Abstract
Black carbon (BC) calibration standards, such as fullerene soot, are routinely used to calibrate single-particle soot photometer (SP2) instruments. Impurities in BC standards create uncertainties in these calibrations, and thus it is desirable to remove non-BC compounds from the aerosol, though removal processes must not significantly alter BC microphysical properties. We present a series of experiments using mobility- and mass-selected fullerene soot particles to assess the performance of a high-temperature denuder system for treating BC prior to SP2 analysis. Particle mass, incandescence, and scattering properties were measured by tandem aerosol particle mass analyzers and an SP2, after thermal treatment at a range of temperatures and residence times (RT). For a longer RT (e.g., ∼6 s at 300°C), monodisperse fullerene soot particles of initial mass 1.4 fg decreased in mass with increasing temperature, by 3% at 300°C to 15% at 600°C. Mass losses were similar for fullerene soot particles of initial mass 10.7 fg. The peak height of the particle laser-induced incandescence (LII) and scattering intensities of the 10.7 fg fullerene soot increased by 7% and 3%, respectively, at 300°C, and by over 15% and 10% at 400°C, possibly due to microphysical changes after heating. When sampling through a 300°C denuder with a particle RT of 2.5 s, the LII intensity of ambient BC particles of initial mass 1.1 fg increased by 8%. In light of these results, denuder temperatures of ∼300°C with 0.4 s ≤ RT ≤ 2.5 s are recommended for SP2 calibration.
Copyright 2013 American Association for Aerosol Research
1. INTRODUCTION
Black carbon (BC) aerosols contribute to the positive radiative forcing (heating) of the atmosphere directly (by efficiently absorbing incident solar radiation), and semidirectly (through cloud burn off; Hansen et al. Citation1997), on both regional and global scales (Intergovernmental Panel on Climate Change 2007). Estimates indicate that BC aerosol may potentially be the second largest contributing factor to global warming (Ramanathan and Carmichael Citation2008; Bond et al. Citation2013). BC particles in ambient air have been shown to be detrimental to human health (Dockery and Pope Citation1994; Laden et al. Citation2000; Slama et al. Citation2007), and as such, BC emissions are controlled and ambient concentrations are closely monitored in many urban and industrial areas throughout the world. In addition, accurate measurements of BC are a prerequisite for an improved assessment of the effects of BC on climate. An assessment of the techniques routinely used to perform ambient BC measurements is provided by Bond et al. (Citation2013).
The mass of refractory BC (hereafter referred to simply as BC) within individual aerosol particles can be quantified using a single-particle soot photometer (SP2; Stephens et al. Citation2003; Baumgardner et al. Citation2004; Schwarz et al. Citation2006), which uses laser-induced incandescence (LII) to measure the intensity of the thermal radiation emitted at the boiling point of BC. The peak of the LII intensity can be used to quantify the mass of BC aerosol on a per-particle basis after calibration (Kondo et al. Citation2011b). The calibration of the SP2 system is typically achieved using commercially available size or mass-selected BC particles of known density (Baumgarder et al. Citation2004), with similar refractive index and particle shape to that of ambient BC. For studies monitoring atmospheric BC, the SP2 calibration should ideally be performed with ambient BC. However, ambient BC calibration particles cannot always be easily obtained in sufficient quantities, and consequently a commercially available standard reference material is generally used as a proxy for ambient BC in SP2 calibrations. The preferred calibration standard among the SP2 community is fullerene soot (Schwarz et al. Citation2006; McMeeking et al. Citation2010; Moteki and Kondo Citation2007, Citation2010; Gysel et al. Citation2011; Kondo et al. Citation2011a,Citationb; Spackman et al. Citation2011; Baumgardner et al. Citation2012), which has shown good agreement with ambient BC in Tokyo for SP2 calibration (accurate to within 10% for particle masses of up to 10 fg; Moteki and Kondo Citation2010) and is insensitive to aerosol generation procedures (Gysel et al. Citation2011).
A significant uncertainty in the absolute calibration of the SP2 results from the presence of additional compounds internally mixed within the BC aerosol. To address this issue, SP2 calibrations have also been performed using ambient BC passed through a thermal-denuder set at 400°C to remove nonrefractory, semivolatile components (Kondo et al. Citation2011b). Kondo et al. (Citation2011b) also reported that when performing BC measurements via other techniques, the use of a 300°C denuder with a particle residence time (RT) 0.4 s led to the evaporation of more than 94% (by volume) of low molecular weight organic species. Gysel et al. (Citation2011) and Laborde et al. (Citation2012) investigated the SP2 sensitivity to fullerene soot downstream of a 400°C thermal-denuder and concluded that, despite the presence of non-BC components in the aerosol, fullerene soot should be used in an unheated form due to observed changes in the response of the SP2. Gysel et al. (Citation2011) and Laborde et al. (Citation2012) interpreted an increase in the incandescence intensity caused by heating BC standards to be a direct result of an increase in the BC mass fraction due to the removal of the presence of non-BC material coexistent in the calibration particles after thermal treatment at 400°C for a few seconds, prior to mass selection. Thickly coated BC particles have been efficiently treated by denuders operating at temperatures as low as 200°C (Ghazi and Olfert Citation2013), however it is common to find such use of thermal-denuders reported without explicitly stating the particle RT in the heated section, even though the importance of this parameter is widely accepted. At present, there is no systematic protocol for the use of a thermal-denuder for BC calibration of the SP2. In order to establish such a protocol, a full characterization of the effects of heating on calibration aerosol to a range of temperatures and for different particle RT is necessary. Although the removal of non-BC compounds from the aerosol is of high priority, it is of paramount importance that such treatment does not change the microphysical or optical properties of the BC particles themselves as the SP2 calibration hinges on the relationship between BC particle mass and LII.
