Abstract
Differential mobility analyzers (DMAs) with more than one monodisperse-particle outlet can offer a number of advantages compared to conventional single monodisperse-particle outlet designs. A generalized theoretical model and experimental measurements describing the performance of a DMA with 3 monodisperse-particle outlets have been independently reported in the literature. The objective of this article is to compare the theoretical predictions with the measurements. Resolutions determined by the theoretically predicted transfer functions for the three monodisperse-particle outlets are compared with measurements when the DMA was operated under different operating conditions. Predictions and measurements show good agreement when the DMA is operated at low sheath flow rates and for aerosol outlets relatively far from the aerosol inlet. For aerosol outlets relatively near the inlet there is evidence that the discrepancy between theoretical predictions and measurements may disappear at higher sheath flow rates, but the chances of flow disturbances in the classifier increase as well. The theory for multiple monodisperse-outlet DMAs is thus seen as successful in predicting the performance of this instrument, provided that disturbances in the flow field are avoided.
Copyright 2013 American Association for Aerosol Research
1. INTRODUCTION
The differential mobility analyzer (DMA) is the most efficient tool for determining the size of aerosol particles in the sub-micrometer and nanometer range by directly measuring their electrical mobility (Flagan Citation1998; McMurry Citation2000; Biskos et al. Citation2008). When used as classifiers in electrical mobility spectrometers, DMAs are typically operated in either a scanning or a stepping mode in order to select particles over a wide range of the mobility spectrum. The required time for scanning or stepping of the operating conditions (typically the potential difference between the two electrodes) can range between 30 s to a few minutes depending on the desired resolution of the measurements and the particle detector employed (Wang et al. Citation2002). Using fast-response mixing-type condensation particle counters (CPCs) (Okuyama et al. Citation1984; Kousaka et al. 1985) in electrical mobility spectrometers, one can use scanning times down to a few seconds without sacrificing the resolution of the mobility distribution measurement (Wang et al. Citation2002). Such particle counters, however, are not yet widely available.
Another way to reduce the response time, which in addition can increase the dynamic range of electrical mobility spectrometers, is to employ mobility instruments that can simultaneously classify particles in different channels. Instruments that fulfill these two objectives include the Tartu spectrometer (Tammet et al. Citation1998, Citation2002), the fast mobility particle spectrometer (FMPS; Johnson et al. Citation2004), and the differential mobility spectrometer (DMS; Biskos et al. Citation2005). Although these instruments have a short response time (i.e., of the order of a second) and a wide dynamic range (from a few nanometers up to a few microns), they suffer from (a) low resolution as a result of the finite width of the channels, and (b) low signal-to-noise ratio because detection is performed by electrometers. A solution around these two limitations is to use a multiple monodisperse-outlet DMA (MMO-DMA), in combination with CPCs. An MMO-DMA operated at fixed conditions can simultaneously select monodisperse particles of different sizes with a very high resolution, whereas the detection limit of CPCs can enable measuring of aerosols of very low particle concentrations.
Chen et al. (Citation2007) built and tested a DMA having three monodisperse-particle outlets, and recently Giamarelou et al. (Citation2012) have derived a generalized theoretical framework for describing the performance of any MMO-DMA. The performance of a DMA can be fully determined by its transfer function, i.e., the function that gives the probability of a particle of a given mobility that enters the classifier to exit through the monodisperse-particle outlet. From the theoretical point of view, the DMA transfer function for relatively large particles can be derived by considering their deterministic trajectories within the column (Knutson and Whitby Citation1975). For smaller particles, however, Brownian motion causes deviation from their deterministic paths, thereby resulting in a broadening of the transfer function (Kousaka et al. Citation1986; Stolzenburg Citation1988; Flagan Citation1999; Hagwood et al. Citation1999; Salm Citation2000; Fernandez de la Mora Citation2002). From the experimental point of view, the transfer function of the DMA can be determined using a tandem DMA system (Fissan et al. Citation1996; Birmili et al. Citation1997; Martinsson et al. Citation2001). In this system, the first DMA is used to produce a flow of monodisperse particles, which is then passed through the second DMA whose transfer function needs to be evaluated. The use of two identical DMAs operated under the same flow conditions is preferred as it minimizes the error propagation of the retrieved transfer function (Mei et al. Citation2011).
In this article, we employ the experimental measurements of the transfer function of the DMA with three monodisperse-particle outlets (referred to as the 3-MO-DMA from this point onward) provided by Chen et al. (Citation2007) to evaluate the performance of the theoretical MMO-DMA transfer function derived by Giamarelou et al. (Citation2012). We first compare the predicted with the measured transfer functions for 20-nm particles when the DMA is operated under balanced and unbalanced flow conditions. To evaluate how well our model captures the effect of particle diffusivity, we then compare the predicted with the measured resolution for each outlet when particles of different diameter are classified through the DMA.
