concentrations (MIC) in liquid medium using standardized Bactec-460 technology have previously been used to establish retention of antibiotic activity post CAN-BD processing (Heifets Citation1988; Heifets et al. Citation1991). To date CAN-BD processed formulations of capreomycin, amikacin, ciprofloxacin-HCl, rifampin, and moxifloxacin-HCl resulted in respirable antibiotics with 100% retention of activity, within error, against M. tuberculosis. Specifically, amikacin, capreomycin, ciprofloxacin, moxifloxacin, and rifampin retained, respectively, 104% +/− 7%, 95% +/− 7%, 93% +/− 20%, 81% +/− 43%, and 109% +/−34% activity (Sievers et al. Citation2006). Kanamycin and isoniazid are expected to show a similarly high retention of activity post CAN-BD processing.
The shape and morphology of capreomycin, isoniazid, and kanamycin were investigated using SEM (). As the capreomycin and kanamycin particles get larger, they tend to have a crumpled morphology. The smaller particles are more spherical. The SEM of isoniazid shows textured spheres, crystals, and some cube-like particles in a range of sizes. However, the amorphous textured spheres are the dominant particle morphology.
FIG. 3 FESEM images of (a) kanamycin (b) capreomycin (c) isoniazid. The antibiotics were formulated with 5% to 5.63% total dissolved solids and consisted of 80% antibiotic with 20% L-leucine.
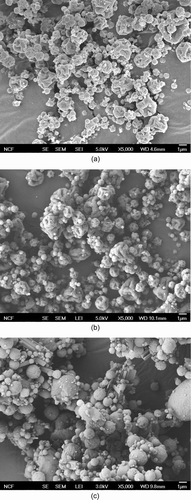
FIG. 4 The top diffractogram is of the isoniazid formulation, which was made from the CAN-BD processing of a 5% TDS solution containing 80% isoniazid and 20% L-leucine. The box below the isoniazid formulation is the DMSNT identified peaks from the isoniazid formulation. The bottom box is the diffractogram of the matching peaks of isonicotinic acid hydrazide from ICDD.
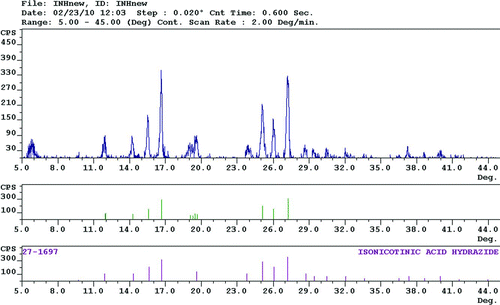
The inhalable antibiotic dry powders are formulated with less than 3% moisture content. This moisture content cutoff was adopted to preserve powder aerosolization and deposition properties as particles that had moisture content above 3% tended to agglomerate, altering powder properties. Beyond preserving powder properties, stabilization via residual moisture reduction is an important factor in preserving potency by inhibiting microorganism growth and other water-facilitated degradation (May et al. Citation1986). To ensure the moisture content remains less than 3% and that the antibiotics are not exposed to UV, oxidants or other contaminants, the antibiotic dry powder can be single-dose-packaged in tightly sealed aluminum foil-polymer film laminate blister packs. Multiple blister packs can be sealed in a foil overwrap with molecular sieve desiccant. This foil laminate unit-dose blister pack improves the safe use of each dose by mitigating the dangers of UV, oxidant inactivation, and general contamination. Moisture content analysis and the monitoring of crystal structure are important for evaluating powder stability and the success of packaging strategies. Powder moisture content is analyzed using coulometric Karl Fischer titration. Similarly, evaluating the structure of the microparticles using X-ray diffraction (XRD) ensures that the powders remain unchanged (crystalline or amorphous) throughout the storage and usage period.
The moisture contents of the capreomycin, kanamycin, and isoniazid formulations indicated the consistency of drying. The capreomycin formulation contained 1.52% moisture with a standard deviation of 0.10% (n = 3). The kanamycin formulation contained 2.70% moisture with a standard deviation of 0.42%. Lastly, the isoniazid formulation contained 1.79% moisture with a standard deviation of 0.24%.
The crystallinity of capreomycin, kanamycin, and isoniazid microparticles formulated with 20% L-leucine were analyzed using pXRD. Capreomycin and kanamycin formulated microparticles are amorphous in character while the isoniazid microparticles show significant crystallinity. The Diffraction Management System for NT (DMSNT) was used to match the peaks in the isoniazid formulation diffractogram to the isonicotinic acid hydrazide reference diffractogram in the International Centre for Diffraction Data database. The isoniazid formulation diffractogram, the DMSNT identified peaks and the matching isonicotinic acid hydrazide peaks are shown in . The peaks from the isoniazid formulation closely match the isonicotinic acid hydrazide peaks indicating that the crystallinity is from the isoniazid in the formulation rather than the L-leucine. This is further supported by the lack of crystallinity in the capreomycin and kanamycin formulations, which contain the same proportion of L-leucine.