In this article, we present the first simultaneous measurements of changes in BC aerosol particle mass, LII, and scattering intensity, under various denuder conditions in order to systematically examine the effects of different thermal denuder parameters (e.g., temperature, RT). We also present measurements of the response of humic-like substances (HULIS; see the online supplemental materials) to a thermal denuder operated up to 800°C in order to investigate the possibility of removing a substantial fraction of HULIS from ambient BC. The results of this study have enabled us to recommend suitable operational conditions for thermal denuders appropriate for SP2 BC calibrations.
2 EXPERIMENTAL METHODS
2.1 Instrumentation
The commercially available SP2 instrument (Droplet Measurement Technology, Boulder, CO, USA) has been well characterized for measuring BC mass, mixing state, and scattering properties, and its operating principles are described in detail elsewhere (Schwarz et al. Citation2006; Moteki and Kondo Citation2007). Briefly, a jet of aerosol particles is introduced into a chamber in which individual particles are heated to incandescence after intersecting a laser beam of wavelength 1064 nm. The incandescence signal produced by the BC at its boiling point is detected by an avalanche photodiode in both broad- (350–800 nm) and narrow-band (630–800 nm) wavelength regimes. We have used the broadband channel data primarily due to the high signal-noise ratio and its accepted use as the primary channel for BC mass quantification. Nonincandescing (i.e., scattering) material internally mixed with BC particles will evaporate prior to BC incandescence, and an avalanche photodiode and a position-sensitive detector measure the light-scattering signal and the position of the particle in the laser beam, respectively. The sensitivity of the SP2 was found to be stable and consistent within around 10% for fullerene soot particles in the mass range 1–10 fg during calibration (without denuder). SP2 calibration methods using ambient BC and fullerene soot have been described in detail elsewhere (CitationMcMeeking et al. 2010; Gysel et al. Citation2011; Kondo et al. Citation2011a,Citationb). It should be noted that we have not performed a detailed analysis of the waveform for scattering signal data.
In order to generate the aerosol sample, powdered fullerene soot (Alfa Aesar fullerene soot, Lot#FS12S011) was dispersed into purified water (of resistivity 18.2 MΩ cm−1) in a temperature-controlled laboratory, minimizing any possible changes in chemical characteristics. Samples were prepared using a 100:1 ratio of purified water to ethanol in order to disperse particles. All solutions were placed into an ultrasonic bath for 15 min to further aid the dispersion of particles.
shows the experimental setup used for measuring the incandescence and scattering intensities, and resultant mass of BC particles of known initial mass. A polydisperse aerosol sample was generated from the solutions using a nebulizer setup and was drawn through a diffusion dryer (TSI model 3062, TSI Inc., Shoreview, MN) at a standard temperature and pressure (STP) flow rate of 0.3 L min−1 (LPM) to a charge neutralizer (241Am), after which the aerosol sample was mobility-diameter (Dm ) size selected with a differential mobility analyzer (DMA; model 3081, TSI Inc.). The DMA was operated with a sheath to sample flow ratio of 10:1. After the DMA, an aerosol particle mass analyzer (APM; model-302, KANOMAX Japan Inc., Osaka, Japan) was used to further select the quasi-monodisperse particles by mass. The accuracy of the particle diameter and mass classifications were ∼5% based on measurements using standard polystyrene latex spheres (JSR Corp., Tokyo, Japan) with known diameter and mass. Due to the strong effects of both multiple charging and the particle shape factor of fullerene soot, a useful by-product of using both an APM and DMA was the removal of particles comprising multiple charges from the quasi-monodisperse aerosol, resulting in a clearly defined monodisperse sample. Two APMs in series would perform a similar function. The precision of the mass classification was determined by the width of the transfer function of the APM. The width of the transfer function of the APM Model-302 under the operating conditions (i.e., flow rate, rotation speed, applied voltage) used in this study was less than 10% of the center mass. The monodisperse fullerene aerosol sample was then sent through the thermal denuder (described in Section 2.2 and the online supplemental materials) and then the heated sample flow was split between another APM (model-3601, KANOMAX Inc.) and Condensation Particle Counter (CPC; model 3022; TSI Inc.) to count the concentration of particles at each APM voltage (from which average particle mass can be derived), and the aforementioned SP2, for detection.
FIG. 1 Experimental setup for the measurement of (a) fullerene soot particle mass and incandescence/scattering properties after passing through a denuder operated between 25°C and 800°C and (b) for the measurement of ambient BC.
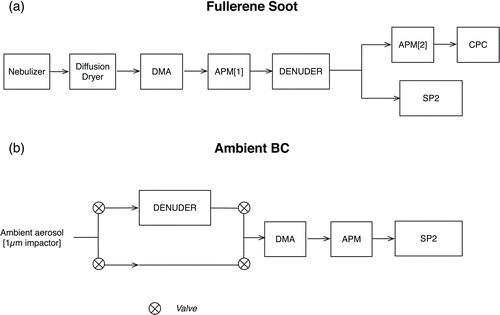
FIG. 2 Plots showing the fullerene soot particle mass response to different LRT-denuder temperatures for particles of initial mass (a) 1.4 fg and (b) 10.7 fg. No discernible differences were observed between 25°C and 300°C (<4%). Up to 400°C, the fullerene soot particle mass remained within 7% of its original value, but by 600°C, the mass distribution has become bimodal (solid circles; dashed gray line denotes fits to different modes).
