Abstract
Many nanoparticle collection devices have limitations related to retention of particle integrity from bounce, shattering, or aggregation. Suspensions of soft nanoparticles (e.g., proteins, lipids) are required for drug delivery and therapy. To enable direct collection of soft nanoparticles into liquid media, a wet electrostatic precipitator (WESP) was designed and evaluated in this work. Different sections were used for ion generation and particle charging, for minimal contact between the corona wire and particles, which were charged using positive nitrogen ions. WESP dimensions and operating parameters were optimized using charge distribution modeling. The prototype WESP was designed for operation with a continuous flow of liquid over the collection plate, to allow continuous particle collection from the exit stream of an aerosol reactor. The collection efficiency of the WESP, in dry and wet modes, was measured using aerosols of monodisperse polystyrene latex (PSL), polydisperse sucrose, and stearic acid (soft lipid) particles, through SMPS measurements, corrected for diffusional losses, at the entry and exit of the device. Measured collection efficiency was 70%–90% for particles of sizes 80–600 nm diameter in reasonable agreement with theoretical estimates. However, for small particles (20–80 nm diameter) measured collection efficiency ranged 40%–70%, significantly lower than theoretical estimates, possibly from incomplete neutralization of negative charges attained during air-jet atomization. Transmission electron microscopy (TEM) images and dynamic light scattering (DLS) measurements confirm that wet collection produces a suspension of free, unaggregated nanoparticles with sizes similar to their measured mean mobility diameter.
Copyright 2012 American Association for Aerosol Research
1. INTRODUCTION
Soft nanoparticles, for example, those made from proteins, lipids, sugars, or those of biological origin (e.g., viruses) have applications in the medical field (Almeida et al. Citation1997; Muller et al. Citation2001). Collection of soft nanoparticles into a liquid medium is needed for preparation of drug suspensions. Drug-loaded nanoparticle suspensions are characterized through measurement of particle properties including biological analysis (Dixkens and Fissan Citation1999), measurement of size in suspension, drug loading, and release rates (Westesen et al. Citation1997). Currently, nanometer sized particles are collected by filtration (Steffens and Coury Citation2007), impaction (Fernandez de la Mora et al. 1990; Hogan et al. Citation2005), thermophoretic (Wen and Wexler Citation2007), and electrostatic precipitation (Mainelis et al. Citation2002b) wherein respective mechanisms of particle collection are inertial, Brownian, thermophoretic, and electrostatic deposition (Hinds Citation1982). Liquid impingement had been used for collecting nanoparticles directly into liquid (Wei et al. Citation2010) for preparing samples that can be used for imaging, properties measurement, and biological analysis (Miljevic et al. Citation2009).
Key concerns in soft nanoparticle collection include need of a gentle collection mechanism along with high efficiency. In filter-based methods, particles collected have to transfer from filter to suspension by sonication, which leads to physicochemical change in particle properties (Berube et al. Citation1999). Conventionally, delicate bioaerosols are collected using all-glass impingers and slot impingers, in which use of a smaller volume of collecting liquid causes bounce of particles, while a larger volume causes re-aerosolization of particles (Grinshpun et al. Citation1997). Aerosol collection using impactors had been shown to adversely affect the viability of the soft, biological particles (Lin Citation2000). Thermophoretic collection with moderate efficiency requires very high, unfeasible temperature gradients (105 K/cm), which would be disruptive to bioaerosols (Wen and Wexler Citation2007). In impactors, particles are collected on hard surfaces where face velocity of the particles is high enough to cause break-up as well as agglomeration (Utsunomiya et al. Citation2004). In electrostatic precipitators (ESP), migration velocity of particles is 2 to 4 orders of magnitude lower than in impactors and impingers (Mainelis et al. Citation2002a), reducing chances of break-up. However, collected particles may fall upon one another forming lumps and aggregates. Wet electrostatic precipitation (WESP), in which particles are collected directly into a liquid medium, could overcome these disadvantages.
Wet electrostatic precipitators (WESPs) are widely used in industry for removal of micron-sized particles from effluent gases. This method is extensively used for sticky particles where they are collected on a plate and then washed off the surface by flowing water (Bologa et al. Citation2009). Previous research on electrostatic precipitation to different surfaces included use of an electrostatic aerosol sampler (EAS) (Model 3100, TSI Inc., St. Paul, MN) for collection of bacteria species (BG spores) onto agar, into water and onto filters (Mainelis et al. Citation1999; Citation2002a; Citation2002b). It was reported that a small amounts of ionization of particles in the ESP inlet increased the overall collection efficiency to 80%–90% for biological as well as nonbiological particles, depending on precipitation voltage. A portable, dry ESP was developed for use as a TEM sampler of ultrafine atmospheric particles showing sufficient particle collection for low exposure time for charging (Fierz et al. Citation2007). The present work undertakes design and fabrication of a WESP for direct collection of soft nanoparticles (20–600 nm in diameter), into liquid media, to make unaggregated, nanoparticle suspensions, intended for use in drug therapy as this size range of particles are relevant for targeting drugs to tumors and brain disease and therefore suitable for drug delivery (Pawar and Venkataraman Citation2011). To allow continuous collection of soft nanoparticles synthesized in an aerosol reactor, the WESP was designed to operate with continuous liquid flow over the collection plate.
FIG. 2 Schematic diagram of experimental set up used for measuring number based collection efficiency of the fabricated wet electrostatic precipitator (WESP) from mobility based size distribution.