FIG. 5 A comparison of the Fine Particle Fractions (FPF) for capreomycin, kanamycin, and isoniazid from two devices, Aerolizer and Puffhaler at 28.3 L/min. The last three columns of data compare the FPFs < 3.3 μm when flow rate is increased from 28.3 L/min to 60 L/min using the Aerolizer.
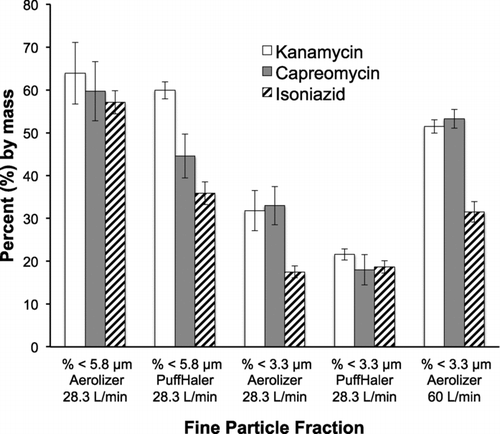
The FPF is dependent on both the device and the flow rate. To examine device effects, the FPFs of the drug formulations were measured with administration from both the Aerolizer and the PuffHaler at 28.3 L/min. shows a side-by-side comparison of the ACI results for fine particle fraction (FPF) using the PuffHaler to disperse capreomycin, isoniazid, and kanamycin microparticles from foil laminate blister packs and the Aerolizer to disperse the antibiotic formulations from capsules. These results described in illustrate that the antibiotic formulations have higher FPFs when dispersed from the Aerolizer. The isoniazid formulation generally had lower FPFs, except for the FPF less than 3.3 μm from the PuffHaler, which was comparable to the kanamycin and capreomycin formulations. The formulation of isoniazid is still being further developed with an emphasis on the improvement of its homogeneity, FPF, and ED.
To examine how flow-rate impacts the FPF less than 3.3 μm the antibiotic formulations were tested using the Aerolizer at a higher flow rate. Initially, the 28.3 L/min flow rate was adopted for comparability to other projects in the laboratory, in which 28.3 L/min was used as the standard test flow rate. As the tuberculosis antibiotics are likely to benefit a broad range of patients, with a variety of inspiration capacities, the antibiotic formulations were also tested using the Aerolizer as a dispersion device at 60 L/min. The Aerolizer was chosen as the test device for comparing flow rates because the PuffHaler was specifically designed to be used at the lower 28.3 L/min flow rate, whereas the Aerolizer is designed for use at 60 L/min. The collapsible reservoir of the PuffHaler does not favor improved dispersion at higher flow rates due to particle trapping. The three antibiotic formulations were compared to the lower flow rate data to examine how flow rate affects FPF, specifically the FPF less than 3.3 μm. The results in the last three columns of illustrate that at a higher flow rate of 60 L/min, FPF less than 3.3 μm increases for all drug formulations, indicating that the higher energy inspiration is capable of breaking apart agglomerates of smaller particles.
The increase in FPF less than 3.3 μm is supported by SEM images of the antibiotic formulations, which show agglomerates of smaller particles. The higher energy of the 60 L/min flow rate is capable of dispersing these agglomerates so that the particles can reach the ACI stages as individual particles, thus increasing the FPF. In , the SEM images of the three antibiotic formulations illustrate the smaller particles in larger agglomerates.
Of particular importance in the device and testing design, the PuffHaler () incorporates POLYBATCH VLA 55 UK, antistatic material, within the polyethylene film used for the reservoir. Prior to this innovation, an earlier tested nonconductive polymer film in the PuffHaler reservoir retained an unacceptable amount of powder; the emitted dose out of the PuffHaler was approximately 20%. After switching to the POLYBATCH VLA 55 UK polyethylene film the emitted dose increased to 50% with a placebo powder. The ED of the capreomycin, kanamycin, and isoniazid formulations from the PuffHaler at 28.3 L/min are 61.5% +/− 7.5%, 62.6% +/− 4.3%, and 42.1% +/− 8.2%, respectively.