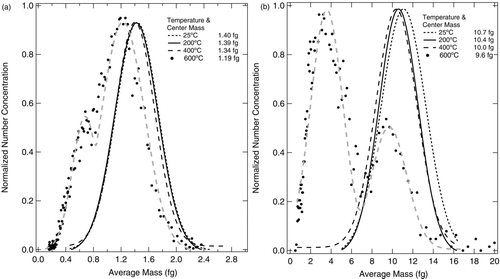
Any changes to particle mass after heating can be directly observed with the tandem APM setup (i.e., the placement of an APM either side of the denuder). The SP2 was used to detect both the peak incandescence and scattering signal intensity of fullerene soot and ambient BC particles of two different masses, M BC (or volume equivalent diameters, Dv , where Dv = (6•M BC/ρ•π)1/3 with an assumed particle density, ρ, of 1.8 g cm−3). The lower limit of the SP2 response to particle scattering is at a volume equivalent diameter of approximately 200 nm for purely scattering, white particles. In order to cover the dominant ambient BC size range observed in Tokyo (as shown by Kondo et al. Citation2011b) fullerene soot particles of mass 1.4 fg (Dm = 150 nm, Dv = 115 nm; close to the peak of the ambient BC mass distribution) and 10.7 fg (Dm = 320 nm, Dv = 220 nm; close to the upper limit typically used for SP2 BC calibration) were selected.
2.2 Thermal-Denuder
After the first APM (APM[1] in ), the monodisperse aerosol samples were passed through a heated inlet, or thermal denuder. When a semivolatile aerosol sample flow is passed through a thermal-denuder, the particles respond by evaporating. The extent to which the particles evaporate provides information about their volatility (Saleh et al. Citation2011). If the RT within the heated section were such that equilibrium is not achieved, kinetic limitations to volatility would result in an underestimation of volatility unless a model is used accounting for evaporation kinetics explicitly (An et al. Citation2007; Riipinen et al. Citation2010; Saleh et al. Citation2011). These points, in addition to model results, are expanded upon in the online supplemental materials.
Two thermal-denuders of different dimensions and materials were used in the laboratory experiments. For simplicity, the two denuders shall be referred to as the long RT denuder (LRT-denuder) and short RT denuder (SRT-denuder). The LRT-denuder comprised a 45-cm-long quartz tube with outer diameter 18 mm and inner diameter 14.5 mm. Quartz was chosen for its inert physicochemical properties, allowing the performance of a denuder at high (∼800°C) temperatures to be assessed. A 35-cm section of the quartz tube was wrapped in a metal wire and insulated in ceramic material. A thermocouple placed alongside the quartz measured the temperature within this insulated area. The SRT-denuder is almost identical to that described by Kondo et al. (Citation2011b). The SRT-denuder is manufactured from stainless steel tubing (SUS304) and is the shorter of the two denuders, with a length of 30 cm. At standard temperature and pressure, the RT in the heated section was calculated to be approximately 12 s for the LRT-denuder and 5 s for the SRT-denuder, for a sample flow of 0.3 LPM, though this will reduce with increased temperature (e.g., at 300°C, the RT reduces to around 6 s and 2.5 s for the LRT and the SRT denuder, respectively).
3 RESULTS AND DISCUSSION
3.1 Changes in Particle Mass
Particles were counted by the CPC after passing through the second APM (APM[2] in Figure 2), which scanned through a range of voltages chosen to maximize the signal of the singly charged particles exiting the DMA. For a given aerosol flow rate, rotation speed, and voltage, the APM transfer function allows the passage of particles comprising a narrow range of masses. Therefore, the APM-derived particle mass (shown on the x-axis in ) represents an average particle mass at each voltage. In order to generate an aerosol number-mass distribution function from these results, an inversion procedure is required. However, we present the data here as uninverted aerosol mass distributions.
shows the concentration-normalized particle number concentration versus average particle mass of fullerene soot, initial mass 1.4 fg, measured by the second APM for four temperature set-points of 25°C, 200°C, 400°C, and 600°C of the LRT-denuder. Data from other temperatures are omitted here for clarity but are summarized in . Generally, average particle mass was observed to decrease with increasing denuder temperature. For denuder temperatures up to 300°C, a loss of particle mass of just 2.8% was observed. This mass loss was observed to increase with increasing denuder temperature (as shown in ). At 500°C and above, the total particle number concentration reduced significantly (by around 70% ± 10%), until almost no particles were detected for a denuder temperature of 800°C.
TABLE 1 APM[2]-derived particle mass, and the percentage changes in mass and LII intensity from unheated 1.4 fg fullerene soot shown in Figures 2a, 3a, and 6a, following treatment with the LRT-denuder between 25°C and 800°C
TABLE 2 APM[2]-derived particle mass, and the percentage changes in mass, LII, and scattering intensity from unheated 10.7 fg fullerene soot shown in Figures 2b, 3b, and 5, following treatment with the LRT-denuder between 25°C and 800°C
It should be noted that the observed mass distribution at 600°C was broader than at lower temperatures and departed from the expected Gaussian function shape, becoming bimodal. As the APM gives no indication of particle composition, the changes to the mass distribution could be due to physical changes to fullerene soot, such as the removal of semivolatile organic compounds (SVOCs) or oxidation processes. Interpretation of the bimodal mass distribution is aided by the analysis of the SP2 results (Section 3.2)
shows the same results but for fullerene soot particles of initial mass 10.7 fg (summarized in ). The trend is consistent with that of the smaller, 1.4 fg particles; average particle mass reduces by around 3% as LRT-denuder temperature is increased up to 300°C. At 400°C, 7% mass is lost from the particles, and at 600°C, the mass distribution again becomes bimodal, with a dominant peak around 70% lower (at 3.43 fg) than the initial selected mass.