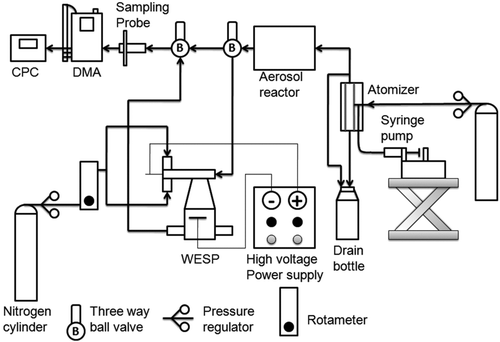
The specific objectives of the present work are as below:
1. | Design and fabrication of a WESP for collection of soft nanoparticles directly into liquid media to make nanoparticle suspensions. | ||||
2. | Measurement of the size dependent collection efficiency of soft nanoparticles onto dry and wet media. | ||||
3. | Evaluation of the extent of aggregation of particles, using transmission electron microscope (TEM) imaging of particles collected onto dry media (TEM grid on plate) and into suspension (transferring suspension drop on TEM grid). |
2. DESIGN APPROACH
The WESP was designed and sized using theoretical equations available in literature (Fuchs Citation1963; White Citation1963) using the steps shown in and described in detail in supplemental Information available online with this publication (online Supplemental Information). The geometrical parameters of ion generation, charging and collection section were estimated using an iterative method which yielded an assumed minimum 70% theoretical collection efficiency for the size range of particles of interest (20–600 nm in diameter). Geometrical parameters of ion generation section (e.g., length of the section, corona and cylinder diameter) were assumed first to predict the threshold voltage for onset of corona. The minimum ion concentration in the section was calculated, followed by number of charges on particles of different sizes, using charge distribution calculations. Assuming a particular geometry of the charging section fixed the residence time available for charging (). The area of the collection plate was fixed and corresponding collection efficiency of particles of different sizes was calculated. The geometrical dimensions were varied until the assumed minimum collection efficiency was obtained.
3. MATERIALS AND METHODS
3.1. Experimental Setup
The experimental setup () used for measuring performance of the WESP in terms of collection efficiency consisted of three sections: an aerosol generation section, a particle collection device (WESP) and an aerosol measurement system (for measuring mobility based size distribution of aerosolized nanoparticles). The setup used for generating nanoparticles comprised of a Collison-type air jet atomizer having an output rate of 50 cm3/s (Model 3076, TSI Particle Instruments Inc., St. Paul, MN, USA) and a reactor tube. The precursor solution was fed to the atomizer by using a syringe pump (Model NE 300 syringe pump, New Era Pump Systems Inc., USA). The reactor, used in previous work (Pawar and Venkataraman Citation2011), from which the stream was sampled for particle size measurement.
For measuring size distribution of particles, a scanning mobility particle sizer was used (SMPS, Model 3936, TSI Inc. Particle Instruments, St. Paul, MN, USA) that consisted of a differential mobility analyzer (Long DMA, Model 3081, TSI Inc. Particle Instruments, St. Paul, MN, USA) and condensation particle counter (CPC, Model 3775, TSI Inc. Particle Instruments, St. Paul, MN, USA).
3.2. Features of Wet Electrostatic Precipitator (WESP)
In the WESP prototype designed and fabricated in this study (), ions were generated by using an integrated positive corona discharge by ionizing nitrogen gas molecules to prevent ozone formation. The ions were mixed with aerosolized nanoparticles, which were collected on a liquid medium kept on a negatively charged brass plate. The prototype had different sections for ion generation and particle charging to ensure no particle deposition on corona wire ensuring constant field and constant rate of ion generation. No electric field was applied in the charging section, causing particles to be charged only by diffusion charging that is a material-independent charging process (Flagan and Seinfeld Citation1988). All sections of the WESP were sized using calculations described in the design section to achieve a theoretical collection efficiency of 70%, which is reported to be a typical expected value for nanoparticle collection (Mainelis et al. Citation2002b).
FIG. 3 Cross sectional view of fabricated wet electrostatic precipitator (WESP) (all dimensions are in mm).
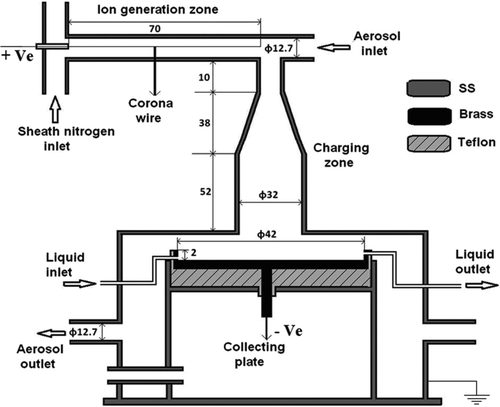
In the ion generation section, a 7 cm long platinum-rhodium (1:1) wire, encased in a 0.5 inch diameter grounded stainless steel (SS 316) tube, was used to create a corona discharge. Positive voltage was applied on the wire to minimize ozone formation. A high voltage DC power supply (Range: 0–10 kV; Model HVPS 2, Polltech Instruments, Mumbai, India) was used for maintaining the wire and collection plate at high voltages of opposing polarity. Pure nitrogen gas (99.99% pure, Med N Gas Equipment, Mumbai, India) at a flow rate of 50 cm3/s was passed through this section for generating positive nitrogen ions while the aerosol was introduced in counter-flow mode at the same flow rate. The equal and opposite flow rates of aerosol and sheath nitrogen minimized contact between particles and the corona wire was intended to minimize changes in wire friction factor from particle deposition and lead to a constant ion concentration during operation. In all experiments, the corona voltage was fixed at +4.2 kV that was higher than measured threshold voltage for generating sufficient nitrogen ions for charging the particles. In the charging section, particles are charged because of diffusion since no voltage was applied to minimize particle loss. A 0.7 s time was provided for charging submicron size particles in the charging section. Charged particles were sent to collection section that consisted of a brass plate having diameter of 4.2 cm and separated from the entire SS structure by Teflon insulation. The collecting plate was maintained at a voltage of −3.5 kV. Charged particles were collected on the plate, while particle free gas exited the outlet of the WESP.