FIG. 6 A comparison of four potential materials for improving the emitted dose from the PuffHaler. The reservoir materials were tested by dispersing placebo powder from reservoirs constructed from each of the test materials. The content and processing conditions for the placebo powder are described in the methods section.
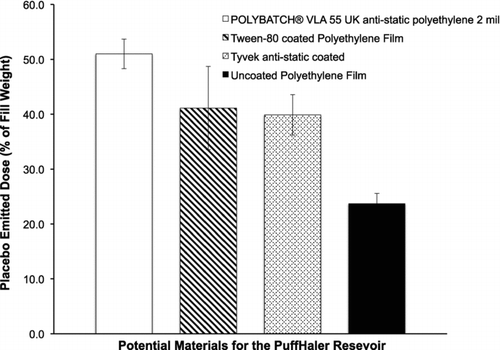
The antistatic agent is FDA-approved for use in food-grade polyethylene films up to 10% by weight for most foodstuffs. It is present in the polyethylene film material used to make the PuffHaler reservoir at a concentration of about 3%. POLYBATCH is incorporated into the surface of the polyethylene film. According to the patent by Amon and Kovalchuk, US patent application number 20090017297 and the manufacturer's description (A. Schulman Inc. Citation2011), the antistatic properties of the film are the result of the formation of a polar layer that absorbs water from the atmosphere. The polar layer is conductive, and dissipates charges on the surface of the film, reducing resistance, and charge decay time. This antistatic polymer was chosen due to its ability to maintain antistatic properties at relatively high humidity, an important characteristic for any future DPI development specifically for inhalable antibiotics, which may be used in nonclimate controlled settings. shows a comparison of several PuffHaler test reservoir materials. The reservoir material tests were conducted with the placebo powder, which is described in the methods. These results illustrate the effectiveness of the POLYBATCH VLA 55 UK antistatic polyethylene for improving emitted dose from the PuffHaler.
CONCLUSIONS
CAN-BD is a powerful supercritical/near-critical fluid spray-drying technique for creating and drying microparticles of antibiotics formulations. Allied with protective unit-dose packaging and a low cost dry powder inhaler, CAN-BD microparticle processing can make a strong impact on shifting the tuberculosis treatment paradigm toward a needle-free solution. The above work illustrates the feasibility of creating inhalable treatment particles in a suitable aerodynamic size range for inhalation therapy of tuberculosis. By exploiting the aerodynamic properties of the inhalable antibiotic formulations, particles can be targeted to the deep lung where granulomas harboring hard to reach bacterial populations extend treatment times, and increase the potential for the development of drug resistance. Beyond aerodynamic properties, the inhalable antibiotic microparticle formulations perform well in different DPIs and contain low moisture contents, which are helpful for maintaining the integrity of the microparticles. The desirable powder properties of these anti-TB antibiotics, combined with the important packaging technologies developed for the inhalable measles vaccine dry powder project, may pave the way for new pulmonary treatments that reduce treatment time as well as the dangers associated with traditional syringe delivery, while also increasing coverage to high-risk populations.
Acknowledgments
This work was funded in part by Grant 1077 from the Foundation for the National Institutes of Health through the Grand Challenges in Global Health Initiative, and a University of Colorado Butcher Grant award. The authors thank D.J. Bennet, M. Higgins, K. Kisich, D. Krank, L. Nicholson, P. Pathak, B. Quinn, N. Shah, N. Varaksa, and S. Winston, for their technical support.
REFERENCES
- A. Schulman Inc. 2011 . Additive: Antistatic Reduce Static Charging , A. Schulman Inc. .
- Borsje , P. , de Jongste , J. C. , Mouton , J. W. and Tiddens , H. 2000 . Aerosol Therapy in Cystic Fibrosis: A Survey of 54 cf Centers . Pediat. Pulmonology , 30 : 368 – 376 .
- Bosquillon , C. , Lombry , C. , Preat , V. and Vanbever , R. 2001 . Influence of formulation excipients and physical characteristics of inhalation dry powders on their aerosolization performance . J. Control. Release , 70 : 329 – 339 .
- Cape , S. P. , Villa , J. A. , Huang , E. T. S. , Yang , T. H. , Carpenter , J. F. and Sievers , R. E. 2008 . Preparation of Active Proteins, Vaccines and Pharmaceuticals as Fine Powders Using Supercritical or Near-Critical Fluids . Pharm. Res. , 25 : 1967 – 1990 .
- Chanwong , S. , Maneekarn , N. , Makonkawkeyoon , L. and Makonkawkeyoon , S. 2007 . Intracellular Growth and Drug Susceptibility of Mycobacterium tuberculosis in Macrophages . Tuberculosis , 87 : 130 – 133 .