2. METHODOLOGY
2.1. Experimental
shows a schematic diagram of the 3-MO-DMA that is used in our evaluation. Details of its design are provided by Li (Citation2006) and Chen et al. (Citation2007). In brief, it is a cylindrical DMA with two coaxial electrodes having radii of 1.27 cm and 1.75 cm, and monodisperse-particle outlets located 2.54 cm, 7.62 cm, and 15.24 cm from the polydisperse aerosol inlet. The polydisperse aerosol flow is introduced alongside the central high-voltage electrode, and the classified particles are repelled to the outer electrode where the three outlets are located. Although the current design was not optimized for high-resolution particle classification, the data provided by Chen et al. (Citation2007) are the only available experimental results at present with which we can compare the predictions of our theoretical model.
FIG. 1 Schematic diagram of the DMA with three monodisperse-particle outlets (3-MO-DMA) located at distances L 1 = 15.24 cm, L 2 = 7.62 cm, and L 3 = 2.54 cm downstream of the polydisperse aerosol inlet. The radii of the inner and the outer electrodes are R 1 = 1.27 cm and R 2 = 1.75 cm.
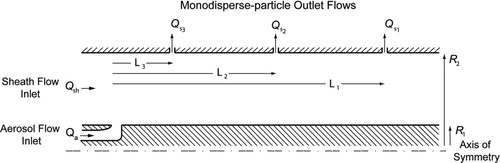
The transfer function of the 3-MO-DMA has been determined experimentally using the tandem DMA (TDMA) technique (see Li Citation2006, for a detailed description of these measurements). In brief, a nano-DMA (TSI Model 3085) was used as the first DMA in the system. The operating flows and the applied potential difference between the electrodes of the nano-DMA were adjusted for each measurement in order to select particles having diameters from 6 to 45 nm (Li Citation2006; Chen et al. Citation2007). Two operating modes of the 3-MO-DMA were used during the measurements: in the first mode the total polydisperse aerosol flow was equal to the sum of the three outlet streams (i.e., unbalanced flow conditions), whereas in the second mode, the sampling flow rate at each outlet was equal to the polydisperse aerosol inlet flow rate (i.e., balanced flow conditions). The potential difference between the electrodes of the 3-MO-DMA, used as the second DMA in the TDMA setup, was varied in order to measure the number of particles collected through the different channels.
The transfer function of the multiple-outlet DMA was then determined using a piecewise-linear function deconvolution algorithm (Li Citation2006; Li et al. Citation2006). This algorithm retrieves the DMA transfer function without pre-assuming its shape. The entire electrical mobility window of the true transfer function is divided into N subsections, and a linear function is assumed in each one of them. A conjugate gradient method is used to obtain the optimal values of the parameters defining the piecewise-linear function by fitting the predicted TDMA response to the experimental measurements. A least-square error criterion is used for the convergence of the iteration procedure (Li Citation2006; Li et al. Citation2006).
2.2. Theoretical
The theoretical framework for determining the transfer function of cylindrical DMAs with multiple monodisperse-particle outlets (MMO-DMAs) has been derived by Giamarelou et al. (Citation2012). Assuming that the flow field is axisymmetric and steady and that the airflow is laminar and incompressible, the transfer function of the ith outlet of the MMO-DMA for diffusing particles is given by

One way to quantify the disparity between the theoretical and the measured transfer functions or resolutions is to introduce several adjustable parameters into EquationEquation (1) in order to fit the model to the measurements. The parametric transfer function can be expressed as follows:

• | η
i
is the ratio of the penetration efficiency through the DMA plumbing leg in the TDMA experimental setup, excluding the DMA classification section itself, over the penetration efficiency through the parallel plumbing leg that bypasses the DMA in order to allow for the measurement of the aerosol concentration upstream of the DMA ( of Chen et al. Citation2007). This parameter represents a simple vertical scaling of the transfer function and has no effect on the resolution. It is a necessary scaling factor in our analysis as the measured FIG. 2 Comparison between predicted and measured transfer functions of the 3-MO-DMA for (a) the outlet furthest from the inlet (i.e., L 1 = 15.24 cm) when the flow rates through the column are Qsh = 20 lpm, Qa = 2.4 lpm, and Q s1 = Q s2 = Q s3 = 0.8 lpm, and the potential difference between the two electrodes is V = 196 V and for (b) the outlet nearest to the inlet (i.e., L 3 = 2.54 cm) when the flow rates are Qsh = 20 lpm, Qa = 1.5 lpm, and Q s1 = Q s2 = Q s3 = 1.5 lpm, and the potential difference between the two electrodes is V = 1260 V. In both cases, the potential difference between the two DMA electrodes is set to select particles having mobility diameter of 20 nm. ![]() | ||||
• |
| ||||
• | β′ i and δ′ i represent corrections to the flow parameters due to errors in the measured flow rates. In the absence of any mixing of flows, δ′ i determines the height of the non-diffusing transfer function, whereas β′ i and δ′ i together determine its width at half the maximum. An increase in β′ i or |δ′ i | over its nominal value reduces the resolution. | ||||
• |
| ||||
• |
| ||||
• | σ′2
i
is the total adjusted spread parameter, which is calculated from |
The parameters η
i
,
, and
or
are used to fit the model to the measured transfer function. The other two adjustable parameters, β′
i
and δ′
i
, remain fixed at their respective theoretical values. Besides the height η
i
, the primary requirement to fit the model to the measured transfer function was found to be the spread σ′2
i
. For a single transfer function measured at a given set of conditions, it is virtually impossible to distinguish the effects of
and
. Thus, the predicted transfer function was fitted to the measured transfer function both ways. The variable parameters η
i
,
, and
or
allow for the (nearly) independent adjustment of the area, mean, and standard deviation, respectively, of the model transfer function, which can then be fit to the corresponding quantities of the measured transfer function. As η
i
< 1 is an expected result, it is only the deviation
and
or
that are of interest as measures of the disparity between the measured transfer function and the original prediction (EquationEquation (1)).