3.3. Experimental Approach
Two sizes (91 nm and 150 nm) of standard monodisperse polystyrene latex (PSL) particles, generated from 0.005 mg/mL PSL suspensions in de-ionized water (DI water), were used for measuring variation in collection efficiency with size of particle. Polydisperse sucrose and stearic acid particles in the size range of interest (20–600 nm), generated from atomization of precursor solutions, were used for collection efficiency measurement on both dry and wet surfaces. Atomization of a 1 mg/mL solution of sucrose (Sucrose GR, Merck Specialities Private Limited, Mumbai, India) in DI water and a 1 mg/mL solution of stearic acid (>95.0% pure; Sigma Aldrich, St. Louis, MO, USA) in chloroform (>99.0% pure; Sigma Aldrich, St. Louis, MO, USA) (Pawar and Venkataraman Citation2011) were used, to generate sucrose and stearic acid nanoparticles, respectively. A 20 mL volume of precursor solution was placed in a BD syringe (Becton Dickinson India Pvt. Ltd., Haryana, India) and delivered to the atomizer at a flow rate of 0.6 mL/min.
At the start of each experiment, the system was operated with no particle generation until the measurement system (SMPS) detected zero particle concentration. The operating regime of Long DMA used for size distribution measurement was 14–600 nm with a cycle time of 135 s. The WESP was attached between the aerosol generation system and the SMPS in a parallel configuration. The flow paths of aerosolized particles were controlled by using two three-way ball valves to enable measurement of particle size distribution at the entry and exit of the dry ESP/WESP (with and without applied voltages on corona wire and collecting plate). These size distributions measurements were used for measuring diffusional losses in the prototype along with its performance in terms of number-based collection efficiency. The measurements for wet collection efficiencies were performed in batch mode where, 5 mL of 100 mg/mL sodium chloride (Merck Specialities Private Limited, Mumbai, India) solution (Solvent: DI water) was placed on collecting plate forming a film of ∼0.5 mm thickness to collect the nanoparticles directly on the liquid medium because of high electrical conductivity of salt-water. For measuring overall mass based collection efficiency, an aluminum foil was placed on collection plate, using a film of salt water to make an electrical connection to the plate. Three repeats of all experiments were made and mean and standard deviations reported for statistical confidence.
3.4. Measurement of Particle Mobility Distribution and Collection Efficiency
Particle mobility diameter distributions were measured by DMA sizing in different bins of 14–600 nm and CPC number counts in each size bin. Size distribution measurements at entry and exit of dry and wet ESP in the absence of plate voltage (Corona voltage: +4.2 kV; collection voltage: 0 kV) were used for measuring diffusional losses inside the device. Total collection efficiency of each size bin was calculated in terms of total collection efficiency, η total:
Where n out and n in are the particle concentration downstream and upstream of ESP (Corona voltage: +4.2 kV; collection voltage: 3.5 kV) for that size bin. Separate experiments were done for measuring total collection efficiency and diffusional losses flowed by total collection efficiency was corrected by subtracting diffusional losses in each size bin, to arrive at collection efficiency from electrostatic deposition. However, overall number based collection efficiency was calculated from overall number concentrations followed by correction due to diffusional losses. For mass based collection efficiency measurement dry, preweighed aluminum foil (0.027 mm thick and 41 mm diameter) were placed on the collecting plate and particles were collected for 10 min. Aluminum foils were cleaned using a standard procedure prior to weighing, and the mass collected (m collected) was calculated from the difference between pre- and postsampling weights. Atomizer output (0.1 mL/min) with solute concentration in precursor solution determined the mass of solute discharged (m discharge) from the atomizer. These two measurements of masses were used for calculating mass based collection efficiency as:
3.5. Particle Structure, After Collection, by TEM Imaging
The extent of aggregation because of particle–particle interaction was investigated by comparing dry and wet collection. Images were taken using a field emission scanning transmission electron microscope (FE-TEM, Model: JEM-2100F, JEOL Ltd., Peabody, USA) with an electron beam of accelerating voltage 200 kV.
In dry collection mode, a TEM grid was attached to the dry collection plate. In wet collection mode, a 10 μL volume of nanoparticle suspension, collected into same liquid media as explained earlier in the WESP was transferred to the TEM grid followed by drying. Further, addition of salt into water would increase particle collection due to increase in conductivity but that would affect the particle properties used for drug delivery. In both the cases, particles were collected for a period of 10 min to have sufficient number of particles on the TEM grids. A negative aqueous stain (Uraynl acetate, passed through 200 nm filter) of mass concentration 1% w/w was used for staining the TEM grid.