- Crowder , T. M. , Rosati , J. A. , Schroeter , J. D. , Hickey , A. J. and Martonen , T. B. 2002 . Fundamental Effects of Particle Morphology on Lung Delivery: Predictions of Stokes’ Law And the Particular Relevance to dry Powder Inhaler Formulation and Development . Pharm. Res. , 19 : 239 – 245 .
- Girling , D. J. 1989 . A Controlled Trial of 3-Month, 4-Month, and 6-Month Regimens of Chemotherapy for Sputum-Smear-Negative Pulmonsrt Tuberculosis-Results at 5 Years . Am. Rev. Respir. Dis. , 139 : 871 – 876 .
- Giudice , E. L. and Campbell , J. D. 2006 . Needle-Free Vaccine Delivery . Adv. Drug Deliver. Rev. , 58 : 68 – 89 .
- Heifets , L. 1988 . Mic as a Quantitative Measurement of the Susceptability of Mycobacterium avium Strains to Seven Antituberculosis Drugs . Antimicrob. Agents Ch. , 32 : 1131 – 1136 .
- Heifets , L. B. , Lindholmlevy , P. J. and Flory , M. 1991 . Comparison of Bacteriostatic and Bactericidal Activity of Isoniazid and Ethionamide Against Mycobacterium avium and Mycobacterium tuberculosis . Am. Rev. Respir. Dis. , 143 : 268 – 270 .
- Helms , R. , Quan , D. , Herfindal , E. and Gourley , D. 2006 . Textbook of Therapeutics: Drug and Disease Management - 8th ed , Philadelphia : Lippincott Williams & Wilkins .
- Hindle , M. and Byron , P. R. 1995 . Dose Emissions from Marketed Dry Powder Inhalers . Int. J. Pharm. , 116 : 169 – 177 .
- Hiyama , J. , Marukawa , M. , Shiota , Y. , Ono , T. and Mashiba , H. 2000 . Factors Influencing Response to Treatment of Pulmonary Tuberculosis . Acta Med. Okayama , 54 : 139 – 145 .
- Horsburgh , C. R. , Feldman , S. and Ridzon , R. 2000 . Practice Guidelines for the Treatment of Tuberculosis . Clin. Infect. Dis. , 31 : 633 – 639 .
- Jovanovic , N. , Bouchard , A. , Hofland , G. W. , Witkamp , G. J. , Crommelin , D. J. A. and Jiskoot , W. 2004 . Stabilization of Proteins in dry Powder Formulations Using Supercritical Fluid Technology . Pharm. Res. , 21 : 1955 – 1969 .
- Kisich , K. O. , Higgins , M. P. , Park , I. , Cape , S. P. , Lindsay , L. Bennett , D. J. 2011 . Dry Powder Measles Vaccine: Particle Deposition, Virus Replication, and Immune Response in Cotton Rats Following Inhalation . Vaccine , 29 : 905 – 912 .
- Lin , W. H. , Griffin , D. E. , Rota , P. A. , Papania , M. , Cape , S. P. Bennett , D. 2011 . Successful Respiratory Immunization with Dry Powder Live-Attenuated Measles Virus Vaccine in Rhesus Macaques . Proc. Nat. Acad. Sci. U S A , 108 : 2987 – 2992 .
- May , J. C. , Wheeler , R. M. and Grim , E. 1986 . The Determination of Residual Moisture in Several Freeze-Dried Vaccines and a Honey-Bee Venom Allergenic Extract by tg/ms . J. Therm. Anal. , 31 : 643 – 651 .
- Mitchell , J. , Nagel , M. , Doyle , C. , Ali , R. , Avvakoumova , V. Christopher , J. 2010 . Relative Precision of Inhaler Aerodynamic Particle Size Distribution (apsd) Metrics by Full Resolution and Abbreviated Andersen Cascade Impactors (acis): Part 1 . AAPS Pharm. Sci. Tech. , 11 : 843 – 851 .
- Mitragotri , S. 2005 . Immunization Without Needles . Nat. Rev. Immunol. , 5 : 905 – 916 .
- Muttil , P. , Wang , C. C. and Hickey , A. J. 2009 . Inhaled Drug Delivery for Tuberculosis Therapy . Pharm. Res. , 26 : 2401 – 2416 .
- Namgyal , P. 2006 . Introduction of New Vaccines in the Asia Pacific Region: Challenges and Opportunities . World Health Organization , Geneva
- Nuermberger , E. L. , Yoshimatsu , T. , Tyagi , S. , O’Brien , R. J. , Vernon , A. N. Chaisson , R. E. 2004 . Moxifloxacin-Containing Regimen Greatly Reduces Time to Culture Conversion in Murine Tuberculosis . Am. J. Resp. Crit. Care , 169 : 421 – 426 .
