Abstract
The counting efficiencies of two TSI 3790 Condensation Particle Counters (CPCs) were investigated experimentally for graphite, poly-(alpha)-olefin (PAO), tetradecane (C14), and hexadecane (C16) particles at saturator-to-condenser temperature differences spanning from 5.6°C to 11.3°C. The efficiencies determined with PAO, C14, and C16 particles were broadly similar, while tests with graphite particles resulted in systematically lower counting efficiencies. The differences between PAO and graphite particles were reduced at elevated temperature differences, i.e., as the saturation ratios inside the condenser increased. The possibility to predict measured counting efficiencies by heterogeneous nucleation theory was also assessed. The results for PAO, C14, and C16 were representative of perfectly wettable particles, while graphite data could only be reproduced with a contact angle of 6–12°, for all temperatures examined. Line tension fits revealed a linear correlation with the graphite mobility diameter for all operating temperatures (5 × 10−11 N at 15 nm to 4 × 10−10 N at 70 nm). This could actually indicate that the mobility diameter underestimates the contact line for these complex geometry graphite aggregates. An examination of the calculated activation regions inside the condenser indicated that the upper part of the counting efficiency curve (>50%) is very sensitive to flow and temperature nonidealities. This observation is in quantitative agreement with systematic deviations observed between theoretical predictions and experimental data. Numerical calculations for a range of working fluids suggested that for a given affinity of the calibration particle to the examined vapors (i.e., for a finite contact angle), the benefit from shifting to a fluid alternative to butanol is limited. Further investigations on the reduction of the material dependence should focus on the identification of working fluids exhibiting greater affinity for different particle materials (e.g., lower contact angle).
Copyright 2012 American Association for Aerosol Research
INTRODUCTION
Diesel exhaust particulate matter (PM) is a very complex physicochemical mixture that has been associated with numerous adverse health effects (NIOSH Citation1988; IARC Citation1989; California EPA Citation1998; EPA Citation2009). Consequently, the mass of PM emitted by diesel light-duty vehicles and heavy-duty engines has been subject to continuously more stringent worldwide regulation. Recently, a particle number limit was additionally introduced in the European legislation that effectively limits PM emissions to a level well below the sensitivity of the conventional gravimetric measurement procedure, effectively enforcing the installation of a wall-flow particulate filter (DPF) in all diesel passenger cars (Commission Regulation 692/Citation2008). A particle number limit is also foreseen to be introduced for the certification of diesel heavy-duty engines (Commission Regulation 715/Citation2007) and gasoline light-duty vehicles (Commission Regulation 692/Citation2008).
The legislated particle number (PN) measurement procedure is largely based on the recommendations of the Particulate Measurement Programme (PMP) (Giechaskiel et al. Citation2012). It is designed on the requirement to remove the volatile fraction of PM that is not controlled by the DPF systems. It requires dilution and thermal treatment of the exhaust aerosol (at 300–400°C) to evaporate volatile species and to decrease their partial vapor pressures to levels that would not initiate their nucleation.
As an additional safeguard, the regulation requires that the number concentration of the thermally treated particles be measured with a condensation particle counter (CPC) having a 50% (±12%) detection efficiency (d50 ) at 23 nm (±1 nm) and more than 90% counting efficiency at 41 nm (±1 nm). In that respect, the CPC would be able to detect the smallest soot particles whose primary size is reported to lie in the 17–32 nm range (Wentzel et al. Citation2003; Mathis et al. Citation2005), while at the same time volatile particles (e.g., formed by homogeneous nucleation) of diameters below this size range would not contribute to the particle counts.
The relatively large cutoff sizeFootnote 1 of PMP-compliant CPCs requires their operation at low saturation ratios, realized through the use of small temperature differences between the condenser and the saturator (nominal 9°C in TSI 3010D, 6.6°C in TSI 3790, and 9°C in Grimm 5.430). Calibration experiments performed on PMP-compliant CPCs identified a strong dependence of the counting efficiency curves on the calibration aerosol employed for all commercially available instruments (Giechaskiel et al. Citation2009; Wang et al. Citation2010).
Subsequent numerical modeling work by Giechaskiel et al. (Citation2011) indicated that the observed strong material dependence of the TSI 3790 CPC at such low saturation ratios was in agreement with predictions of classical heterogeneous nucleation theory (Fletcher Citation1958). Poly-(alpha)-olefin (PAO) particles were found to be perfectly wettable to butanol at the nominal operating temperatures of the instrument, while CAST and diesel soot particles showed a lower affinity for butanol that could be reproduced assuming a contact angle in the range of 5–10°. Even lower counting efficiencies were observed for sodium chloride (contact angle 15–20°) and thermally treated tetracontane (∼25°) particles.
The numerical model, though, failed to capture the shape of the measured counting efficiency curves, especially at the upper part (above 50% counting efficiencies). This was particularly evident for PAO results in the 40–90% regime that were found to lie systematically above the theoretically predicted efficiencies for perfectly wettable particles. The discrepancy was attributed to contamination of the PAO particles by ethanol or water due to the generation method employed (electrospray).
This follow-up work provides additional insight onto the reason(s) for the observed differences between predictions of the heterogeneous nucleation model and experimental results. To this end, dedicated calibration experiments were performed on two TSI 3790 CPCs by using PAO, graphite, tetradecane (C14), and hexadecane (C16) particles. In addition, the numerical model was amended to investigate the effects of line tension and condensational growth, and to quantify the effect of flow and temperature nonidealities on the counting efficiency curves. The model was also applied to predict the operating conditions that would be required for working fluids alternative to butanol, and to identify the most suitable of them.
EXPERIMENTAL
Two TSI 3790 CPCs (CPC#1 and CPC#2) were calibrated in the study. Like all currently available PMP-compliant CPCs, this model uses butanol as the working fluid. The sampled aerosol first passes through eight cylindrical channels inside a wick saturated in butanol at 38.3°C and then enters eight cylindrical condensers that are maintained at the same lower temperature (Crooks Citation2007). One unit (CPC#1) operated at a nominal (according to the manufacturer certificate) condenser temperature of 31.7°C, while the other (CPC#2) at 31.3°C. CPC#1 was also tested at condenser temperatures of 27°C, 29°C, and 32.7°C.