3.6. Particle Size in Suspension
The composition of the liquid medium was optimized to enable the highly hydrophobic stearic acid nanoparticles to remain in suspension. Sodium cholate (Sodium cholate hydrate, >99% pure, Sigma Aldrich, St. Louis, MO, USA), surfactant solution in DI water was used as the liquid collection medium. A concentration of 2 mg/mL was used, below critical micelle concentration (CMC) (3.8 mg/mL) to ensure negligible micelle formation. The WESP was operated in continuous collection mode with an average liquid flow rate of 20 μL/s during the operating cycle of the aerosol reactor to obtain a lipid nanoparticle suspension. Size distributions of the particles suspended in collection liquid were measured by dynamic light scattering (DLS) method using Zetasizer (Model: Zetasizer Nano ZS, Malvern Instruments Limited, Malvern, UK).
4. RESULTS AND DISCUSSION
4.1. Voltage-Current (V-I) Characteristics of Corona
Geometrical parameters of the ion generation section (i.e., corona wire diameter, length, and grounded cylinder diameter) were fixed at 0.05, 7, and 1.27 cm respectively. From these parameters, corona threshold voltage for ion generation was measured as ∼3.5 kV. The measured threshold voltage was used for estimating the friction factor of wire followed by theoretical V–I characteristics of the wire. These characteristics were measured for three different flow rates (0, 50, and 100 cm3/s) of sheath nitrogen in order to compare with theoretical results. Variation of generated current at corona wire with applied voltage is shown in . With increasing voltage, current also increases showing a higher generation rate of free ions. From the graph, it is clear that theoretical V–I characteristics were close to experimental one in the absence of sheath nitrogen for low applied voltages. It was not possible to compare the ion generation at different sheath gas flow rates with theoretical results because the theoretical equation did not include a gas-flow term in it. At high voltages, for no flow of sheath nitrogen, measured current was unstable (high error bars) and was not comparable with theoretical results. Experimental V–I characteristic measurements were limited from threshold to spark-over Voltage (3.2–5.7 kV), therefore, theoretical threshold values were compared with experimental one in this range only. Increasing flow rate of nitrogen had no significant effect on the threshold voltage but current for a particular applied voltage was higher for higher flow rate of sheath nitrogen indicating higher generation rate of ion due to increase in flow rate.
4.2. Charge Distribution Calculations
For designing the WESP, the applied voltage at corona wire was set at 4.2 kV, higher than measured threshold voltage (∼3.5 kV) that produced a corona current of 4 μA. Minimum ion concentration in the charging section was calculated as 1.29 × 1015 ions/m3. Using the geometry of the charging section and the selected aerosol and sheath gas flow-rates, residence time of the aerosolized nanoparticles in that section was calculated as 0.7 s. To estimate charge distribution on particles, combination coefficients (β) were calculated first by numerical integration of the equations (Equations S10–S13; Adachi et al. 1985; Zhuang et al. 2000) using MATLAB (Version 7.8.0.347 [R2009a], The MathWorksTM, USA). Ionic parameters () used in previous work (Li and Christofides Citation2006) were used for these calculations (Kennard Citation1938; Bricard and Pradel Citation1966). Combination coefficients were calculated between particles and positive ions, generated in the current WESP prototype, and the resulting positively charged particles (). Negative values of combination coefficients, which are impractical, are not shown in the graph. Consistent with expectation, combination coefficients for a particular size of particle decrease as the number of charges on the particle increases. This is because of increase of electrostatic repulsive force acting between charged particles and same polarity ions. The graph also indicates that the numerical value of combination coefficient increases as the particle size increases because of larger surface area for accumulating charges. For particles smaller than 15 nm diameter, the numerical value of the only applicable β 0 is positive, therefore, particles in this range can attain a maximum of one charge.
TABLE 1 Ionic parameter used for simulating diffusion charging model
FIG. 5 Combination coefficients (β) of diffusion charging between sucrose nanoparticles (1–100 nm) and positive nitrogen ions (p represents the subscript of β).
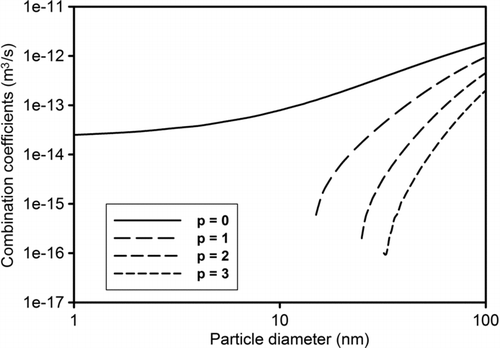
Using the numerical values of combination coefficients, the charge distribution on particles was calculated using unipolar diffusion charging equations (Equations S6–S9; Liu and Pui 1977; Wiedensohler and Fissan 1991; Hussin et al. 1983; Adachi et al. 1985) solved in MATLAB (Version 7.8.0.347 [R2009a], The MathWorksTM, USA). Particles synthesized through air-jet atomization are expected to have bipolar charges; however, a neutralizer was not used, in keeping with the objective of designing a simple collection device for soft nanoparticles of mean diameter around 100 nm, relevant to drug delivery applications. It is expected that in the unipolar positive-ion charging section, negatively charged particles would be neutralized very quickly (because of large numerical values of combination coefficients) and then charged positively. However, neutralization of particles using bipolar charging followed by unipolar charging can increase collection efficiency. To demonstrate the unipolar charging behavior of particles in the charging process, the charge distribution as a function of time of charging for sucrose particles of 50 nm sizes is shown (). The graph indicates that the charging fraction is not uniformly distributed for particles in nanosized range, as a result of the dynamic interplay of different combination coefficients. Because –β 0< –β 1< –β 2< –β 3< 0, there is typically a peak in the charging fraction 1 to p-1, while the charging fraction 0 continuously decreases and charging fraction p continuously increases. The average charge on the aerosol (calculated as particle number weighted average among different numbers of charges) monotonically increases with time of charging. Estimated mean charge from charge distribution as a function of particle size was compared with average charge demonstrated by other researchers (White Citation1963) for size range of 10–600 nm (). The mean charge estimated from charge distribution is somewhat higher than that from White (Citation1963), which would allow lower charging time and collection area requirement for getting significant collection efficiency.