- Pandey , R. and Khuller , G. K. 2005 . Antitubercular Inhaled Therapy: Opportunities, Progress and Challenges . J. Antimicrob. Chemoth. , 55 : 430 – 435 .
- Rebits , L. G. , Bennett , D. J. , Bhagwat , P. A. , Morin , A. and Sievers , R. E. 2007 . Method for Quantifying the Sample Collected by an Andersen Cascade Impactor Using Total Organic Carbon Analysis . J. Aerosol Sci. , 38 : 1197 – 1206 .
- Shakoor , O. , Taylor , R. B. and Behrens , R. H. 1997 . Assessment of the Incidence of Substandard Drugs in Developing Countries . Trop. Med. Int. Health , 2 : 839 – 845 .
- Shin , S. Y. , Furin , J. , Alcantara , F. , Hyson , A. , Joseph , K. Sanchez , E. 2004 . Hypokalemia Among Patients Receiving Treatment for Multidrug-Resistant Tuberculosis . Chest , 125 : 974 – 980 .
- Sievers , R. E. , Cape , S. P. , Quinn , B. P. , Searles , J. A. , Best , J. A. Burger , J. L. 2006 . Stabilization, Nebulization, and Near-Ambient Temperature Drying of Measles Vaccine, Igg Antibody, tb Antibiotics, and Zanamivir . Resp. Drug Deliv. X , 2 : 401 – 404 .
- Sievers , R. E. , Huang , E. T. S. , Villa , J. A. , Kawamoto , J. K. , Evans , M. M. and Brauer , P. R. 2001 . Low-Temperature Manufacturing of Fine Pharmaceutical Powders with Supercritical Fluid Aerosolization in a Bubble Dryer (r) . Pure Appl. Chem. , 73 : 1299 – 1303 .
- Sievers , R. E. , Quinn , B. P. , Cape , S. P. , Searles , J. A. , Braun , C. S. Bhagwat , P. 2007 . Near-Critical Fluid Micronization of Stabilized Vaccines, Antibiotics and Anti-Virals . J. Supercrit. Fluid , 42 : 385 – 391 .
- Stelmach , R. , Bussamra , M. H. , Rodrigues , J. C. and Cukier , A. 2009 . A Randomized, Comparative Study of Formoterol and Terbutaline Dry Powder Inhalers in the Treatment of Mild to Moderate Asthma Exacerbations in the Pediatric Acute Care Setting . Ann. Allerg. Asthma, Im. , 103 : 248 – 253 .
- Taylor , R. B. , Shakoor , O. and Behrens , R. H. 1995 . Drug Quality, a Contributor to Drug-Resistance . Lancet , 346 : 122 – 122 .
- Traini , D. , Adi , H. , Young , P. M. , Chan , H. K. and Agus , H. 2010 . Co-Spray-Dried Mannitol-Ciprofloxacin Dry Powder Inhaler Formulation for Cystic Fibrosis and Chronic Obstructive Pulmonary Disease . Eur. J. Pharm. Sci. , 40 : 239 – 247 .
- Tyagi , S. , Nuermberger , E. , Yoshimatsu , T. , Williams , K. , Rosenthal , I. Lounis , N. 2005 . Bactericidal Activity of the Nitroimidazopyran pa-824 in a Murine Model of Tuberculosis . Antimicrob. Agents Ch. , 49 : 2289 – 2293 .
- Varmus , H. , Klausner , R. , Zerhouni , E. , Acharya , T. , Daar , A. S. and Singer , P. A. 2003 . Grand Challenges in Global Health . Science , 302 : 398 – 399 .
- Vianna , E. O. , Souza , M. L. D. , Meneghini , A. C. , Ferraz , E. and Borges , M. C. 2009 . Knowledge of and Technique for Using Inhalation Devices Among Asthma Patients and Copd Patients . J. Bras. Pneumol. , 35 : 824 – 831 .
- 2011 . Frequently Asked Questions About tb and hiv . World Health Organization, Geneva, , http://www.who.int/tb/hiv/faq/en/ World Health Organization
- Wolf , A. J. , Linas , B. , Trevejo-Nunez , G. J. , Kincaid , E. , Tamura , T. , Takatsu , K. and Ernst , J. D. 2007 . Mycobacterium tuberculosis Infects Dendritic Cells with high Frequency and Impairs Their Function in Vivo . J. Immunol. , 179 : 2509 – 2519 .