FIG. 3 Plots showing the changes in fullerene soot LII intensity after exposure to different LRT-denuder temperatures for particles of initial mass (a) 1.4 fg and (b) 10.7 fg. The LII peak height increases at 400°C (large dashed line) by 17% and 15.4%, respectively, potentially as a small SVOC coating is partitioned to the gaseous phase. At 500°C, the LII intensity reaches its peak at 20.8% and 16%, respectively. This increasing trend is reversed at 600°C (black circles) with only around an 8% increase from unheated samples observed.
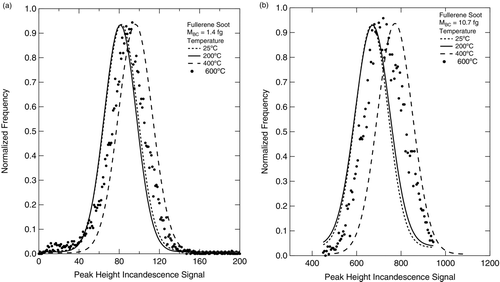
These results show that the particle mass distribution strongly deviates from that at room temperature, with the peak mass shifting toward lower masses. It should again be noted that the particle number concentrations are normalized, and the total particle concentration was significantly lower at 600°C than at 300°C, primarily due to wall-losses. From these effects (particle number loss, bimodal mass distribution), we conclude that denuder temperatures above 500°C are inappropriate for accurate SP2 calibration.
3.2 Changes to Particle Incandescence and Scattering
shows the results of fitting a Gaussian function to the histograms of the incandescence intensity peak heights (normalized for frequency), for the same temperature set-points as in . No discernible difference was observed between 25°C and 200°C in the LII intensity. Increasing the LRT-denuder temperature to 300°C, the LII intensity was 17% higher than that measured at 25°C, perhaps due to the partitioning of non-BC material (e.g., SVOC) to the gaseous phase. The LII intensity remained at this elevated level at 400°C. At 500°C, the relative percentage in LII intensity increased slightly to 21%, but further increasing the temperature of the denuder reversed this trend; at 600°C, the entire range of LII intensity was only 9% higher (denoted by solid black circles). From the simultaneous APM measurements, we know that the particle mass distribution started to become bimodal at this point (as shown in ). Should the particles present in this smaller mode be fullerene soot, they would have an associated LII signal. However, the absence of any smaller mode of reduced LII intensity implies that these smaller particles are non-BC. For denuder temperatures of 700°C, the LII intensity decreased by 23%, and at 800°C, data were sporadic, with a reduction in LII intensity of 67%. The same pattern was observed for changes in the LII intensity for particles of initial mass 10.7 fg, as shown in , which are summarized in .
The calibration of the SP2 depends on the relationship between LII intensity and particle mass. We derived the BC particle mass profiles from the observed LII intensity, using the original calibration data (performed at 25°C) as outlined by Moteki and Kondo (Citation2010). shows the results of normalized Gaussian function fits to the APM-derived uninverted particle mass distribution (solid lines) and SP2 LII intensity converted to mass (dashed lines) at 600°C for the LRT-denuder for particles of initial mass (a) 1.4 fg and (b) 10.7 fg. As the uncertainty and sensitivity of the APM and SP2 differ, the data are presented with arbitrary units and scaled such that the upper-edge of the Gaussian fit functions are approximately coincident. Some variance in the x-axis (mass) is expected, due to the fact that the original calibration was performed at 25°C. shows that non-BC particles populate the smaller mode present in the APM data. shows that the SP2 did not detect any BC particles below ∼8 fg, and since BC particles of masses down to ∼1 fg are easily detectible by the SP2, we conclude that the aerosol population is an external mixture of BC particles (of mass ∼10 fg) and non-BC particles (of mass ∼3.4 fg), which may have been generated from SVOC originally internally mixed with the fullerene soot particles, or caused by the walls of the stainless steel and conductive tubing immediately following the heater.
FIG. 4 Normalized plots showing the results of Gaussian function fits to the APM voltage-derived particle mass (solid lines) and SP2 LII intensity converted to mass (dashed lines) at 600°C for the LRT-denuder for particles of initial mass (a) 1.4 fg and (b) 10.7 fg. The vertical bars represent the 1-sigma standard deviation of the data.
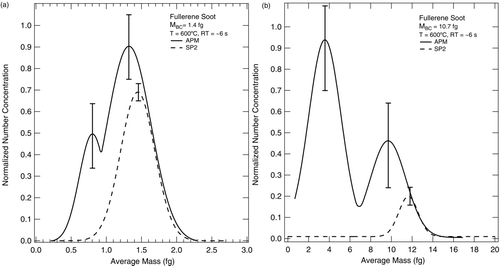
FIG. 5 A graph showing the changes in SP2 scattering intensity of fullerene soot particles of mass 10.7 fg, after treatment with the LRT-denuder at 25°C, 200°C, 400°C, and 600°C.