A TSI 3025A CPC was employed as a reference against which CPC#1 and CPC#2 were calibrated. The reference CPC was shipped to TSI for maintenance 6 months before the measurement campaign and it was hardly used in between. Its performance was also checked before the tests in dedicated calibration experiments against a TSI 3068B electrometer by using silver particles (Mamakos et al. Citation2011).
Both CPCs were calibrated against graphite and PAO particles, while CPC#2 was also tested with tetradecane (C14) and hexadecane (C16). Graphite particles were produced by spark discharge (DNP3000 from PALAS GmbH, operating at 3 lpm N2, 3 lpm air, 2 mA current, and medium energy), while PAO, C14, and C16 particles were generated by evaporation–condensation in a generator made in-house (Mamakos et al. Citation2011). The generators operated on purified (HEPA filtered and dehumidified) air and bottled nitrogen to minimize the risk of particle contamination. The evaporation–condensation generator was thoroughly cleaned between changes of the working liquid (PAO, C14, and C16), by extended operation at elevated temperatures and the use of nitrogen flow to purge any condensed residuals.
A schematic diagram of the experimental setup is illustrated in . The polydisperse aerosols produced in the generators (geometric standard deviation of ∼1.65 with a peak at ∼50 nm for DNP3000, and geometric standard deviation of ∼1.5 with a peak at ∼60 nm for the evaporation–condensation generator) were first diluted in a single dilution bridge to control the concentration of the calibration aerosol and then passed through a TSI 1035900 impactor (equipped with a 0.071 cm nozzle) to remove particles of aerodynamic diameter larger than approximately 1 μm. The particles were then size-classified in a tandem differential mobility analyzer (TDMA) setup to minimize interference from multiply charged particles (the peak doubly charged fraction was estimated to be lower than 3%). The TDMA consisted of a Grimm 5.5-900 Differential Mobility Analyzer (DMA) connected in series with a TSI 3081 DMA. The first DMA (Grimm 5.5-900) was equipped with a 0.1-mCi 241Am neutralizer (Grimm 5.522) and operated at a sheath flowrate of 3 lpm. The second DMA (TSI 3081) was equipped with a 10-mCi 85Kr neutralizer (constructed by Eckert and Ziegler GmbH) and operated at a sheath flowrate of 10 lpm. The sample flowrate in this TDMA setup was set to 1 lpm, necessitating the removal of the internal impactor of the Grimm 5.522 DMA that would have increased significantly the backpressure (as it was designed for a sample flowrate of 0.3 lpm). All flows were regularly checked with a bubble flowmeter (Gillian Gilibrator 2). The two DMAs were calibrated before the measurement campaign against polystyrene spheres having a traceable diameter of 498 ± 5 nm (Thermo Scientific 3500A) (Mamakos et al. Citation2011).
The aerosol classified in the TDMA was mixed with purified make-up air supplied by a Mass Flow Controller (MFC) set at 1.5 lpm. The two streams were mixed in a 70 cm long, conductive silicon tube (that was found to allow for sufficient mixing) (Mamakos et al. Citation2011) and then split again into two streams through a metallic Y-type connector. The concentration of the classified particles was then measured with the CPC under calibration sampling in parallel with the reference TSI 3025A CPC. Conductive silicon tubes of different length (15 cm for the TSI 3025A and 10 cm for the TSI 3790s) were employed for the connection of the two CPCs to the Y-type connector, to allow for similar diffusional losses at the different operating flowrates of the instruments (1.5 lpm for 3025A and 1 lpm for 3790).
MODEL DESCRIPTION
Numerical calculations were performed to get insight onto the reasons for the experimentally observed material dependence of the counting efficiencies. Their basis was the numerical model developed by Giechaskiel et al. (Citation2011), further modified by Mamakos et al. (Citation2011). The original model calculates CPC counting efficiencies by assuming that seed-particle activation occurs via heterogeneous nucleation (Fletcher Citation1958). The required thermodynamic quantities, butanol saturation ratio and fluid temperature, are calculated from the analytical solution of two decoupled heat and mass transfer problems in axisymmetric geometry (Stolzenburg and McMurry Citation1991; Housiadas et al. Citation1999). Herein, we present the main modifications: an extended description of the original model and its modifications can be found in the online supplemental information and the original references.
Heat and mass transfer inside the CPC condenser were calculated under ideal (base model) and nonideal flow and temperature conditions. The flow in the condenser was taken to be either fully developed laminar (parabolic Hagen–Poiseuille, as in the original model) or plug, chosen to be an example of an extreme flow nonideality (i.e., exhibiting significant departure from parabolic flow). Temperature nonidealities (i.e., nonuniform temperature at the condenser inlet) were modeled by assuming a 2°C temperature difference (induced by a constant temperature gradient) between the inlet centerline and wall temperatures (Housiadas et al. Citation2000).
The original model was extended to consider the effect of the line tension that forms at the circumference of the spherical cluster cap where the three phases (particle seed, cluster, and vapor) meet (Lazaridis Citation1993). The line tension modifies explicitly the free energy of formation of the critical cluster and the contact angle (and, consequently, the multiplicative geometric factor in the cluster free energy of formation).
We also performed condensational growth calculations (Hinds Citation1999) to estimate the size of activated particles at the condenser outlet and the relative importance of particle growth and evaporation. For simplicity, particle activation was assumed to occur at the Kelvin ratio, and latent heat release and vapor depletion were considered negligible to modify the local saturation ratio. Nevertheless, the droplet temperature was allowed to change due to vapor condensation and heat conduction.
Diffusional losses in the condenser were ignored as they had been found negligible (Giechaskiel et al. Citation2011). The numerical calculations were performed for one of the eight identical cylinders of the 3790 condenser. The cylinder (53 mm length and 2.3 mm radius) was discretized in a 500 × 500 two-dimensional cylindrical grid.
RESULTS AND DISCUSSION
Experimentally Determined Counting Efficiencies
compares measured counting efficiencies of CPC#1 for graphite and PAO particles. Results are shown for the nominal operating temperatures, as well as for three other condenser temperatures.