FIG. 6 Charge distribution on 50 nm sucrose particles due to diffusion charging (0, 1, 2 … represents number of charge on particles and ion concentration = 1.29 × 1015 ions/m3).
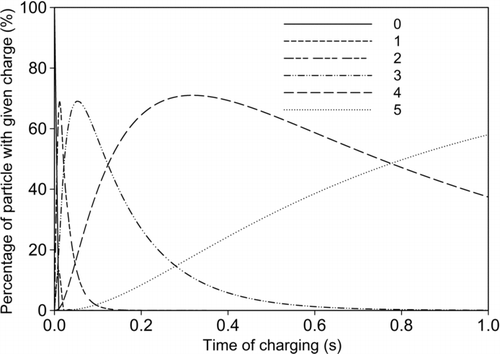
FIG. 7 Percentage deviation of average number of charge by diffusion charging using deterministic (White, Citation1963) from average charge calculated from charge distribution for different charging time (0.4 0.7, and 1 s).
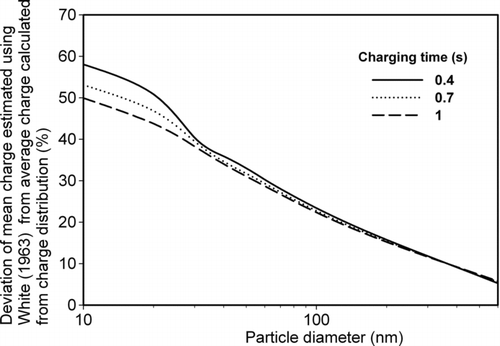
shows that the percentage deviation of mean charge predicted by the White equation, from average charge estimated from charge distribution calculations. Agreement between the two estimates is good (difference <20%) for larger particles (>100 nm), but there is significant disagreement for particles smaller than 100 nm diameter. Some of this difference can be attributed to the estimated average charge from the White equation having a fractional value. In a practical sense particles cannot attain fractional charge. So, charge distribution calculation is more reliable for estimating average charge on particles. Agreement between the methods increases with increasing particle size and charging time.
4.3. Size Dependent Collection Efficiency
The number of charges estimated from the charge distribution calculation was used to calculate migration velocity and resulting theoretical collection efficiency. The measured collection efficiency was calculated in each size range using measured mobility size distributions, corrected for diffusional losses, as explained in Section 3.3. Mean mobility diameter, GSD and total number concentration from mobility size distributions of all 3 types of particles (PSL, sucrose and stearic acid) are tabulated () for wet and dry collection. These size distributions were used for estimating the measured total collection efficiency and diffusional losses followed by collection efficiency due to electrostatic deposition in the designed prototype.
TABLE 2 Count mean diameter (CMD), geometric standard deviation (GSD), and total number concentration of particles size distributions measured after the aerosol reactor and the prototype for with and without applied voltages on collecting plate (V denotes voltage)
Theoretical and measured collection efficiencies, as a function of particle size, are shown in . From theoretical results (Deutsch Citation1922; Friedlander Citation2000), it was concluded that size dependent collection efficiency was a poor function of material electrical properties, e.g., dielectric properties because particles were charged by diffusion charging mechanism only in the current study that is a weak function of dielectric constant. For particles having mobility diameter <50 nm, particle size had almost no effect on collection efficiency. This was because of high migration velocity and collection efficiency of these small particles once they acquire even unit charge. Increasing particle size leads to higher drag, and lower migration velocity, even though particles attain multiple charges leading to lower collection efficiency (Li and Christofides Citation2006). The measured collection efficiency of PSL particles for the two sizes under study was comparable because difference between their diameters was not significant. For polydisperse particles, it was found that collection efficiency increased first to reach a peak and then decreased with increasing mobility diameter. This results from an interplay between increasing charging fraction and decreasing electrical mobility (Li and Christofides Citation2006), with increasing size. For sucrose
FIG. 8 Comparison between calculated and measured size dependent number based collection efficiency of (a) sucrose, (b) stearic acid, and (c) polystyrene latex (PSL) particles in dry electrostatic precipitator (voltage applied on corona wire = +4.2 kV and voltage applied on collecting plate = −3.5 kV).