The measured and predicted resolutions of the DMA can be numerically determined from the measured and predicted transfer functions, respectively, as the inverse of the full width at half maximum (FWHM) normalized by the centroid mobility. When fitting the model to the measured resolution, we found it most convenient to use the adjustable parameters ,
, and δ′
i
. Parameter η
i
has no effect on the resolution, and the effects of small variations in
are virtually undetectable. For negligible variations in
(i.e., within the uncertainty of the flow rate measurements) about the predicted value of the resolution in the non-diffusing limit,




3. RESULTS
shows the comparison between predicted and measured transfer functions when the 3-MO-DMA is operated at different conditions. Measurements and predictions shown in correspond to the transfer function for the outlet furthest from the inlet (i.e., outlet #1 as depicted in ) when the flow rates through the DMA are Qsh = 20 lpm, Qa = 2.4 lpm, and Q s1 = Q s2 = Q s3 = 0.8 lpm, and the potential difference between the two electrodes is set to select particles having mobility diameter of 20 nm. Although these operating conditions correspond to an unbalanced flow scheme (i.e., δ i ≠ 0), they enable the use of a closed-loop sheath-flow system that gives a higher measurement precision. On the other hand, in the case of balanced flows (which can only be achieved with an open sheath-flow system), one can maximize the transfer function of each outlet by increasing the Q si /Qa ratio.
Two model transfer functions are shown in . The first, “Predicted,” corresponds to calculations using EquationEquation (1), whereas the second, “Fitted,” uses EquationEquation (2) after matching the area, mean, and standard deviation to the respective values from the measurements. The adjustable parameters used in EquationEquation (2) to achieve the fit are η′ = 0.808, , and σ
mix
= 0.0161 or fG
= 1.71. The first value indicates that there is a 19% relative loss in the plumbing leading up to and following the DMA classification section compared to that in the DMA bypass line. The second value indicates a shift in the centroid of the transfer function of less than 0.4%, which is well within the experimental uncertainty of the sheath and excess airflow rates. The third value (expressed as σ
mix
or fG
) represents a 14% increase in the spread of the transfer function as measured by its total variance. The FWHMs for the measured, predicted, and fitted transfer functions are 0.121, 0.125, and 0.127, respectively. The predicted and the measured transfer functions are overall in good agreement in this case.
compares the predicted with the measured transfer function for sample outlet #3 (L 3 = 2.54 cm) of the DMA when the flow rates through the classifier are Qsh = 20 lpm, Qa = 1.5 lpm, and Q s1 = Q s2 = Q s3 = 1.5 lpm, and the centroid particle diameter is 20 nm. In this case, the operating conditions correspond to balanced flow conditions (i.e., δ i = 0), resulting in a shape of the transfer function that is closer to triangular.
The fitting process yielded values of the adjustable parameters as follows: η′ = 0.896, , and σ
mix
= 0.0253 or fG
= 12.1. This last corresponds to a 64% increase in the total variance of the transfer function. The FWHMs for the measured, predicted, and fitted transfer functions are 0.089, 0.081, and 0.100, respectively. Both measures of spread or width indicate that the measured transfer function is substantially broader than the predicted one.
Next we compare the predicted with the measured resolution of each of the monodisperse-particle outlets as a function of particle size. As described in Section 2.2, the resolution of each monodisperse-particle outlet of the DMA can be determined numerically as the inverse of the FWHM of the measured or predicted transfer functions, and analytically using EquationEquations (3) and Equation(4) for the non-diffusing and diffusing limiting cases, respectively. – show measured (symbols), predicted (normal lines; EquationEquation (1)) and fitted model (bold lines; EquationEquation (2)) resolutions at various sets of DMA operating conditions. Shaded areas about the predicted lines correspond to a ±2% uncertainty in the measured flow rates. Fit parameters and their 95% confidence intervals are summarized in . The confidence intervals are unusually wide because of the small sample sizes for the measurements.