FIG. 2 Measured counting efficiencies of CPC#1 for PAO (squares) and graphite (circles) at four different temperature settings (the efficiencies at the nominal operating temperatures are shown on the top right panel). Symbols of different shadings correspond to test repetitions. The calibration points by TSI are also shown on the top right panel as filled rhombi.
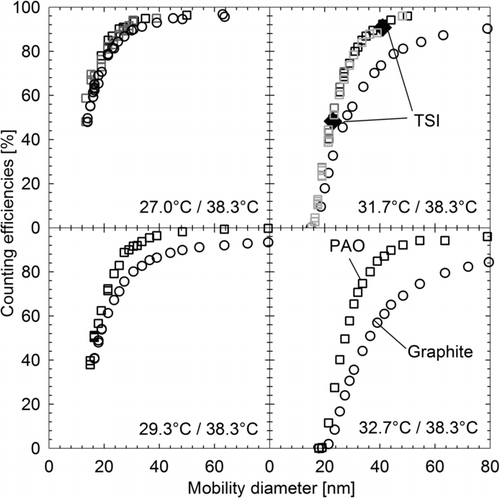
The counting efficiency for 23 nm PAO particles at the nominal operating temperatures ranged between 49% and 54% over three test repetitions, averaging at 52%. These results verify the calibration certificate by the manufacturer that is issued from tests with PAO particles. The corresponding counting efficiency for graphite, however, was only 33%, falling outside the limit specified in the European regulation (minimum efficiency of 38%). Similarly, the CPC complied with the >90% requirement for 41 nm particles only for PAO (92%) and not for graphite (74%).
Wang et al. (Citation2010) also reported reduced counting efficiencies of the same magnitude (reduced by 10–19% at 23 nm and 10–15% at 41 nm) for combustion aerosol standard generator (CAST) (Jing Citation1999) soot, oxidized silver, and polystyrene particles with respect to PAO. Moreover, Giechaskiel et al. (Citation2009) also observed similar differences between PAO and diesel soot (15% at 23 nm) or CAST soot (18% at 23 nm), at slightly elevated temperature differences (30.8°C and 38.3°C). Validation of many 3790 CPCs gave an average difference between PAO and CAST of 15% for 23 nm and 6% for 41 nm (Giechaskiel and Bergmann Citation2011).
The experimental data collected in the present study at different operating temperatures () suggest that the effect of calibration material becomes more pronounced as the temperature difference between the saturator and the condenser decreases. This is in line with heterogeneous nucleation theory predictions for particles exhibiting different affinity for butanol, as shown by Giechaskiel et al. (Citation2011; their ).
FIG. 3 Left panels: measured counting efficiencies of two TSI 3790 CPCs set at different condenser temperatures (31.7°C versus 31.3°C) during manufacturer calibration. Different symbols indicate different calibration materials (PAO, C14, and C16), while different shadings correspond to repetitions with the same material. The numerically calculated counting efficiency curves for perfectly wettable particles at the two set temperatures are also shown. Right panels: comparison of measured counting efficiencies of CPC#1 for PAO (upper panel) and graphite (lower panel) with model predictions for contact angles of 0° (upper panel) and 10°C (lower panel). Calculated counting efficiencies are shown for both plug and fully developed laminar flow (parabolic velocity profile), as well as for a constant temperature gradient at the condenser inlet (assuming that the temperature at the centerline is 2°C higher than the condenser wall temperature).
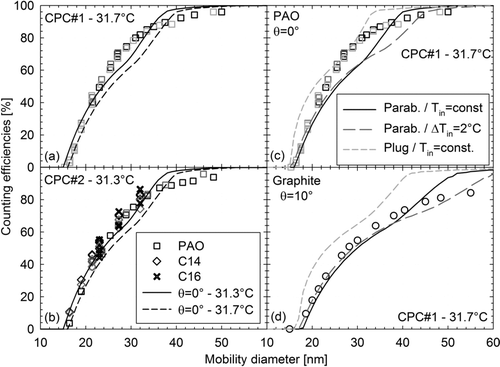
Comparison of Experimental Results with the Base Model
compares measured counting efficiencies with PAO for CPC#1 at its nominal operating temperatures to theoretical predictions with the base model for perfectly wettable particles. While the model accurately predicts particle activation at counting efficiencies approximately <50%, it fails to capture the shape of the counting efficiency curve. The model yields systematically lower efficiencies over the upper part of the curve (50–80%), and it predicts that peak counting efficiencies (>90%) occur at smaller sizes. The perfect wetting limit corresponds to the minimum activation free energy barrier; it cannot be further reduced by considering the effects of line tension as the cosine of the contact angle cannot exceed unity (Equation (S6b)). In that respect, the experimentally observed higher counting efficiencies could reflect either (a) physicochemical interactions between PAO and butanol (i.e., partial solubility of the butanol in PAO or contamination of PAO droplets with impurities, as hypothesized by Giechaskiel et al. Citation2011) or (b) nonidealities that are not captured by the numerical model (e.g., different from assumed flow and temperature profiles). The possibility that the aforementioned mechanisms could explain the observed discrepancies is investigated below.
Steep Section of the Counting Efficiency Curve
shows the measured counting efficiencies of the second TSI 3790 CPC (CPC#2) for PAO, C14, and C16 particles. The counting efficiency curve was broadly similar for these three materials. The average counting efficiencies at 23 nm were 52% for C14, 49% for C16, and 48% for PAO, with the relative differences lying well within experimental uncertainty (3–5% in terms of standard deviation). This suggests that the elevated PAO efficiencies reported in previous studies (Giechaskiel et al. Citation2009, Citation2011; Wang et al. Citation2010) did not originate from physicochemical interactions specific to butanol and PAO (e.g., partial solubility) that could have enhanced its activation in the CPC, since results with the two alkanes (C14 and C16) were found to be similar to PAO data. Particle contamination is also highly unlikely in the evaporation–condensation generator employed in the present study.