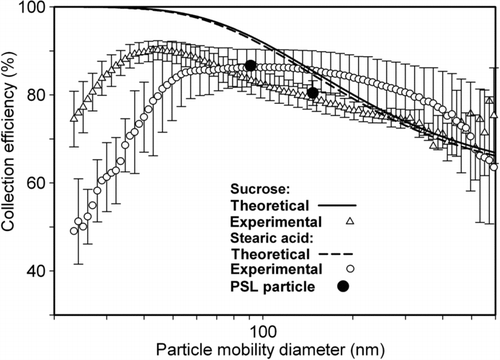
FIG. 9 Comparison between calculated and measured size dependent number based collection efficiency of (a) sucrose, (b) stearic acid, and (c) polystyrene latex (PSL) particles in wet electrostatic precipitator (WESP) (voltage applied on corona wire = +4.2 kV and voltage applied on collecting plate = −3.5 kV).
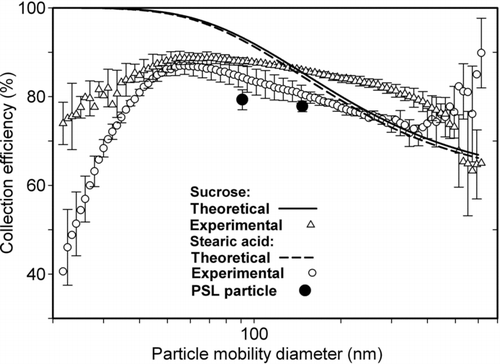
particles, ∼45 nm size particles were collected most efficiently with ∼93% collection efficiency. In case of stearic acid particles, there was a broad flat peak in collection efficiency. Particles having sizes 40–130 nm were collected with >80% collection efficiency. Collection efficiency of sucrose and stearic acid particles can be compared because the current charging technique is independent of dielectric constant. Measured collection efficiency was >70% for all 3 kinds of large particles (80–400 nm diameter) that was in reasonable agreement with respective theoretical values. However, small size particles (20–80 nm diameters) had significant lower collection efficiency than theoretical values. Small sized particles have a low saturation charge capacity. Air-jet atomization, the method used for particle production, typically places negative charges on particles (Tsai et al. Citation2006). The lower rates of charging of small particles (<70 nm in diameter), likely led to their incomplete neutralization during the residence time of 0.7 s provided in the charging section. Therefore, small particles could not attain the positive charge needed for their collection whereas large particles (>70 nm in diameter) did not experience the same because of their large numerical values of combination coefficients (time for neutralizing is <0.1 s).
Wet collection efficiency was also measured for all 3 kinds of particles. Measured, size-dependent collection efficiency in the WESP was compared with theoretical collection efficiency (). Because of lower electrical conductivity of the liquid medium than the metal plate, the voltage attained by the upper surface of liquid is likely to be lower than the voltage attained by plate surface. Therefore, the resulting electrical field strength is likely to be lower in case of WESP than dry ESP for the same operating conditions (plate voltage and corona voltage). The theoretical collection efficiency was not corrected to reflect this difference. Measured collection efficiency trends as a function of particle size obtained in the wet collection were similar to those in dry collection. Collection efficiency obtained in the WESP was just a little lower than that obtained in dry collection (70–80% for particles of 40–130 nm). As explained earlier, further addition of electrolyte salts (like NaCl) could increase the conductivity of the liquid medium, but are not suitable, increase nanoparticle sizes, during re-aerosolization of the suspension.
FIG. 10 TEM images of particles collected in (a, left) dry ESP and (b, right) WESP (voltage applied on corona wire = +4.2 kV and voltage applied on collecting plate = −3.5 kV).
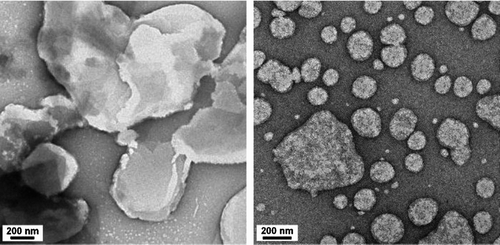
In the wet collection mode, ∼60 nm diameter particles of both polydisperse types (sucrose and stearic acid) were collected with the highest efficiency of ∼85%. The reasonably high particle collection efficiency obtained with the WESP and the additional advantage that collection efficiency does not depend upon particle material properties, make the WESP designed here suitable for collection of particles made of different materials with about the same collection efficiencies measured in this work.
Mass collection efficiency was measured by pre- and postcollection weighing of an aluminum foil placed on the collection plate. Measured mass collection efficiency for sucrose and stearic acid particles was ∼70% (having less than 5% standard deviation) for both types of particles, lower than respective overall number based collection efficiency, calculated from total number concentrations reported in . Possible reasons for this could include the presence of particles having sizes greater than 600 nm, not measured by the SMPS, or particle losses inside charging section due to image forces acting between charged particles and grounded surface, leading to wall deposition, which was visible in some experiments.
4.4. Advantages of a WESP for Soft Nanoparticle Collection
TEM images of stearic acid particles collected in dry ESP (, left) showed that sizes of particles collected on TEM grid (800–2000 nm) were significantly larger than sizes of upstream particles measured by SMPS (125 ± 8 nm). As particles were collected on dry plate directly, these soft particles formed aggregates and coalesced into larger particles of irregular shape. In case of collection into wet medium (, right), mean sizes of 50–150 nm were obtained, which compares reasonably with their measured mean mobility diameter of 125 ± 8 nm. However, some aggregated particles were seen. In addition to TEM imaging, mean hydrodynamic diameter measurements were made using DLS. Mean hydrodynamic diameter of 135 ± 3 nm of stearic acid particles suspended into sodium cholate solution, produced by continuous operation of WESP for 10 min was in reasonable agreement with particle mean mobility diameter (125 nm) from SMPS measurement.