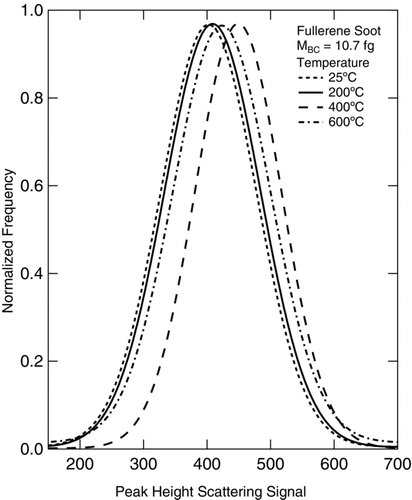
shows the peak height of the scattering intensity from fullerene soot of initial mass 10.7 fg measured by the SP2, for the same denuder temperature set-points. No discernable change in scattering intensity was observed between 25°C and 200°C. At 300°C, the increase in the intensity was minimal (to the order of 3%), as shown in . At a temperature of 400°C, the scattering intensity increased by 11%, but at 600°C, the scattering intensity was almost unchanged from that of the unheated sample. The smaller particles emerging in the APM[2]-derived mass distributions, shown in , are too small to have their scattering properties detected by the SP2 (Dv < 200 nm). This explains the absence of a smaller scattering signal in . Overall, the trends are the same as for the changes in the LII intensity, but are smaller in magnitude. This indicates a physical change to the properties of fullerene soot at temperatures above 600°C.
The percentage change in the incandescence and scattering intensities was recalculated after correcting for the percentage changes in the APM-derived particle mass. For bimodal APM-derived mass distributions, the correction was performed using the center mass of the mode closest to the original mass. shows the results of this analysis for fullerene soot particles of initial mass 10.7 fg, which are also summarized in . Taking changes in mass into consideration, at 300°C, particle incandescence and scattering intensity increased by around 11% and 6%, respectively. At 400°C, the increase in both incandescence and scattering intensity was about 20%. This increasing trend is reversed above 600°C. The vertical bars show the percentage change in the full width at half maximum (FWHM) of the Gaussian function fit, compared with the FWHM at room temperature. The large reduction at 700°C corresponds to the large reduction of LII and scattering intensities.
The same calculation was performed for fullerene soot of initial mass 1.4 fg for the LRT-denuder as shown in (solid circles) and is summarized in . The results show little change to the SP2 LII intensity response up to 200°C, and at 300°C, the LII intensity has increased by around 20%. The mass-corrected LII intensity reached a peak at 500°C, with an increase of around 30% from its unheated value.
3.3 Dependence on Residence Time (RT)
Particle RT in the denuder is hypothesized to impact on the degree of the physical change to the aerosol. Thermal equilibrium is likely to be reached relatively quickly within a denuder, but additional thermal processes, such as oxidation or charring due to overexposure to high temperatures (Kondo et al. Citation2011b), may change the physicochemical characteristics of aerosol particles. This point was demonstrated by the use of the SRT denuder. shows the results of the changes in SP2 LII intensity for fullerene soot of initial mass 1.4 fg as a function of denuder temperature, corrected for the observed changes in particle mass measured by the APM instruments. The results for the LRT-denuder (solid circles) and SRT-denuder (empty circles) are compared in this figure. Between 25°C and 200°C, the changes to the incandescence signal are negligible for both denuders (at ∼1%). At temperatures of 300°C and 400°C, the LII intensity increases for the SRT-denuder were considerably smaller (5% and 12%) than those for the LRT-denuder (around 20%). The SRT-denuder was only operated up to 400°C, as above that temperature, the stainless steel discolors and could be damaged. For denuder temperatures of 400°C and below, no denuder-material dependence was observed for the same RT. These results clearly show particle RT in the denuder to have a significant influence on particle microphysical properties.
3.4 Ambient BC Versus Fullerene Soot
Ambient BC is the source of the absolute calibration for ambient BC measurement (Kondo et al. Citation2011b). However, ambient BC particles are not always available in sufficient quantities for calibration, and so the SP2 community is still searching for a suitable BC standard with similar physicochemical and optical properties (Gysel et al. Citation2011; Laborde et al. Citation2012). Kondo et al. (Citation2011b) reported that ambient BC in Tokyo is mainly derived from vehicular emissions and resembles the physicochemical and optical properties of fullerene soot. However, the authors also found that ambient BC aerosols generally contain volatile and semivolatile organic compounds and suggested the use of a heated inlet for SP2 BC calibration and ambient BC measurements by filter-based instruments. Although Kondo et al. (Citation2011b) used a thermal denuder set at a temperature of ∼300°C for calibration, the performance and response of the SP2 LII signal at various temperatures was not investigated. Here we present data for both ambient BC and fullerene soot for a variety of denuder temperatures.