On the other hand, the different set temperature points of the two CPCs (both operated at a saturator temperature of 38.3°C, but at condenser temperatures 31.7°C for CPC#1 and 31.3°C for CPC#2) are indicative of instrumental imperfections. Despite the different set temperatures, the measured counting efficiencies agreed very well, ranging between 46.5% and 49% at 23 nm for CPC#2 when the average counting efficiency at the same size for CPC#1 was 52%. Yet, numerical predictions for perfectly wettable particles (also plotted in and ) suggest that even a temperature difference of 0.4°C is sufficient to reduce the counting efficiency at 23 nm from 48% (31.3°C) to 41% (31.7°C). Similar and even higher (up to 0.9°C) differences in the set temperatures of different TSI 3790 models were reported by Giechaskiel and Bergmann (Citation2011). Such manufacturing imperfections, whatever their origin (one might speculate inaccurate thermocouples), need to be taken into account when assessing the numerical model.
Temperature and flow nonidealities inside the condenser can also affect the shape of the counting efficiency. For example, and illustrate the effect of (a) a constant temperature gradient and (b) a uniform velocity profile at the inlet of the condenser on the counting efficiency curves of CPC#1. Results are shown for contact angles of 0° () and 10° () that were found to describe well the onset of the measured counting efficiencies for PAO and graphite, respectively, with the base model. The calculations suggest that temperature nonidealities mostly affect the upper part of the curve (>80% efficiency), while the plug-flow assumption shifts the entire curve toward smaller sizes. Interestingly, the calculated efficiency curves encapsulate most of the experimental data. Yet, a precise quantification of such effects requires an accurate determination of the temperature and velocity profiles (e.g., through computational fluid dynamics calculations), a study beyond the scope of the present investigations. The simplified calculations performed here, though, suggest that the observed discrepancies might reflect deviations from the assumed uniform inlet temperature and parabolic velocity in the condenser.
In the perfect wetting limit, the activation probability depends solely on the kinetic prefactor (Equations (S2–S4)), the determination of which is accurate only to within a couple of orders of magnitude. Inaccuracies in its calculation could affect calculated counting efficiencies as well. However, a 1-nm shift of the 50% counting efficiency toward smaller sizes could only be reproduced if the kinetic prefactor is underestimated by 12 orders of magnitude, a highly unlikely underestimation.
Peak Counting Efficiencies
Another observed systematic deviation of experimental data from calculated results pertains to the size at which the counting efficiency reaches its peak value. The measured counting efficiencies systematically peaked at larger sizes than model predictions, especially for graphite (). To a certain extent, this could be explained by a nonuniform temperature profile at the inlet of the condenser as shown in and .
FIG. 4 Activation regions (bottom panel) of 17, 23, and 41 nm perfectly wettable particles at condenser temperatures of 31.3°C (black lines) and 31.7°C (gray lines). Symbols illustrate the expected width of the activation regions based on the measured counting efficiencies with CPC#1 and CPC#2 for PAO (the axial position of the symbols is arbitrarily selected). The top panel illustrates the counting efficiency as a function of the width of the activation region assuming fully developed laminar flow.
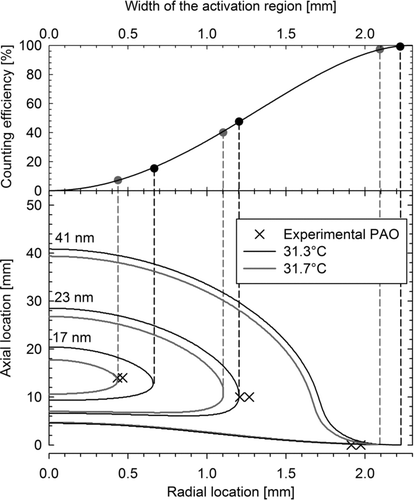
However, and more importantly, this part of the efficiency curve (>90%) also reflects the activation and subsequent growth of particles near the condenser wall. shows the activation regions of particles of different sizes inside the condenser for perfectly wettable particles at the nominal operating temperatures of the two CPCs. Provided that all activated particles grow to sufficiently large sizes, all particles entering the activation region would be detected by the optical sensor. Then, in accordance to Equation (S13), the counting efficiency for a given particle size solely depends on the fraction of the condenser cross section that is covered by the activation region and the velocity profile within this region. For the axisymmetric geometry of the condenser, the cross section of the activation region is a circle characterized by its radius, referred to as the activation width in this work. Stated differently, for a given velocity profile and a given particle size, a one-to-one correspondence between the width of the activation region and the counting efficiency for that same particle size exists. This correspondence is shown in the upper panel of (for fully developed laminar flow).
One could also invert the previous argument and calculate the width of the activation region inside the condenser from the measured counting efficiencies. The so-calculated widths for the experimental data for PAO are also specified by crosses in (the two points per particle diameter correspond to the maximum and minimum counting efficiencies determined by the two CPCs). A relatively good agreement is observed only for 17 nm and 23 nm particles, in that the range of the activation widths intersects the simulated curves at the two condenser temperature. However, it appears that the 41-nm particles entering the condenser at a distance smaller than 0.3 mm from the wall of the condenser are not detected. The activation region near the wall (0.3–0.07 mm from the wall) becomes very narrow and approaches the inlet (<0.2 mm from the inlet). The close distance to the entrance makes particle activation very sensitive to temperature nonidealities, as already shown in and .
In the above analysis, it is assumed that (a) all activated particles grow and (b) the particles at the exit of the condenser are large enough to be optically detected. The first assumption requires that condensational growth proceed faster than any increase of the critical radius as the activated particle moves through regions of lower saturation ratios. The second assumption is valid if the size of the grown droplet is larger than circa 0.5 μm (Zhang and Liu Citation1990).
FIG. 5 Condensational growth of 17 nm (solid gray lines) and 41 nm (solid black lines) at the outermost radius of the corresponding activation regions. Dotted lines illustrate the Kelvin diameter at the conditions prevailing along the axial motion of the particle.