TABLE 1 Values and 95% confidence limits of the fit parameters δ′,
, and fG
used in EquationEquation (2) to fit the modeled to the measured resolutions
FIG. 3 Comparison between predicted (curves) and measured (symbols) resolutions for outlets 1 (dash-dotted), 2 (dashed), and 3 (solid) of the 3-MO-DMA. The flow rates through the DMA are (a) Qsh = 20 lpm, Qa = 2.4 lpm, and Q s1 = Q s2 = Q s3 = 0.8 lpm (i.e., unbalanced flows) and (b) Qsh = 20 lpm, Qa = 2.4 lpm, and Q s1 = Q s2 = Q s3 = 2.4 lpm (i.e., balanced flows). Normal curves correspond to the numerical predictions when using the nominal flows, and the shaded area when assuming a ±2% variation. Bold curves correspond to the model (EquationEquation (2)) fitted to the measurements. Inset: predictions using the numerical (black line; EquationEquation (1)), the non-diffusing limit (gray horizontal line; EquationEquation (3)), and the diffusing limit (gray sloped line; EquationEquation (4)) resolutions.
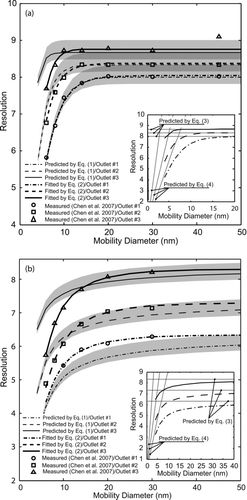
FIG. 4 Comparison between predicted (curves) and measured (symbols) resolution for outlets 1 (dash-dotted), 2 (dashed), and 3 (solid) of the 3-MO-DMA. The flow rates through the DMA are (a) Qsh = 36 lpm, Qa = 4.5 lpm, and Q s1 = Q s2 = Q s3 = 1.5 lpm (i.e., unbalanced flows) and (b) Q sh = 36 lpm, Q a = 1.5 lpm, and Q s1 = Q s2 = Q s3 = 1.5 lpm (i.e., balanced flows). Normal curves correspond to the numerical predictions when using the nominal flows, and the shaded area when assuming a ±2% variation. Bold curves correspond to the model (EquationEquation (2)) fitted to the measurements. Inset: predictions using the numerical (black line; EquationEquation (1)), the non-diffusing limit (gray horizontal line; EquationEquation (3)), and the diffusing limit (gray sloped line; EquationEquation (4)) resolutions.
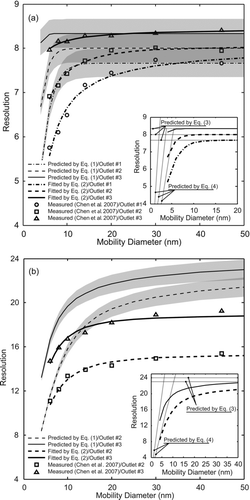
FIG. 5 Comparison between predicted (curves) and measured (symbols) resolution for outlets 1 (dash-dotted) and 2 (dashed) of the 3-MO-DMA. The flow rates through the DMA are Qsh = 50 lpm, Qa = 4.5 lpm, and Q s1 = Q s2 = Q s3 = 1.5 lpm (i.e., unbalanced flows). Normal curves correspond to the numerical predictions when using the nominal flows, and the shaded area when assuming a ±2% variation. Bold curves correspond to the model (EquationEquation (2)) fitted to the measurements. Inset: predictions using the numerical (black line; EquationEquation (1)), the non-diffusing limit (gray horizontal line; EquationEquation (3)), and the diffusing limit (gray sloped line; EquationEquation (4)) resolutions.
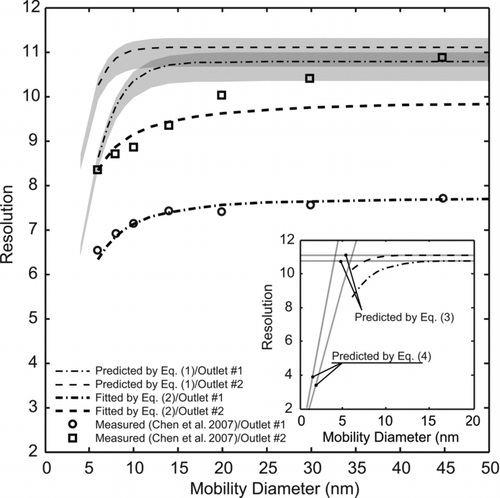
shows the comparison between predicted and measured resolutions as a function of particle size for all three outlets of the DMA, when the sheath flow rate is set to 20 lpm. The data shown in correspond to unbalanced flow conditions, whereas those in to balanced flows. In the case of the unbalanced flows (i.e., Qsh = 20 lpm, Qa = 2.4 lpm, and Q s1 = Q s2 = Q s3 = 0.8 lpm), there is excellent agreement between the experimental and the numerically predicted resolution for sample outlets #1 and #2 for the whole particle size range tested (i.e., from 6 to 45 nm). For outlet #3 the agreement is within experimental uncertainty for particles having mobility diameter down to 10 nm, but for smaller particles the predicted resolution overestimates the measurements by up to 8.5% (e.g., for the 6-nm particles). Note that the point at 45 nm for outlet #3 is clearly outside reasonable limits of uncertainty in the non-diffusing resolution as determined by the measurements in the 10–30 nm range such that it has been designated as an outlier and excluded from analysis here. Considering a ±2% uncertainty in the flow rates (i.e., using a 2% increase or decrease of the sheath flow rate with simultaneous 2% decrease or increase of both the aerosol and the sample flow rates, respectively) gives a maximum uncertainty in the predicted resolution of ±4.2% for outlet #1, ±4.0% for outlet #2, and ±3.8% for outlet #3. The uncertainty increases for the outlets furthest from the inlet because more flows (i.e., those of the outlets that precede) indirectly define the total flow in the respective sections of the column. For outlet #1, the fitted model is nearly indistinguishable from the prediction. For the other two outlets, there is a clear difference between fitted/measured and predicted resolutions for particles having diameters equal to or smaller than 10 nm. For outlet #2, the predicted value of fG = 1.00 is just beyond the confidence interval of the fitted value of fG = 1.25, whereas for outlet #3 the fitted value of fG is significantly greater than one. The confidence intervals of the fitted values of δ′ all include the nominal value of δ = −0.5.