The ambient mass-size distribution was such that the observed peak in BC mass was around 1.1 fg (Dm = 120 nm, Dv = 105 nm). The instrumental setup for the ambient measurements is shown in . The low ambient BC particle concentrations required long sampling intervals, with each sampling interval lasting approximately 3 h, and thus only the SP2 was used. At the time of this measurement, the second APM was unavailable for use. The layout as shown in is different to the previous experiments in that the polydisperse ambient aerosol was passed through the thermal-denuder before being fed through the DMA and APM for size selection. This changed configuration should mean that the relative BC mass, and thus incandescence intensity, within each 1.1 fg particle will increase with increasing temperature until almost all the semivolatile matter within the aerosol is partitioned to the gaseous phase, as shown in previous works (Gysel et al. Citation2011; Kondo et al. Citation2011b; Laborde et al. Citation2012). This setup is similar to that used by Moteki and Kondo (Citation2010) and Kondo et al. (Citation2011b), i.e., with a denuder upstream of the sizing/mass-classification equipment. The following comparison between unheated and denuded air are approximations in this regard. In order to use inlet temperatures higher than 400°C, a flow rate of 0.7 LPM was used through the LRT-denuder, corresponding to an RT of around 2.5 s at 300°C (equivalent to the particle RT in the SRT-denuder at 300°C). As the ambient size distribution is more susceptible to change than an aerosol generator, the aerosol was alternately fed through either the denuder or an unheated stainless steel line of identical length, via manually operated valves. An estimate of the relative percentage change in LII intensity was calculated from alternate samples. The results from ambient BC experiments are shown in and are summarized in .
FIG. 6 A graph showing the absolute percentage change in LII (full circles) and scattering (empty circles) intensity of fullerene soot (10.7 fg), as a function of temperature. The results have been corrected for changes in APM-derived particle mass. The vertical bars show the percentage change in Gaussian FWHM relative to the sample taken at room temperature.
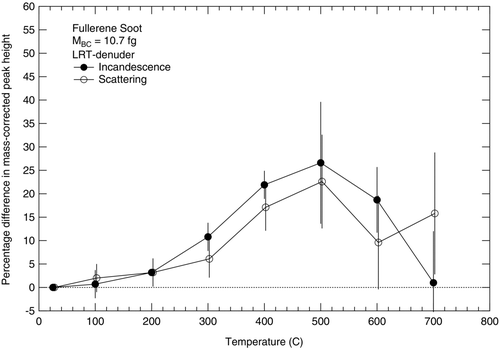
TABLE 3 APM[2]-derived particle mass, and the percentage changes in mass and LII intensity from unheated 1.4 fg fullerene soot as shown in Figure 7a, following treatment with the SRT-denuder between 25°C and 400°C
FIG. 7 The percentage change in LII intensity of (a) fullerene soot particles of mass 1.4 fg and (b) ambient BC of mass 1.1 fg. Solid shapes denote longer residence times, and empty shapes denote shorter residence times. The vertical bars show the percentage change in Gaussian FWHM relative to the sample taken at room temperature.
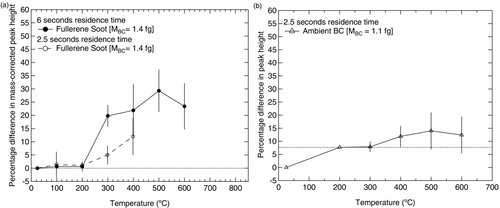
TABLE 4 SP2-derived peak LII intensity percentage change from unheated 1.1 fg ambient BC particles also shown in Figure 7b, after passing through the LRT-denuder between 25°C and 600°C
Unlike the fullerene soot measurements, at 200°C, an increase of 7.7% was observed in the LII intensity. However, as previously mentioned, it is safe to assume that ambient BC is more thickly coated than laboratory-generated fullerene soot and that this increase in LII intensity can be attributed to a higher BC mass-fraction in the treated 1.1 fg mass-selected aerosol particles, as there is no change in LII intensity between 200°C and 300°C. The dotted line at 7.7% in highlights this point. The maximum increase in LII intensity occurs at 500°C (14%) after which the increase in intensity is reduced (to 12% at 600°C). Data were not collected over 600°C, due to the risks associated with setting the denuder to such high temperatures for many hours.
The LII intensity of ambient BC changed little between 200°C and 300°C, and the increase in the LII intensity at 400°C relative to the values at 200°C–300°C was about 5%. Therefore, these results confirm that the use of an SRT-denuder operated at around 300°C is appropriate for calibrations using ambient BC.
3.5 Humic-Like Substances
In order to characterize the inlet for the volatilization of organic matter, the number-size distributions of various HULIS were assessed for loss after heating. It has previously been shown that heated-inlet temperatures above 300°C partition a significant fraction of the HULIS present in ambient BC aerosols to the gaseous phase (Kondo et al. Citation2011b). Ambient BC can be thickly coated with organic material and thus may have a significant impact on the SP2 calibration and derived BC mass concentration. We have therefore tested the effectiveness of a thermal denuder for the removal of HULIS at heater temperatures of 300°C and above. The results and experimental methods are provided in detail in the online supplemental materials and will only be summarized here.
Three different HULIS compounds (Suwanee River humic acid II, Suwanee River fulvic acid II, and Pahokee Peat fulvic acid) were aerosolized and sized-selected using a DMA, and alternately passed through either a standard line or the LRT-denuder at 0.7 STP LPM (equivalent to an RT of around 2.5 s at 300°C), after which the aerosol number size distribution (in the mobility diameter range 10–500 nm) was measured using a wide-range particle spectrometer (Kondo et al. Citation2011b). The number fraction remaining was calculated by dividing the integrated particle number after heating by its corresponding unheated value. Volume fractions were calculated in the same manner, assuming spherical particles.
For the species tested, particles of mobility diameter 150 nm had a remaining volume fraction of between 0.38 and 0.67 following exposure to the LRT-denuder at 300°C. At 400°C, the remaining volume fraction was between 0.21 and 0.55, and by 500°C, a fraction of just 0.12–0.28 remained. At 600°C, the volume fraction remaining was less than 0.01, for all species.