5. CONCLUSIONS
A WESP was designed and evaluated in this work for wet collection of soft nanoparticles to overcome the different disadvantages (in terms of breaking and agglomeration of particles) of the current collectors. Three kinds of particles (PSL, sucrose and stearic acid) were used for performance measurement of the prototype, while it was found that the performance of the prototype was independent of type of particles as particles were charged by diffusion charging mechanism only. This characteristic of the current prototype will help to collect any kinds of particles with equal efficiency shown in result section. Measured collection efficiency was in reasonable agreement with theoretical estimates for particle diameter above 80 nm. However, for small particles (20–80 nm diameters) measured collection efficiency was significantly lower than the corresponding theoretical estimate from possible incomplete neutralization of pre-existing negative charge (from air-jet atomization) and attainment of positive saturation charge during the residence time provided. Other aerosol flow configurations should be evaluated to ensure complete positive charging of small nanoparticles in the charging section. Particles were collected with somewhat equal efficiency in WESP and dry ESP, because high electrical conductivity of salt water film used for wet collection. Performance tuning to improve collection efficiency is possible through control of applied voltages at the corona wire and collecting plate, charging time and electrical conductivity of liquid collection medium. Suspensions were obtained from continuous collection of lipid nanoparticles synthesized in an aerosol reactor, into a flowing surfactant solution in the WESP. Dry collection led to particles was highly aggregated into large, nonspherical shapes (in TEM images) of dimensions much larger than those of the original nanoparticle sizes based on mobility measurements. However, wet collection led to free and unaggregated particles in suspension, whose sizes measured by both TEM and DLS, were similar to the mean mobility diameter of the original aerosols. Therefore, WESP systems offer a useful collection method to obtain nanoparticle suspensions from aerosol collection directly into liquid media efficiently, without affecting their integrity, which can lend it to scale-up for operation with aerosol reactors.
uast_a_664295_sup_24584829.zip
Download Zip (30.9 KB)Acknowledgments
This work is performed under Grant No. SR/S3/CE/54/2008 of the Department of Science and Technology, Government of India, New Delhi. The authors also acknowledge Department of Chemical Engineering, Indian Institute of Technology Bombay and Centre for Research in Nanotechnology and Science, Indian Institute of Technology Bombay for granting fund also. Authors are also thankful to Mr. Amol Ashok Pawar, PhD Scholar, department of Chemical engineering, Indian Institute of Technology Bombay, Mumbai for using his setup for aerosol generation.
REFERENCES
- Adachi , M. , Kousaka , Y. and Okuyama , K. 1985 . Unipolar and Bipolar Diffusion Charging of Ultrafine Aerosol Particles . J. Aerosol Sci. , 16 : 109 – 123 .
- Almeida , A. J. , Runge , S. and Müller , R. H. 1997 . Peptide-Loaded Solid Lipid Nanoparticles (Sln): Influence of Production Parameters . Int. J. Pharm. , 149 : 255 – 265 .
- Berube , K. A. , Jones , T. P. , Williamson , B. J. , Winters , C. , Morgan , A. J. and Richards , R. J. 1999 . Physicochemical Characterisation of Diesel Exhaust Particles: Factors for Assessing Biological Activity . Atmos. Environ. , 33 : 1599 – 1614 .
- Bologa , A. , Paur , H. R. , Lehner , M. , Seifert , H. , Wäscher , T. and Woletz , K. 2009 . Collection of Fine Particles by Novel Wet Electrostatic Precipitator . IEEE T. Ind. Appl. , 45–46 : 2170 – 2176 .
- Bricard , J. and Pradel , J. 1966 . Aerosol Science , London : Academic Press .
- Deutsch , W. 1922 . Bewegung Und Ladung Der Elektrizitatstrager Im Zylinderkondensator . Ann. Phys. , 68 : 335 – 344 .
- Dixkens , J. and Fissan , H. 1999 . Development of an Electrostatic Precipitator for Off-Line Particle Analysis . Aerosol Sci. Technol. , 30 : 438 – 453 .
- Fernandez de la Mora , J. , Hering , S. V. , Rao , N. and McMurry , P. H. 1990 . Hypersonic Impaction of Ultrafine Particles . J. Aerosol Sci. , 21 : 169 – 187 .
- Fierz , M. , Kaegi , R. and Burtscher , H. 2007 . Theoretical and Experimental Evaluation of a Portable Electrostatic Tem Sampler . Aerosol Sci. Tech. , 41 : 520 – 528 .
- Flagan , R. C., and Seinfeld , J. H. 1988 . Fundamentals of Air Pollution Engineering , New Jersey, NJ. : Prentice Hall, Inc. .
- Friedlander , S. K. 2000 . Smoke, Dust, and Haze , New York : Oxford University Press .
- Fuchs , N. A. 1963 . On the Stationary Charge Distribution on Aerosol Particles in a Bipolar Ionic Atmosphere . Geofisica Pura e Applicata , 56 : 185 – 193 .
- Grinshpun , S. A. , Willeke , K. , Ulevicius , V. , Juozaitis , A. , Terzieva , S. Donnelly , J. 1997 . Effect of Impaction, Bounce and Reaerosolization on the Collection Efficiency of Impingers . Aerosol Sci. Technol. , 26 : 326 – 342 .