shows the comparison between measured and predicted resolution when the DMA is operated under balanced flows (i.e., Qsh
= 20 lpm, Qa
= 2.4 lpm, and Q
s1 = Q
s2 = Q
s3 = 2.4 lpm). Because the flow removed by each monodisperse-particle outlet relative to the total flow through the DMA is much higher compared to the case of the unbalanced flows (i.e., measurements and predictions shown in ), the resolution here decreases noticeably as we move from the nearest to the furthest outlet from the aerosol inlet. Agreement between numerically predicted and measured resolution is within experimental uncertainty for particles larger than ca. 10 nm for both sample outlets #2 and #3. For smaller particles, the numerical predictions overestimate the measurements by as much as 22% (e.g., for the 6-nm particles classified through outlet #3). For sample outlet #1 the theoretically predicted resolution is systematically less than the measured resolution for the entire size range. For all three outlets the fitted value of δ′ is notably different from zero. Theoretically, the modeled resolution is independent of the sign of δ. Note that the confidence intervals for the fitted parameters for outlet #1 are especially large due to the reduced sample size for this data set. As in , the fitted values of fG
for outlets #2 and #3 are significantly greater than #1. For the fits shown in both figures, the value of fG
increases the closer the outlet is to the inlet in both cases. The fitted values of are also within 1% or less of the corresponding predicted values, which is well within the range defined by the uncertainty of the flows.
shows the comparison between predicted and measured resolutions for all three monodisperse-particle outlets of the DMA when the sheath flow rate is set to 36 lpm. and show the predicted and the measured resolutions in the case of unbalanced and balanced flow conditions, respectively. When the DMA is operated under unbalanced flows (i.e., Qa
= 4.5 lpm and Q
s1 = Q
s2 = Q
s3 = 1.5 lpm), the experimental and the theoretically predicted resolutions of all three outlets are in agreement, within measurements uncertainty, for particles having mobility diameters larger than ca. 14 nm. More specifically, for sample outlet #3 the measured resolution for almost the entire size range is between the two limiting predicted curves that correspond to a ±2% change in the flow rates. For sample outlets #2 and #1 that happens only for particles larger than ca. 14 nm, and for smaller particles the measured resolution drops faster than the predicted. The nominal value of δ for all three outlets is -0.5, but, surprisingly, the fitted values of δ for all three are essentially zero. Contrary to the plots in and , the value of fG
decreases the closer the outlet is to the inlet. Some of the fits here also show greater deviations from the predicted values of than in .
shows the comparison between predicted and measured resolutions for monodisperse-particle outlets #2 and #3 of the DMA (experimental data for outlet #1 are not available) when the sheath flow is set to 36 lpm, and the aerosol and sample flows are balanced (i.e., Qa
= 1.5 lpm and Q
s1 = Q
s2 = Q
s3 = 1.5 lpm). Under these operating conditions the experimental measurements deviate substantially from the numerically predicted resolutions. This deviation increases from 10.8% to 16.6% for outlet #3, and from 1.0% to 34.7% for outlet #2, when particle size increases from 6 nm to 45 nm. The reduction of the measured values from the theoretically predicted ones is substantially beyond that which might be accounted for by uncertainties in flows. This can only be due to some sort of flow disturbance in the DMA. Though the model of EquationEquation (2) using
with β′
i
= β
i
was used to fit the measurements, the uncertainty of the fitted parameters was so large as to render the fitted values meaningless.
compares the predicted and the measured resolution for sample outlets #1 and #2, when the DMA is operated at Qsh = 50 lpm, Qa = 4.5 lpm, and Q s1 = Q s2 = Q s3 = 1.5 lpm. The discrepancy between measured and numerically predicted values is high, with the measured resolution being less than the predicted in all cases. The difference increases from 30.9% to 38.5% for outlet #1 and decreases from 21.8% to 1.8% for outlet #2, when particle size increases from 6 nm to 45 nm. For outlet #2, the gradual approach of the resolution to the non-diffusing limit as diameter increases cannot be fit with the model given in EquationEquation (2) while the resolution for outlet #1 is clearly limited by flow mixing.
