Abstract
The organic macromolecules in total suspended particulate (TSP) are complex and have not been thoroughly investigated. In this article, six composite TSP samples were collected from urban, suburban, and forest sites of Guangzhou. Organic macromolecules in the TSP were separated into humic acids (HA), kerogen and black carbon (KB), and black carbon (BC) by chemical treatment with sodium hydroxide, hydrochloric acid/hydrofluoric acid, and potassium dichromate/sulfuric acid. Note that the BC isolated is part of KB. To obtain sufficient amounts of HA, KB, and BC for subsequent analyses, 1.5 g TSP were used for each sample. The isolated fractions were characterized by elemental analysis, solid-state 13C crosspolarization and magic angle spinning nuclear magnetic resonance spectroscopy, Fourier transformed infrared spectroscopy, stable carbon isotopic ratio, and radiocarbon ratio mass spectrometry. Total carbon content in TSP ranged from 12.8 to 19.0%, and HA, KB, and BC were accounted for 2.39–14.8, 37.4–53.5, and 16.0–32.1% of total carbon, respectively. Isolated HAs were characterized by high H/C and N/C atomic ratios; KB showed high H/C but low N/C atomic ratios; BC was low in H/C, indicating a predominance of aromatic units in its carbon skeleton. The δ13C and radiocarbon data showed that all macromolecular fractions were mixtures of components having biogenic and fossil-fuel origins. The contribution of biogenic material to HA, KB, and BC, estimated by radiocarbon ratios, are 44.2–61.5%, 17.5–24.8%, and 3.3–17.2%, respectively. The atmospheric optical properties and hygroscopicity of kerogen are not known at present and warrant investigation because of the high content of kerogen in TSP.
Copyright 2013 American Association for Aerosol Research
1. INTRODUCTION
Atmospheric particulate matter is composed of diverse chemical compounds, both organic and inorganic, or low molecular weight and macromolecular compounds. It may play an important role in climate change or cause deterioration of air quality (Gill et al. Citation1983; Posfai et al. Citation1998; Tan et al. Citation2009). As a carrier of nutrients and anthropogenic pollutants, atmospheric particulate matter can greatly affect global carbon cycles and control the fate of organic and inorganic pollutants in the atmosphere (Lin et al. Citation2008; Ayrault et al. Citation2010). These effects have sparked interest in elucidation of the chemical composition of airborne particulate matter.
To gain insight into organic compositions, one must isolate organic matter from atmospheric particulate matter. In comparison to organic matter in dust, water, and sediment, large amounts of atmospheric particulate matter must be collected and its organic matter isolated for detailed study of its composition. Because this is difficult, to date most work has concentrated only on low molecular weight organic matter in total suspended particulate (TSP) and PM2.5 that can be easily isolated and detected by the highly sensitive techniques of gas chromatography–mass spectrometry (GC–MS) and high performance liquid chromatography–mass spectrometry (HPLC-MS) (Zappoli et al. Citation1999; Bi et al. Citation2008; Hoyos et al. 2008; Ayrault et al. Citation2010; Ma et al. Citation2010a).
Although the organic macromolecules have been less investigated, the humic-like substances (HULIS) and soot have been studied in detail (Krivácsy et al. Citation2000; Graber and Rudich Citation2006; Duarte et al. 2007; Sannigrahi et al. 2006; Dutkiewicz et al. Citation2009; Song et al. Citation2012). HULIS seems to be formed secondarily by photochemical oxidation, deoxidization, and polymerization of volatile organic compounds in the atmosphere (Hoffer et al. Citation2004) and biomass burning (Lin et al. Citation2010), with average molecular weights in the range of 215–345 Da (Kiss et al. Citation2003). Soot is derived from primary particle emissions (Mukai and Ambe Citation1986; Zappoli et al. Citation1999; Graber and Rudich Citation2006; Blanchard et al. Citation2008). These two macromolecular fractions make up ∼30–50% of the total organic carbon. Therefore, it seems that there must be a fraction of organic macromolecules in the aerosol that has not been isolated and characterized.