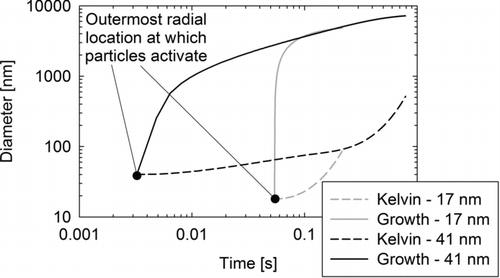
In order to investigate the validity of the aforementioned assumptions, condensational growth calculations were performed based on Kelvin activation theory. summarizes these results for particles sizes of 17 nm and 41 nm entering at the outermost radial location at which these particles activate (limit of the activation region). This corresponds to a worst-case scenario, in that the critical saturation ratio coincides with the maximum saturation ratio at that radial location. At all axial locations following this activation point, the Kelvin diameter is larger and if growth is not fast enough, the cluster may evaporate. The calculations suggest though that condensational growth is rapid enough to ensure that evaporation will not occur. Furthermore, all activated particles grow to supermicron sizes (>4 μm) (Mamakos et al. Citation2011) at the exit of the condenser. For comparison, the Kelvin diameter at the outlet of the condenser was calculated to be less than 1 μm across the radial locations that correspond up to 98% of the counting efficiency (Mamakos et al. Citation2011).
The calculations did not consider changes in the saturation ratios in the vicinity of activated particles associated with butanol depletion or latent heat release. The maximum reduction in the local saturation ratio was estimated to be 3.5% due to the consumption of butanol and 10% due to the increase of the carrier gas temperature. (Mamakos et al. Citation2011). Yet, the average distance between particles ( for a maximum inlet concentration of 10,000#/cm3) is at least two orders of magnitude larger than the diameter of grown droplets; therefore, local changes of the saturation ratio (which according to heat transfer calculations should be confined to within a few droplet diameters from the activated particle) are not expected to affect the activation of neighboring particles.
These calculations suggest that all activated particles grow to sufficiently large sizes at the outlet of the condenser. Yet, the model does not consider the dead volume between the condenser (or, more accurately, the eight parallel condensers) and the optics section. A prolonged residence time in these regions may initiate shrinkage of the grown droplets, especially those lying close to the walls where butanol vapors would be lost by diffusion. However, as the exact geometry of the CPC is not known, it is not possible to assess or quantify such effects.
Model Fitting
Heterogeneous nucleation theory allows for the consideration of the contact angle and the line tension, both of which affect nucleation rates and, thus, counting efficiencies. Thus, it is possible to determine them by fitting each one (separately) to the measured counting efficiencies, such that model predictions agree with experimental data for each particle size and operating temperatures.
Contact Angle
summarizes the results of such a fitting procedure for the contact angles, based on the experimental data from CPC#1. Since the actual operating temperatures may differ from the set values (as discussed in the previous section), calculations were also performed assuming an ±0.2°C uncertainty, whose effect is shown by error bars in .
FIG. 6 Calculated contact angles as a function of counting efficiency for graphite (circles) and PAO (squares) particles, based on the experimental data from CPC#1. Different shadings correspond to different set condenser temperatures (saturator temperature was maintained at 38.3°C in all tests). The error bars illustrate the effect of a ±0.2°C uncertainty in the set temperatures on the calculated contact angles.
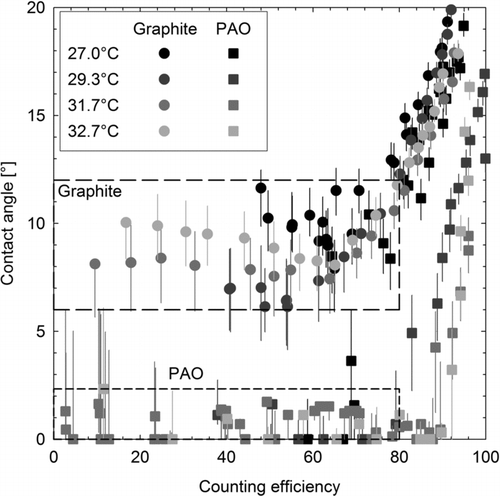
The analysis in the previous section suggests that the most plausible cause of the deviations of model predictions from experimental data is temperature and/or flow nonidealities, the effect of which is more pronounced at the upper part of the counting efficiency curve. This is better illustrated in where results are plotted against measured counting efficiencies. Two distinct regions can be identified. Efficiencies below circa 80% can be described reasonably well by a relatively constant contact angle (±2°) for each particle material at each operating temperature. Above this region (>80%), the calculated contact angles increase abruptly with increasing counting efficiency. This arises because the measured counting efficiencies reach the peak value (100%) at a much larger size than model predictions (). Hence, if the model were to reproduce the experimental data, a gradually higher free energy barrier would be required with increasing particle size.
The sub 80% efficiency regime clearly illustrates the different behavior of graphite and PAO particles. The efficiencies determined for graphite particles can be reproduced with a contact angle of 6–12°, while those for PAO particles suggest a contact angle smaller than 2.5°. It needs to be stressed that some zero contact angles do not correspond to a perfect fit since some PAO experimental data lie above the maximum counting efficiencies predicted by the model ( and ).
Focusing on the graphite results, no systematic dependence of the calculated contact angles on the temperature difference could be identified. The calculated contact angles at 50% ± 5% counting efficiency were 9.8–11.6° at 11.3°C (T cond = 27°C), 6.7–7.0° at 9°C (T cond = 29.3°C), 7.5–7.9° at 6.6°C (T cond = 31.7°C), and 8.4–9.3° at 5.6°C (T cond = 32.7°C). Hence, within the variability of the contact angles (3.2–5°) as determined to reproduce the counting efficiencies up to 80% at a given operating condition, a variability that is further extended if the temperature uncertainty of ±0.2°C is included (1.5–4.5°), no clear dependence of the fitted contact angles on the condenser temperature could be identified.
FIG. 7 Calculated line tension as a function of the counting efficiency for graphite (circles) and PAO (squares) particles, based on the experimental data from CPC#1. Different shadings correspond to different set condenser temperatures (saturator temperature was maintained at 38.3°C in all tests). The error bars illustrate the effect of a ±0.2°C uncertainty in the set temperatures on the calculated line tensions. The contact angle was assumed to be 0.5°, as a zero value would result in an infinite energy barrier (Equation (S7) substituted into Equation (S6)). The inset shows the calculated line tensions plotted against the mobility diameter.