4. DISCUSSION
Consider, first, the transfer functions in . Though the fit is quite good in (δ ≠ 0) with only a slight increase in spread, the fit in (δ = 0) is only fair with a significant increase in spread and a distinct problem at the peak of the transfer function. As noted in both the predicted and modeled curves, any amount of diffusion should quickly round the apex of the familiar triangular non-diffusing transfer function. This is also true of any disturbance (σ2 mix > 0) in the flows of the DMA. It is therefore puzzling to see such a prominent peak on the measured transfer function. This could in part be an artifact of the fitted piecewise-linear spline, which has a node right at the centroid. Such a spline will inherently have difficulty tracking any sharp bend in the transfer function. In such a situation, the spline nodes will tend to be on the outside of the bend as is the case here. The extension of the node above the anticipated rounded peak of the model, however, is greater than expected, even with the addition of a bit of measurement uncertainty. The slight flaring of the base of the measured transfer function from a strictly triangular shape would tend to confirm the effect of a small amount of diffusion not evident in the peak. An explanation for this seeming contradiction is not forthcoming.
The shape of the non-diffusing transfer function can have a marked effect on the resolution as a function of particle diameter. Consider the sharpness of the bend between the theoretically predicted non-diffusing and diffusing limits of the resolution shown in and . For |δ| = 0.5 as in , the bend is quite sharp, while for |δ| = 0 as in , the bend is much gentler. This can be readily explained as follows. The effect of diffusion as diameter decreases can be thought of as running a Gaussian average of ever-increasing width over the span of the transfer function. Given the piecewise-linear nature of the theoretical transfer function, for an initially narrow averaging window, there is no effect on the bulk of the linear sections but the corners are rounded. This initial rounding is restricted to the regions immediately adjacent to the corners and does not reach to the center of the linear pieces of the transfer function. For a trapezoidal transfer function (i.e., |δ| > 0), neither the height nor the FWHM is affected for small amounts of diffusion. Thus, the resolution is maintained at the value of even with a significant degree of diffusion. Even when the averaging effect reaches the midpoints of the sides of the trapezoid, the effects from the upper and lower corners will actually cancel each other out. When the averaging window gets wide enough, the rounding effects of the upper corners reach the midpoint of the top of the trapezoid. There they add together to lower the peak of the transfer function, thereby increasing the FWHM value and lowering the resolution. For a triangular transfer function (i.e., |δ| = 0), the peak of the transfer function begins to be rounded even with the smallest amount of diffusion, thereby decreasing the height of the transfer function and the resolution. As a result, the behavior of the mid-range of the resolution curve is quite sensitive to the value of |δ|.
In , the fits of the model to the resolution data gave consistent values of |δ′| notably greater than the nominal value |δ| = 0. This can be explained by possible flow mismatches between the aerosol inlet and outlet. In Figure 3a, the seeming difference between the fitted values of δ′≅ − 0.4 (note that the higher value of δ′ for outlet #3 has a much wider confidence interval) and a nominal value of δ = −0.5 would tend to confirm this mismatch in flows. Since, this offset in δ′ from δ is in the positive direction, this suggests that the flow through the aerosol outlet was greater than that reported.
In , both plots show the same increasing trend of the fitted values of fG as the aerosol outlet gets nearer the aerosol inlet. This discrepancy between theory and measurements seems most likely to be due to a problem with the theory. One of the weaknesses of the theory is the estimate of Gradial , a geometric- and flow-dependent factor associated with the radial velocity of a particle in the DMA. The expression used by Giamarelou et al. (Citation2012) to calculate Gradial neglects the effects of radial flows in the vicinity of the aerosol inlet and outlet slits. This was justified by a rough calculation of the effect while the particle passes through the region near an outlet slit while transiting to an outlet slit further downstream. Also, in this calculation, Li ≫ R 2 − R 1 such that the contribution to Gtotal due to the axial component of particle velocity was far greater than that of the radial component. The largest component of Gradial that is neglected is probably that due to the accelerating flow velocity in the converging flow as the particles approach the outlet slit that they will exit. The flow velocities in that region are generally much higher than those in the diverging flow from the aerosol inlet slit because the outlet slits are usually much narrower than the inlet slit. It is also very important to match the inlet aerosol flow velocity with the sheath flow to avoid flow disturbances. The higher the particle velocity the greater the contribution to the estimate of G, so the neglected contribution to G at the outlet slit should be greater than that at the inlet slit. A very crude estimate of the former contribution indicates that it can more than double the value of Gradial , but, even for Li ≅5 · (R 2 − R 1)—corresponding to the aerosol outlet nearest to the inlet, where the radial velocities relative to the axial velocities are the greatest—the relative contribution of Gradial to Gtotal is still quite small. Thus, it is difficult to see how this effect could account for fitted fG values as high as 5.