It is known that the impact of macromolecular compounds on climate depends greatly on their compositions and on their chemical and physical properties. For example, the water-soluble fraction of HULIS most likely influences the absorption of solar radiation (Hoffer et al. Citation2004) as well as the hygroscopicity and the ability of particles to form Cloud condensation nuclei (CCN) (Dinar et al. Citation2006; Salma et al. Citation2006), whereas BC is a component that may cause positive solar radiative forcing to the Earth (Jacobson Citation2001; Chung and Seinfeld Citation2005). It would certainly be valuable if we could fully identify all fractions of macromolecules in TSP.
Nomenclatures of macromolecules in the aerosol, sediment, and sedimentary rock communities are not always in agreement. Table S1 (available in the online supplementary information) lists discrepancies, whether in the name or the scientific meaning, of macromolecules among different societies. Briefly, we have organic and elemental carbon, brown and black carbon, HULIS and soot in the aerosol community, and fulvic and humic acids, kerogen and black carbon in the geochemistry community working with modern sediment. In sedimentary geology, only one term, “kerogen,” is used to describe insoluble organic matter in shale and carbonate rocks. Notably, the same name may refer to different material in different communities. For examples, black carbon in sediment is similar to EC and not the same as black carbon in aerosols, which is the most strongly light-absorbing component of particulate matter. Kerogen in sedimentary rock includes both kerogen and the black carbon fraction, but the two are recognized separately by the geochemical community working with modern sediment. At present, the name “kerogen” has not been used in atmospheric science.
In general, macromolecules in aerosols have been named according to the determination method, while those in sediment and sedimentary rock have been named in terms of the isolation procedure. The isolation of humic acids (HA), kerogen and black carbon (KB), and BC by treatment with sodium hydroxide (NaOH), hydrochloric acid/hydrofluoric acid (HCl/HF), and potassium dichromate/sulfuric acid (K2Cr2O7/H2SO4) has been widely performed in soils and sediments (Garcette-Lepecq et al. Citation2000; Simpson and Hatcher Citation2004; Li et al. Citation2006) and occasionally in dust (Zhao et al. Citation2011). The characterization of those isolated macromolecules yields a general picture of the chemical structure of organic matter in those samples.
As yet, these isolation and characterization approaches have not been applied to TSP. In the present study, the isolation methods for HAs, KBs, and BCs were evaluated with ambient aerosols. We used 10–15 sheets of glass fiber filter (20.3 cm × 25.4 cm) for each sample, collected from the air of Guangzhou, China, to isolate HAs, KBs, and BCs from TSPs. Total amounts of TSPs were 1–1.5 g for each sample. The large amounts minimized the impact of laboratory and field contamination. The isolated macromolecular fractions were then characterized by elemental analysis (EA), solid-state 13C crosspolarization and magic angle spinning nuclear magnetic resonance spectroscopy (13C-CP/MAS NMR), and Fourier transformed infrared spectroscopy (FTIR). The 13C/12C and 14C/12C ratios were also determined. The results indicated that this isolation procedure is applicable to TSP and data obtained give an insight into complex chemical structure of organic macromolecules in TSP.
2 MATERIALS AND METHODS
2.1 Sample Collection
The TSP samples were collected at three sites in Guangzhou (Figure S1): Wushan (WS) in the Tianhe district, the university town (UT) in the Panyu district, and Maofeng Mountain Forest Park (MF) in the Baiyun district. These sites represent urban, suburban, and forest areas, respectively. To prevent dust and soil particles from entering the sampler, we carefully selected sampling sites to be away from any construction and trafficked road and collected TSP samples at more than 15 m above ground. For a more detailed description of the sampling sites, see the online supplementary information.
A high-volume air sampler with a Whatman glass-fiber filter (GFF, 20.3 cm × 25.4 cm) was used to collect TSP samples at a flow rate of 1.2 m3/min (TianhongIntellient Instruments, Wuhan, China) for 40 h. The filters were precleaned by baking in a furnace at 450°C for 5 h and then stored in aluminum foil within sealed polyethylene bags before use. TSP samples from three sites were collected from 16 June to 30 August 2006 (representing summer) and from 9 January to 20 February 2007 (representing winter). After sampling, filters were wrapped in aluminum foil and stored in a freezer at −40°C until analysis.
TSP concentrations were determined by weighing the filters before and after collection. Before weighing, filters were conditioned in a chamber maintained at 25°C and 50% humidity until constant weight was reached.
We obtained 40 filters for each site and season. Ten to 15 filters collected on different days during the same season were chosen and combined for analysis.