These results demonstrate that, with an RT of less than 2.5 s at 300°C, the efficiency of a thermal-denuder in partitioning HULIS to the gaseous phase is limited. Longer RTs were not tested since the BC measurements were already shown to have a dependence on RT (Section 3.3). For the same RT of 2.5 s, increases in the incandescence and scattering intensities of fullerene soot were less than 8% at 300°C.
4 SUMMARY AND RECOMMENDATION
We have evaluated the performance of a thermal-denuder during fullerene soot and ambient BC calibrations of the SP2 by testing a range of denuder materials, temperatures, and RTs. The impact of each of these parameters was determined by measuring changes in BC mass, LII, and scattering intensity. Unlike previous studies, we have measured changes in the mass of fullerene soot particles after thermal treatment, through the use of a tandem APM system.
The small loss of mass of fullerene soot (less than around 4%) for a denuder temperature of 300°C suggests that for the samples used in this study, the amount of additional compounds mixed with the soot was relatively small. Nevertheless, since the fraction of impurities contained within various batches of fullerene soot is unknown, the use of a thermal denuder is still desirable. The mass of monodisperse fullerene soot particles of initial mass 1.4 and 10.7 fg decreased by 9% and 11%, respectively, for a denuder temperature of 500°C. Above 500°C, the aerosol mass distribution became bimodal with the appearance of a mode populated by non-BC aerosol of lower mass, and the total number concentration was reduced significantly. This implies that an appropriate upper limit denuder temperature for achieving reliable BC calibration without distorting or losing the BC mass is around 400ºC (mass losses ≤7%).
The SP2-derived LII intensity of fullerene soot particles with mass 1.4 fg increased by around 1% and 6% when passed through a denuder at 300°C and 400°C, respectively, for a particle RT of about 2.5 s. The increase in the LII intensity was considerably larger for a denuder with a particle RT of ∼6 s (17% at 300°C and 400°C). Some increase in LII intensity is expected due to a higher relative BC mass in the aerosol particle, though this does not explain the simultaneous increase in the scattering intensity observed with denuder temperatures of 400°C and above. These results suggest that the microphysical or optical properties of BC (e.g., its refractive index) may have changed. Further experiments investigating the relationship between the refractive index of BC particles and temperature (including their natural thermal histories and their generation processes) may further improve the calibration of the SP2.
Ambient BC is typically internally mixed with nonrefractory compounds, as shown by numerous studies. It is necessary to use denuders for calibration using ambient BC, which should be regarded as the primary standard. Measurement of the temperature dependence of the LII intensity for ambient BC were less accurate due to the use of a single APM. However, the increase in the LII intensity of ambient BC particles with 1.1 fg mass was about 4% between denuder temperatures of 200°C and 400°C, similar to the results for fullerene soot.
In light of these results, we recommend denuder temperatures of 300°C ± 50°C, with corresponding particle RT of ≤2.5 s for the calibration of the SP2 for BC measurements. Kondo et al. (Citation2011b) previously showed that the use of a denuder at this temperature with RT of 0.4 s at 300°C could efficiently remove a significant fraction of low molecular weight compounds. However, only around 10%–30% of HULIS was removed using these denuder conditions. We therefore consider these conditions to be a lower limit for successful denuder operations. We have shown in this study that for a particle RT of 2.5 s at 300°C, the volume of HULIS was reduced by around 30%–60% (see the online supplemental materials). Almost 100% of the HULIS was removed at higher temperatures (i.e., 600°C), but such heating results in both low number concentrations (wall losses) and non-BC artifacts. The choice of a denuder temperature of 300°C is therefore a compromise in this regard, in order to remove as much HULIS as possible while not altering the microphysical properties of the BC particles.
In summary, we have derived a range of conditions for SP2 calibration using fullerene soot or ambient BC passed through a denuder and APM; 0.4 ≤ RT ≤ 2.5 s and a denuder temperature of around 300°C allow maximum removal of HULIS without altering the microphysical properties of BC. With these parameters constrained, the uncertainty in the changes in LII intensity by heating should be less than 8%.
AST-MS-2013-026_SUPPLEMENTAL INFORMATION.zip
Download Zip (423.1 KB)Acknowledgments
This work was supported by the Ministry of Education, Culture, Sports, Science, and Technology and Japan Society for the Promotion of Science (MEXT/JSPS) KAKENHI grant number 23221001. This work was also supported by the strategic international cooperative program of the Japan Science and Technology Agency, and the global environment research fund of the Japanese Ministry of the Environment (A-0803 and A-1101), and the GRENE Arctic Climate Change Research Project.
[Supplementary materials are available for this article. Go to the publisher's online edition of Aerosol Science and Technology to view the free supplementary files.]
REFERENCES
- An , W. , Pathak , R. , Lee , B. and Pandis , S. 2007 . Aerosol Volatility Measurement Using an Improved Thermodenuder: Application to Secondary Organic Aerosol . J. Aerosol Sci., , 38 : 305 – 314 .
- Baumgardner , D. , Kok , G. and Raga , G. 2004 . Warming of the Arctic Lower Stratosphere by Light Absorbing Particles . Geophys. Res. Lett., , 31 : L06117 doi: 10.1029/2003GL018883
- Baumgardner , D. , Popovicheva , O. , Allan , J. , Bernardoni , V. , Cao , J. Cavalli , F. 2012 . Soot Reference Materials for Instrument Calibration and Intercomparisons: A Workshop Summary with Recommendations . Atmos. Meas. Tech., , 5 : 1869 – 1887 .