- Hinds , W. C. 1982 . Aerosol Technology: Properties, Behavior, and Measurement of Airborne Particles , John Willy & Sons, New York .
- Hogan , C. J. , Kettleson , E. M. , Lee , M. H. , Ramaswami , B. , Angenent , L. T. and Biswas , P. 2005 . Sampling Methodologies and Dosage Assessment Techniques for Submicrometre and Ultrafine Virus Aerosol Particles . J. Appl. Microbiol. , 99 : 1422 – 1434 .
- Hussin , A. , Scheibel , H. G. , Becker , K. H. and Porstendorfer , J. 1983 . Bipolar Diffusion Charging of Aerosol Particles-I: Experimental Results within the Diameter Range 4–30 Nm . J. Aerosol Sci. , 14 : 671 – 677 .
- Kennard , E. 1938 . Kinetic Theory of Gases , New York : McGraw-Hill .
- Li , M. H., and Christofides , P. D. 2006 . Collection Efficiency of Nanosize Particles in a Two-Stage Electrostatic Precipitator . Ind. Eng. Chem. Res. , 45 : 8484 – 8491 .
- Lin , X. 2000 . Survival of Airborne Microorganisms During Swirling Aerosol Collection . Aerosol Sci. Technol. , 32 : 184 – 196 .
- Liu , B. Y. H., and Pui , D. Y. H. 1977 . Unipolar Diffusion Charging of Aerosols in Continuum Regime . J. Colloid. Interf. Sci. , 58 : 142 – 149 .
- Mainelis , G. , Adhikari , A. , Willeke , K. , Lee , S. A. , Reponen , T. and Grinshpun , S. A. 2002a . Collection of Airborne Microorganisms by a New Electrostatic Precipitator . J. Aerosol Sci. , 33 : 1417 – 1432 .
- Mainelis , G. , Grinshpun , S. A. , Willeke , K. , Reponen , T. , Ulevicius , V. and Hintz , P. J. 1999 . Collection of Airborne Microorganisms by Electrostatic Precipitation . Aerosol Sci. Tech. , 30 : 127 – 144 .
- Mainelis , G. , Willeke , K. , Adhikari , A. , Reponen , T. and Grinshpun , S. A. 2002b . Design and Collection Efficiency of a New Electrostatic Precipitator for Bioaerosol Collection . Aerosol Sci. Technol. , 36 : 1073 – 1085 .
- Miljevic , B. , Modini , R. L. , Bottle , S. E. and Ristovski , Z. D. 2009 . On the Efficiency of Impingers with Fritted Nozzle Tip for Collection of Ultrafine Particles . Atmos. Environ. , 43 : 1372 – 1376 .
- Muller , R. H. , Jacobs , C. and Kayser , O. 2001 . Nanosuspensions as Particulate Drug Formulations in Therapy: Rationale for Development and What We Can Expect for the Future . Adv. Drug Deliver. Rev. , 47 : 3 – 19 .
- Pawar , A. A., and Venkataraman , C. 2011 . Droplet-Phase Synthesis of Nanoparticle Aerosol Lipid Matrices with Controlled Properties . Aerosol Sci. Tech. , 45 : 811 – 820 .
- Steffens , J. , and Coury , J. R. 2007 . Collection Efficiency of Fiber Filters Operating on the Removal of Nano-Sized Aerosol Particles. Ii. Heterogeneous Fibers . Sep. Purif. Technol. , 58 : 106 – 112 .
- Tsai , C. J. , Lin , J. S. , Deshpande , C. G. and Liu , L. C. 2006 . Electrostatic Charge Measurement and Charge Neutralization of Fine Aerosol Particles During the Generation Process . Part. Part. Syst. Charact. , 22 : 293 – 298 .
- Utsunomiya , S. , Jensen , K. A. , Keeler , G. J. and Ewing , R. C. 2004 . Direct Identification of Trace Metals in Fine and Ultrafine Particles in the Detroit Urban Atmosphere . Environ. Sci. Technol. , 38 : 2289 – 2297 .
- Wei , Z. C. , Rosario , R. C. and Montoya , L. D. 2010 . Collection Efficiency of a Midget Impinger for Nanoparticles in the Range of 3-100 Nm . Atmos. Environ. , 44 : 872 – 876 .
- Wen , J. , and Wexler , A. S. 2007 . Thermophoretic Sampler and Its Application in Ultrafine Particle Collection . Aerosol Sci. Technol. , 41 : 624 – 629 .
- Westesen , K. , Bunjes , H. and Koch , M. H. J. 1997 . Physicochemical Characterization of Lipid Nanoparticles and Evaluation of Their Drug Loading Capacity and Sustained Release Potential . J. Control. Release , 48 : 223 – 236 .
- White , H. J. 1963 . Industrial Electrostatic Precipitation , Addison Wesley Publishing Company, Massachusetts .
- Wiedensohler , A. , and Fissan , H. J. 1991 . Bipolar Charge Distrubutions of Aerosol Particles in High-Purity Argon and Nitrogen . Aerosol Sci. Technol. , 14 : 358 – 364 .
- Zhuang , Y. , Jin Kim , Y. , Gyu Lee , T. and Biswas , P. 2000 . Experimental and Theoretical Studies of Ultra-Fine Particle Behavior in Electrostatic Precipitators . J. Electrostat. , 48 : 245 – 260 .