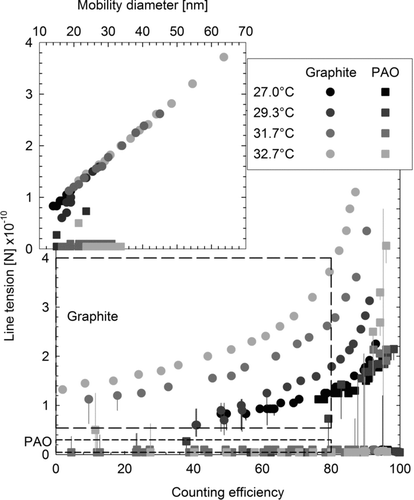
Line Tension
summarizes the fitted line tensions on the experimental data collected with CPC#1. The calculations were performed for a contact angle of 0.5°, which per se has insignificant effect on the calculated counting efficiencies compared to the baseline case of a contact angle of 0°. The use of a very small, yet nonzero, contact angle is required because the sine of the contact angle appears in a denominator of the equations describing the cluster free energy of formation for nonzero line tension (evident by substituting Equation (S7) to Equation (S6)).
The distinctly different performance of the CPC in detecting PAO and graphite particles is also evident. The majority of the experimental data with PAO could be represented by a line tension value of less than 5 × 10−12 N. On the other hand, the line tensions that better described the graphite results spanned from 5 × 10−11 N to 4 × 10−10 N in most cases. Graphite results also showed a dependence on the operating temperature difference, with the calculated line tension value increasing as the condenser temperature increased.
FIG. 8 Numerically calculated counting efficiencies at contact angles of 0° (left-hand panel) and 10° (right-hand panel), for line tensions of 0 N, ±10–12 N and ±2 × 10–12 N.
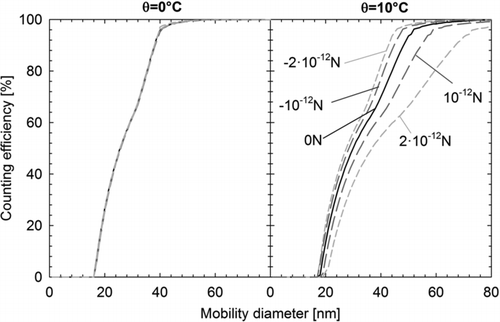
Interestingly, when the fitted line tensions are plotted against the mobility diameter (inset in ), the graphite results appear to fall onto a single straight line, at all four set temperatures investigated. Such an apparent increase of the line tension with increasing mobility size may reflect a size-dependent underprediction of the contact line length (circumference of the critical cluster, πdp sinφ, Equation (S6a)), since the critical free energy of formation depends on both these quantities (i.e., the line tension and the circumference). The underestimation of the contact line length may originate from a failure of the base heterogeneous nucleation model, which assumes spherical particle seeds, to model accurately the free energy barrier of graphite aggregates, whose morphology in this treatment is solely described by their mobility equivalent diameter.
The magnitude of the calculated line tensions is in agreement with reported values (10−13 to 10−10 N) (Hienola et al. Citation2007). Most studies suggest that the line tension is negative, i.e., its consideration enhances nucleation rates. Yet, Wang et al. (Citation2001), in their experiments of n-octane and 1-octene droplets forming onto hexadecyltrichlorosilane-coated silicon wafer substrate, found that the sign of the line tension becomes positive at conditions close to the wetting transition with the sign change accompanied by a decrease of the contact angles. The interpretation of the line tension results is complicated because the change of the counting efficiency due to a finite value of a line tension becomes higher at increasing contact angles. For example, as shown in , a line tension of 2 × 10−12 N has practically no effect on the counting efficiency curve for a contact angle of 0.5° but can shift the 50% counting efficiency by approximately 7 nm in the case of 10° contact angle. This synergetic effect of contact angle and line tension renders difficult the determination of any one of them solely from measured CPC counting efficiencies.
TABLE 1 Calculated saturator temperature (T in) required to detect 50% of 23 nm perfectly wettable particles entering the condenser at 31.3°C (d50 (θ = 0°) = 50%) and difference in the 50% cutoff size for 0° and 10° contact angle, for different working fluids. Also shown are the average vapor pressure (P vapor), temperature (T), liquid density (ρ l), surface tension (σ lg), saturation ratio (S), and critical radius (r*) across the envelope of the activation region of perfectly wettable 23 nm particles, as well as the maximum and minimum droplet size of the activated particles grown via condensation at the exit of the condenser
Working Fluid Investigations
The strong material dependence observed in the counting efficiencies of PMP-compliant CPCs raises the question whether a different working fluid would improve their performance. There are two aspects to this problem. The first pertains to the affinity of the particle material for the working fluid. A suitable working fluid would form clusters exhibiting low interfacial free energy barriers, namely, it would exhibit lower contact angles and line tensions than butanol for a given aerosol material type.
The second aspect pertains to the physicochemical properties of the working fluid that should ideally minimize the effect of a finite contact angle and/or line tension on the counting efficiency. In order to investigate whether such suitable physicochemical properties, or combinations of them, exist numerical calculations were performed for a range of liquids that have been considered for application in CPCs (Magnusson et al. Citation2003). The list includes a range of alcohols, fatty acids, chlorinated hydrocarbons, polyols, aromatics, and n-alkanes. The necessary thermophysical properties were taken from Magnusson et al. (Citation2003).
To simplify the analysis, the effect of line tension was disregarded. The calculations proceeded as follows: First, the operating temperatures that would result in a 50% counting efficiency of perfectly wettable particles (contact angle 0°) at 23 nm were determined. Since a desirable design feature is prevention of water condensation inside the condenser, the condenser temperature was fixed at 31.3°C (Crooks Citation2007). Subsequently, the particle diameter that would result (at these operating temperatures) in a 50% counting efficiency for a contact angle of 10° was determined. The results of these calculations are summarized in .
The first thing to notice is that limited room for improvements exists, with the best performing working fluid (hexane) showing a 3.1-nm difference in the 50% efficiency particle size (d50 ) for contact angles of 10° and 0°, when the corresponding value for butanol is 3.6 nm. The best performing working fluids appear to be alcohols and isoalkanes. Yet, no correlation could be identified between the shift in d50 and a given thermophysical property. In fact, the difference between d50 at 0° and 10° is found to correlate well with the critical radius at the conditions prevailing in the activation region (Figure S4), which is a combined parameter, i.e., a parameter that depends on a number of working fluid properties.