- Bond , T. C. , Doherty , S. J. , Fahey , D. W. , Forster , P. M. , Berntsen , T. DeAngelo , B. J. 2013 . Bounding the Role of Black Carbon in the Climate System: A Scientific Assessment . J. Geophys. Res., , : 2169 – 8996 . doi: 10.1002/jgrd.50171
- Dockery , D. W. and Pope , A.C. 1994 . Acute Respiratory Effects of Particulate air Pollution . Annu. Rev. Public Health, , 15 ( 1 ) : 107 – 132 .
- Ghazi , R. and Olfert , J. 2013 . Coating Mass Dependence of Soot Aggregate Restructuring due to Coatings of Oleic Acid and Dioctyl Sebacate . Aerosol Sci. Technol., , 47 ( 2 ) : 192 – 200. .
- Gysel , M. , Laborde , M. , Olfert , J. S. , Subramanian , R. and Gröhn , A. J. 2011 . Effective Density of Aquadag and Fullerene Soot Black Carbon Reference Materials Used for SP2 Calibration . Atmos. Meas. Tech., , 4 : 2851 – 2858 .
- Hansen , J. , Sato , M. and Ruedy , R. 1997 . Radiative Forcing and Climate Response . J. Geophys. Res., , 102 : 6831 – 6864 .
- Intergovernmental Panel on Climate Change . 2007 . “ Climate Change 2007: The Physical Science Basis. Contribution of Working Group 1 to the 4th Assessment Report of the IPCC ” . Cambridge, UK : Cambridge University Press .
- Kondo , Y. , Matsui , H. , Moteki , N. , Sahu , L. , Takegawa , N. Kajino , M. 2011a . Emissions of Black Carbon, Organic, and Inorganic Aerosols from Biomass Burning in North America and Asia in 2008 . J. Geophys. Res., , 116 : D08204 doi: 10.1029/2010JD015152
- Kondo , Y. , Sahu , L. , Moteki , N. , Khan , F. , Takegawa , N. Liu , X. 2011b . Consistency and Traceability of Black Carbon Measurements Made by Laser-Induced Incandescence, Thermal-Optical Transmittance, and Filter-Based Photo-Absorption Techniques . Aerosol Sci. Technol., , 45 : 295 – 312 .
- Laborde , M. , Mertes , P. , Zieger , P. , Dommen , J. , Baltensperger , U. and Gysel , M. 2012 . Sensitivity of the Single Particle Soot Photometer to Different Black Carbon Types . Atmos. Meas. Tech., , 5 : 1031 – 1043 .
- Laden , F. , Neas , L. M. , Dockery , D. W. and Schwartz , J. 2000 . Association of Fine Particulate Matter from Different Sources with Daily Mortality in Six US Cities . Environ. Health Perspectives, , 108 ( 10 ) : 941 – 947 .
- McMeeking , G. R. , Hamburger , T. , Liu , D. , Flynn , M. , Morgan , W. T. Northway , M. 2010 . Black Carbon Measurements in the Boundary Layer Over Western and Northern Europe . Atmos. Chem. Phys., , 10 : 9393 – 9414 .
- Moteki , N. and Kondo , Y. 2007 . Effects of Mixing State on Black Carbon Measurements by Laser-Induced Incandescence . Aerosol Sci. Technol., , 41 : 398 – 417 .
- Moteki , N. and Kondo , Y. 2010 . Dependence of Laser-Induced Incandescence on Physical Properties of Black Carbon Aerosols: Measurements and Theoretical Interpretation . Aerosol Sci. Technol., , 44 : 663 – 675 .
- Ramanathan , V. and Carmichael , G. 2008 . Global and Regional Climate Changes Due to Black Carbon . Nature Geoscience, , 1 : 221 – 227 .
- Riipinen , I. , Pierce , J. R. , Donahue , N. M. and Pandis , S. N. 2010 . Equilibration Time Scales of Organic Aerosol Inside Thermodenuders: Evaporation Kinetics Versus Thermodynamics . Atmos. Env. , 44 : 597 – 607 .
- Saleh , R. , Shihadeh , A. and Khlystov , A. 2011 . On Transport Phenomena and Equilibration Time Scales in Thermodenuders . Atmos. Meas. Tech., , 4 : 571 – 581 .
- Schwarz , J. , Gao , R. , Fahey , D. and Thomson , D. 2006 . Single-Particle Measurements of Midlatitude Black Carbon and Light-Scattering Aerosols from the Boundary Layer to the Lower Stratosphere . J. Geophys. Res., , 111 : D16207 doi: : 10.1029/2006JD007076
- Slama , R. , Morgenstern , V. , Cyrys , J. , Zutavern , A. , Herbarth , O. Wichmann , H. E. 2007 . Traffic-Related Atmospheric Pollutants Levels During Pregnancy and Offspring's Term Birth Weight: A Study Relying on a Land-Use Regression Exposure Model . Environ. Health Perspectives, , 115 ( 9 ) : 1283 – 1292 .
- Spackman , J. R. , Gao , R. S. , Schwarz , J. P. , Watts , L. A. , Fahey , D. W. Pfister , L. 2011 . Seasonal Variability of Black Carbon Mass in the Tropical Tropopause Layer . Geophys. Res. Lett., , 38 : L09803 doi: 10.1029/2010GL046343.
- Stephens , M. , Turner , N. and Sandberg , J. 2003 . Particle Identification by Laser-Induced Incandescence in a Solid-State Laser Cavity . Applied Optics, , 42 : 3726 – 3736 .