In , the fits of the model to the measurements look good though not quite as good as those in . For all the outlets, the fitted values of δ′ are essentially zero as compared to their nominal value of − 0.5. The offsets here are greater than those observed in , but also positive. The fitted values for fG nominally show the opposite trend with outlet distance from the inlet. However, the value fG = 1 is within the confidence intervals for both outlets #1 and #2. For outlet #3, given the lack of data near the diffusing limit for the resolution, it is surprising that the confidence interval for fG should be so small, even smaller than that of the other outlets. Potential problems associated with the fitting process do not necessarily contradict the possibility for fG = 1 for all the outlets. This would be in contrast to the trend found in the fits of , but it can be explained by the higher sheath flow, which can increase Gaxial relative to Gradial such that any error in the latter could become negligible, relative to Gtotal . Finally, upon closer inspection, one can observe a high degree of correlation between the residuals of the measurements about the respective curves of the fitted models for outlets #1 and #2 in . This would suggest that these residuals are not due to random errors in the measurements but rather an inadequacy in the theory. There is nothing obvious in the model that could be modified to accommodate this phenomenon.
For and , there is strong evidence of problems from disturbances in the flow. Although and both use a sheath flow of 36 lpm, the difference in the aerosol inlet flows may account for the presence of flow disturbances for the conditions of the measurements shown in while not for those in . Most DMAs are designed to minimize the chance of flow disturbances at the confluence of the aerosol inlet and sheath flows for a flow ratio of 1/10. This flow ratio is much closer to that corresponding to the conditions of the measurements shown in (0.125) than for those in (0.042). As a result, there might be flow disturbances at this confluence in the latter case while not in the former. For outlet #2 in , the measurements show a clear trend to approach the theoretical resolution in the non-diffusing limit. This is very different from the observed behavior of the measurements at outlet #1, where the measured resolution appears to plateau in the non-diffusing limit at values well below of those of the predicted resolution. Although this difference is odd, it is possible that it is a consequence of some complexity of the flow mixing that the crude model used in EquationEquation (2) cannot mimic.
5. CONCLUSIONS
The performance of the MMO-DMA transfer function model provided by Giamarelou et al. (Citation2012) was shown to be in good agreement with measurements using the 3-MO-DMA designed and tested by Chen et al. (Citation2007), when operated with low flow rates. The measured and calculated transfer functions were in good agreement, when the sheath flow rate was 20 lpm. This was true both for balanced and unbalanced flow conditions, and assuming some measurement errors in the aerosol outlet flow associated with flow mismatches. This was the case only for the aerosol outlets furthest from the inlet. For outlets closer to the inlet, the predictions overestimate the measured resolutions, possibly due to an inadequacy of the theory. For higher sheath flows (i.e., 36 lpm and greater), the theoretical overestimation of the measured resolution for outlets closer to the inlet may essentially disappear but the chance of flow disturbances increases significantly, thereby reducing the resolution. At least for the 3-MO-DMA designed and tested by Chen et al. (Citation2007), using low sheath flows to avoid flow disturbances is particularly important. As for the theory, it appears that additional work is needed to improve the accuracy for relatively short column lengths.
Acknowledgments
This research has been co-financed by the European Social Fund (ESF, European Union) and Greek national funds through the Operational Program “Education and Lifelong Learning” of the National Strategic Reference Framework (NSRF)—Research Funding Program: Heracleitus II. Investing in knowledge society through the European Social Fund.
REFERENCES
- Birmili , W. , Stratmann , F. , Wiedensohler , A. , Covert , D. , Russell , L. M. and Berg , O. 1997 . Determination of Differential Mobility Analyzer Transfer Functions Using Identical Instruments in Series . Aerosol Sci. Technol. , 27 : 215 – 223 .
- Biskos , G. , Reavell , K. and Collings , N. 2005 . Description and Theoretical Analysis of a Differential Mobility Spectrometer . Aerosol Sci. Technol. , 39 : 527 – 541 .
- Biskos , G. , Vons , V. , Yurteri , C. U. and Schmidt-Ott , A. 2008 . Generation and Sizing of Particles for Aerosol-based Nanotechnology . KONA , 26 : 13 – 35 .
- Chen , D. R. , Li , W. and Cheng , M. D. 2007 . Development of a Multiple-Stage Differential Mobility Analyzer (MDMA) . Aerosol Sci. Technol. , 41 : 217 – 230 .
- Coleman , T. F. and Li , Y. 1994 . On the Convergence of Reflective Newton Methods for Large-Scale Nonlinear Minimization Subject to Bounds . Math. Program. , 67 : 189 – 224 .
- Coleman , T. F. and Li , Y. 1996 . An Interior, Trust Region Approach for Nonlinear Minimization Subject to Bounds . SIAM J. Optimiz. , 6 : 418 – 445 .
- Fernandez de la Mora , J. 2002 . Diffusion Broadening on Converging Differential Mobility Analyzers . J. Aerosol Sci. , 33 : 411 – 437 .
- Fissan , H. , Hummes , D. , Stratmann , F. , Buscher , P. , Neumann , S. Pui , D. Y. H. 1996 . Experimental Comparison of Four Differential Mobility Analyzers for Nanometer Aerosol Measurements . Aerosol Sci. Technol. , 24 : 1 – 13 .