These calculations only examine the favorable properties of the working fluid from an activation point of view. It is also important that the activated particles grow to sufficiently large sizes (>0.5 μm) (Zhang and Liu Citation1990) to allow their detection by the optical system. This is not addressed by heterogeneous nucleation theory that only considers saturation ratios and not the quantity of vapors available. An examination of the results presented in , illustrates that the size of droplets at the exit of the condenser (determined by condensational growth calculations) is too low when employing glycols and acids (with the exception of acetic acid).
In summary, calculations suggest that the benefit obtained from shifting to a different from butanol working fluid would be limited if the affinity of different calibration aerosol materials for that working fluid remains the same (i.e., the contact angle remains the same). Research should rather focus on the identification of liquids exhibiting a better tendency than butanol to condense onto different particles types at the operating conditions required to achieve a d50 at 23 nm (). Such investigations would probably require dedicated experiments. Of course, the suitability of a given working fluid for CPC applications would eventually depend on other parameters as well, including corrosiveness, toxicity, consumption, and cost, among others.
CONCLUSIONS
The counting efficiencies of two TSI 3790 CPCs designed to satisfy the requirements specified in UN-ECE Regulation 83 were experimentally investigated for graphite, PAO, tetradecane (C14), and hexadecane (C16) particles. The results obtained with PAO, C14, and C16 were broadly similar, and the results at 23 nm and 41 nm were in very good agreement with the calibration certificate values issued by the manufacturer. Tests with graphite particles, however, resulted in systematically lower counting efficiencies, in agreement with previous studies for soot, oxidized silver, and polystyrene spheres.
The effect of the calibration aerosol material is amplified at the low temperature differences between the condenser and the saturator, temperature differences necessary to comply with the regulation requirement of a 50% counting efficiency at 23 nm. The observed material dependence is in agreement with predictions of heterogeneous nucleation theory for particles of different affinity for butanol (i.e., different contact angles). Numerical calculations suggest that experimental data with PAO, C14, and C16 are representative of perfectly wettable particles. The experimentally observed lower affinity of graphite particles for butanol could be reproduced by a contact angle of 6–12°, for efficiencies up to 80% at all temperature differences examined.
Line tension values fitted on the graphite data spanned over almost one order of magnitude (5 × 10−11 N to 4 × 10−10 N), exhibiting a dependence on the temperature difference. Furthermore, the calculated line tensions were found to correlate linearly with the graphite mobility diameters. This could reflect a failure of the mobility size to capture the true contact-line length of aggregate particles, and accordingly their activation probability.
Even if the resulting contact angles and line tensions lie within the range of values reported in the literature, the calculations did not consider their synergetic effect on particle activation. This renders the problem of quantifying their value from experimentally determined CPC counting efficiencies ill-posed. Furthermore, unraveling the effect of these parameter is even more complicated by flow and temperature nonidealities that can have a strong effect on the calculated counting efficiencies, especially at the upper part of the counting efficiency curve (where most discrepancies between experimental and model results are observed).
The compliance of commercial CPC units to automotive regulation specifications was achieved by reducing the operating temperature differences of CPCs to reach a higher nominal cutoff size (e.g., Grimm 5.410 versus Grimm 5430, or TSI 3010D versus TSI 3010, or TSI 3790 versus TSI 3772). This raises the question whether room for further optimization exists. One particular option might be the use of an alternative working fluid with suitable thermophysical properties that would reduce the dependence of the counting efficiency on the affinity of different particles for the working fluid.
Numerical calculations performed with a range of working fluids, including alcohols, fatty acids, polyols, n-alkanes, chlorinated hydrocarbons, and aromatics, suggested that the dependence of the counting efficiency on the contact angle cannot be attributed to a single thermophysical property. The material dependence, as quantified by the difference between the 50% counting efficiencies at contact angles of 0° and 10°, reduces with increasing size of critical radius across the envelope of the activation region in the CPC condenser. The critical radius, though, is a combined property that depends on the temperature, the surface tension, the liquid density, and, most importantly, the saturation ratio. The determination of all these parameters in the activation envelope, as well as the location of activation envelope per se, requires the solution of the heat and mass transfer problems. This is not straightforward and requires extensive numerical calculations, like those described in the present study. Nevertheless, the results of the numerical calculations indicate that overall the benefit from shifting to an alternative working fluid in terms of reducing the dependence of the counting efficiencies on the contact angle is minimal. Future research should rather focus on the identification of a working fluid exhibiting a better tendency (affinity) to interact with different materials at the operating conditions required to achieve a cutoff size at 23 nm.
uast_a_716174_sup_27794170.zip
Download Zip (6.2 MB)Acknowledgments
[Supplementary materials are available for this article. Go to the publisher's online edition of Aerosol Science and Technology to view the free supplementary files.]
The authors would like to gratefully acknowledge Dominique Lesueur for his valuable contribution to the experimental work and Carsten Gruening and Sebastiao Martin Dos Santos for providing the nebulizer employed in the calibration of the DMAs. A. Mamakos and Y. Drossinos would like to thank Giorgio Martini, Panagiota Dilara, and Alois Krasenbrink for their support. INEOS Oligomers is also acknowledged for supplying the poly-(alpha)-olefin employed in the experiments. The authors would also like to thank TSI for supplying one of the TSI 3790 units employed in the study. The study was partially funded by the EMRP-ENV02 PartEmission project. The EMRP is jointly funded by the EMRP participating countries within EURAMET and the European Commission.
Notes
The CPCs do not exhibit a steep counting efficiency curve and therefore a well-defined cutoff size may not be indentified. This terminology is widely used in the literature as a surrogate to the mobility diameter at which the counting efficiency reaches 50% (d50 ). The two terms (cutoff size and d50 ) are employed interchangeably in the present study.