- Flagan , R. C. 1998 . History of Electrical Aerosol Measurements . Aerosol Sci. Technol. , 28 : 301 – 380 .
- Flagan , R. C. 1999 . On Differential Mobility Analyzer Resolution . Aerosol Sci. Technol. , 30 : 566 – 570 .
- Giamarelou , M. , Stolzenburg , M. and Biskos , G. 2012 . The Multiple Monodisperse Outlet Differential Mobility Analyzer: Derivation of its Transfer Function and Resolution . Aerosol Sci. Technol. , 46 : 951 – 965 .
- Hagwood , C. , Sivathanu , Y. and Mulholland , G. 1999 . The DMA Transfer Function with Brownian Motion A Trajectory/Monte-Carlo Approach . Aerosol Sci. Technol. , 30 : 40 – 61 .
- Johnson , T. , Caldow , R. , Pöcher , A. , Mirme , A. and Kittleson , D. B. 2004 . A New Electrical Mobility Particle Sizer Spectrometer for Engine Exhaust Particle Measurements . SAE Technical Paper , 10.4271/2004- 01-1341
- Knutson , E. O. and Whitby , K. T. 1975 . Aerosol Classification by Electric Mobility: Apparatus, Theory, and Applications . J. Aerosol Sci. , 6 : 443 – 451 .
- Kousaka , Y. , Okuyama , K. and Adachi , M. 1986 . Determination of Particle-Size Distribution of Ultra-Fine Aerosols Using a Differential Mobility Analyzer . Aerosol Sci. Technol. , 4 : 209 – 225 .
- Kousaka , Y. , Okuyama , K. , Adachi , M. and Mimura , T. 1986 . Effect of Brownian Diffusion on Electrical Classification of Ultrafine Aerosol Particles in Differential Mobility Analyzer . J. Chem. Eng. Japan , 19 : 401 – 407 .
- Li , W. 2006 . Operation of Differential Mobility Analyzers (DMAs). Ph.D. Thesis, , St. Louis , MO : Washington University in St. Louis .
- Li , W. , Li , L. and Chen , D.-R. 2006 . Technical Note: A New Deconvolution Scheme for the Retrieval of the True DMA Transfer Function from the Tandem DMA Data . Aerosol Sci. Technol. , 40 : 1052 – 1057 .
- Martinsson , B. G. , Karlsson , M. N. A. and Frank , G. 2001 . Methodology to Estimate the Transfer Function of Individual Differential Mobility Analyzers . Aerosol Sci. Technol. , 35 : 815 – 823 .
- McMurry , P. H. 2000 . A Review of Atmospheric Aerosol Measurements . Atmos. Environ. , 34 : 1959 – 1999 .
- Mei , F. , Huijing , F. and Chen , D.-R. 2011 . A Cost-Effective Differential Mobility Analyzer (cDMA) for Multiple DMA Column Applications . J. Aerosol Sci. , 42 : 462 – 473 .
- Okuyama , K. , Kousaka , Y. and Motouchi , T. 1984 . Condensation Growth of Ultrafine Aerosol-Particles in a New Particle-Size Magnifier . Aerosol Sci. Technol. , 3 : 353 – 366 .
- Salm , J. 2000 . Diffusion Distortions in a Differential Mobility Analyzer: The Shape of Apparent Mobility Spectrum . Aerosol Sci. Technol. , 32 : 602 – 612 .
- Stolzenburg , M. 1988 . An Ultrafine Aerosol Size Distribution Measuring System. Ph.D. Thesis , St. Paul , MN : University of Minnesota .
- Tammet , H. , Mirme , A. and Tamm , E. 1998 . Electrical Aerosol Spectrometer of Tartu University . J. Aerosol Sci. , 29 : S427 – S428 .
- Tammet , H. , Mirme , A. and Tamm , E. 2002 . Electrical Aerosol Spectrometer of Tartu University . Atm. Res. , 62 : 315 – 324 .
- Wang , J. , McNeill , F. V. , Collins , D. R. and Flagan , R. C. 2002 . Fast Mixing Condensation Nucleus Counter: Application to Rapid Scanning Differential Mobility Analyzer Measurements . Aerosol Sci. Technol. , 36 : 678 – 689 .
APPENDIX
The dimensionless diffusional broadening parameter in EquationEquation (1) can be expressed as
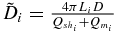




For plug flow
and
where F
γ(ω) is the fraction of flow between ω and γ. Here, L
j + 1 and Lj
are the lengths between the aerosol inlet and the j+1st and the jth sample outlets, respectively. Also whereas Qa
is the aerosol flow rate,
,
,
are the sheath, excess, and sample flow rates of the ith outlet, respectively,
is the total flow through the jth segment, and Qi
(z) is the flow between the trajectory at the axial position z and the outer wall at R
2 (Giamarelou et al. Citation2012) given by
The ratio is the flow fraction between the particle trajectory at (r, z) and the outer wall at R
2 where z is in the jth segment.
For the case of plug flow, the flow fraction is given by
whereas, for the case of Poiseuille flow, it is (Stolzenburg Citation1988)