REFERENCES
- California EPA . 1998 . “ Part B: Health Risk Assessment for Diesel Exhaust ” . In Proposed Identification of Diesel Exhaust as an Air Toxic Contaminant http://www.arb.ca.gov/regact/diesltac/partb.pdf
- Commission Regulation 692/2008 . http://eurlex.europa.eu/LexUriServ/LexUriServ.do?uri=CELEX:32008R0692:en:NOT
- Commission Regulation 715/2007 . http://eurlex.europa.eu/LexUriServ/LexUriServ.do?uri=OJ:L:2007:171:0001:0016:EN:PDF
- Crooks , M. 2007 . “ The New Engine Exhaust CPC Model 3790 EECPC. 2007 ” . Cambridge : Particle Meeting .
- EPA . 2009 . Integrated Science Assessment for Particulate Matter (Final Report) , Washington , DC : U.S. Environmental Protection Agency . EPA/600/ R-08/139F, 2009. http://cfpub.epa.gov/ncea/cfm/recordisplay.cfm?deid=216546#Download
- Fletcher , N. H. 1958 . Size Effect in Heterogeneous Nucleation . J. Chem. Phys. , 29 : 572 – 576 .
- Giechaskiel , B. and Bergmann , A. 2011 . Validation of 14 used, re-calibrated and new TSI 3790 condensation particle counters according to the UN-ECE Regulation 83 . J. Aerosol Sci. , 42 : 195 – 203 .
- Giechaskiel , B. , Mamakos , A. , Andersson , J. , Dilara , P. , Martini , G. Schindler , W. 2012 . Measurement of Automotive Nonvolatile Particle Number Emissions within the European Legislative Framework: A Review . Aerosol Sci. Technol. , 46 : 719 – 749 .
- Giechaskiel , B. , Wang , X. , Gilliland , D. and Drossinos , Y. 2011 . The Effect of Particle Chemical Composition on the Activation Probability in n-Butanol Condensation Particle Counters . J. Aerosol Sci. , 42 : 20 – 37 .
- Giechaskiel , B. , Wang , X. , Horn , H. , Spielvogel , J. , Gerhart , C. Southgate , J. 2009 . Calibration of Condensation Particle Counters for Legislated Vehicle Number Emission Measurements . Aerosol Sci. Technol. , 43 : 1164 – 1173 .
- Hienola , A. I. , Winkler , P. M. , Wagner , P. E. , Vehkamäki , H. , Lauri , A. Napari , I. 2007 . Estimation of Line Tension and Contact Angle from Heterogeneous Nucleation Experimental Data . J. Chem. Phys. , 126 : 094705
- Hinds , W. C. 1999 . Aerosol Technology. Properties, Behaviour and Measurement of Airborne Particles. Second Edition , New York : John Wiley and Sons .
- Housiadas , C. , Ezquerra Larrodé , F. and Drossinos , Y. 1999 . Numerical Evaluation of the Graetz Series. . Int. J. Heat Mass Transfer. , 42 : 3013 – 3017 .
- Housiadas , C. , Ezquerra Larrodé , F. and Drossinos , Y. 2000 . Convective Diffusion in a Tube with Non-Uniform Inlet Conditions . J. Aerosol Sci. , 31 : 959 – 968 .
- IARC . 1989 . Diesel and Gasoline Engine Exhausts and Some Nitroarenes , Lyon : International Agency for Research on Cancer .
- Jing , L. 1999 . Standard Combustion Aerosol Generator (SCAG) for Calibration Purposes . 3rd ETH Workshop “Nanoparticle Measurement,” ETH Hönggerberg, Zürich, 9–10 August 1999 http://www.sootgenerator.com/documents/Pub-ETH-Workshop1999_Ji.pdf
- Lazaridis , M. 1993 . The Effects of Ssurface Ddiffusion and Line Tension on the Mechanism of Heterogeneous Nucleation . J. Colloid Interface Sci. , 155 : 386 – 391 .
- Magnusson , L. E. , Koropchak , J. A. , Anisimov , M. P. , Pznjakovskiy , V. M. and de la Mora , J. F. 2003 . Correlations for Vapor Nucleating Critical Embryo Parameters . J. Phys. Chem. Ref. Data , 32 : 1387 – 1410 .
- Mamakos , A. , Giechaskiel , B. , Drossinos , Y. , Lesueur , D. , Martini , G. and Krasenbrink , A. 2011 . Calibration and Modeling of PMP Compliant Condensation Particle Counters . JRC Scientific and Technical Report, EUR 25145 EN
- Mathis , U. , Mohr , M. , Kaegi , R. , Bertola , A. and Boulouchos , K. 2005 . Influence of Diesel Engine Combustion Parameters on Primary Soot Particle Diameter . Env. Sci. Technol. , 39 : 1887 – 1892 .
- NIOSH . 1988 . Carcinogenic Effects of Exposure to Diesel Exhaust , Cincinnati , OH : U.S. Department of Health and Human Services, National Institute for Occupational Safety and Health .
- Stolzenburg , M. R. and McMurry , P. H. 1991 . An Ultrafine Aerosol Condensation Nucleus Counter . Aerosol Sci. Technol. , 14 : 48 – 65 .
- Wang , J. Y. , Betelu , S. and Law , B. M. 2001 . Line Tension Approaching a First-Order Wetting Transition: Experimental Results From Contact Angle Measurements . Phys. Rev. E , 63 : 031601
- Wang , X. , Caldow , R. , Sem , G. J. , Hama , N. and Sakurai , H. 2010 . Evaluation of a Condensation Particle Counter for Vehicle Emission Measurement: Experimental Procedure and Effects of Calibration Aerosol Material . J. Aerosol Sci. , 41 : 306 – 318 .
- Wentzel , M. , Gorzawski , H. , Naumann , K.-H. , Saathoff , H. and Weinbruch , S. 2003 . Transmission Electron Microscopical and Aerosol Dynamical Characterization of Soot Aerosols . J. Aerosol Sci. , 34 : 1347 – 1370 .
- Zhang , Z. Q. and Liu , B. Y. H. 1990 . Dependence of the Performance of TSI 3020 Condensation Nucleus Counter on Pressure, Flowrate and Temperature . Aerosol Sci. Technol. , 13 : 493 – 504 .