2.2 Fractionation of Macromolecular Organic Matter
The isolation protocol of macromolecular organic matter (including HA, KB, and BC) used was based on that of Song et al. (Citation2002). For detailed procedures, see the online supplementary information and . Briefly, HA was extracted from six to eight sheets of glass-fiber filter with 0.1 M NaOH and precipitated with concentrated HCl (6 mol/L). Dissolved organic carbon (DOC) was measured in this procedure. The isolation of the KB fraction with HCl/HF was performed on the residue following NaOH extraction. Note, the KB isolated is a mixture fraction.
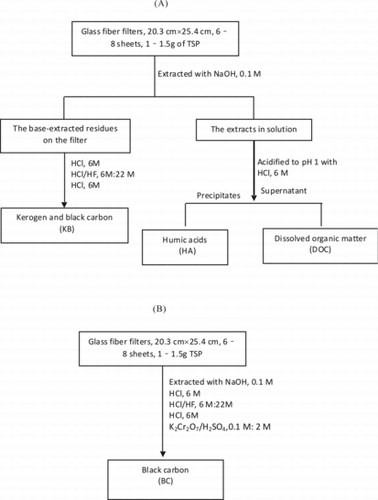
The isolation of BC was carried out with another batch of filters. The—six to eight sheets of glass-fiber filter were treated successively with NaOH, HF/HCl, and K2Cr2O7/H2SO4 solution. The final residue was BC.
To determine the contents of HA, KB, and BC in total organic carbon of TSP, each TSP sample was run twice with the extraction procedure. After isolation, a total of 36 samples were obtained. The amounts of isolated HAs were very low. Therefore, we combined the two HA subsamples from each TSP sample. Finally, 30 samples were subjected to chemical characterization. The mass of each sample was determined on a microbalance (accuracy: ±10 μg).
To check for laboratory contamination, we ran six blank filters through the above procedure. The organic carbon in the blank was negligible.
2.3 EA and DOC Measurements
The total carbon (TC) in TSP and C, H and N contents of the HA, KB, and BC fractions were measured with an Elementar Vario EL III elemental analyzer (Hanau, Germany). Before analysis, the samples were decarbonated with 0.5 M HCl.
The DOC content of aqueous extract with NaOH (after precipitation of HA; ) was measured with an automated, segmented flow analyzer (TOC-VCPH, SHIMADZU) based on a UV-persulfate-oxidation method (Lopes et al. Citation2006). The data reported are the average results of three measurements.
2.4 Solid-State CP/MAS 13C NMR Spectroscopy
The carbon structures of the isolated fractions were determined by solid-state 13C NMR spectroscopy using a Bruker DRX-400 (Switzerland) on a 4-mm probe at 100.63 MHz carbon frequency with a CP/MAS model. The rotor was made of zirconia with a Kel-F cap. The signals were recorded at 1-ms crosspolarization contact time, and approximately 1000 data points were obtained for each sample. The time periods for signal acquisition for a given sample varied from 1 to 10 h.
2.5 FTIR Spectroscopy
The isolated fractions were mixed with potassium bromide at a sample to potassium bromide ratio of 1:10, ground, and pressed into a thin slice. Finally, the prepared sample slice was fitted in the sample chamber and analyzed by FTIR spectroscopy (Bruker-Vector 33, Germany).
2.6 Stable Carbon Isotopic Ratio Measurements (13C/12C)
Stable carbon isotopic ratios (13C/12C) of the HAs, KBs, and BCs were measured on a Finnigan DeltaPlus XL stable isotope mass spectrometer (Thermo America) and are expressed in standard delta notation (‰ = per mil) relative to the Pee Dee Belemnite standard, South Carolina, USA, which is accepted as the international standard. The standard deviation of this method for sextuple runs of a black carbon standard is 0.10‰.
All of the organic fractions were analyzed in duplicate for δ13C values, and the average difference between duplicates was ±0.10‰.
2.7 Radiocarbon Isotopic Ratio Measurements (14C/12C)
The contents of 14C were measured with an accelerator mass spectrometer (AMS) in the State Key Laboratory of Nuclear Physics and Technology (Peking University). The samples were first converted to carbon dioxide (CO2) and purified cryogenically. The purified CO2was then reduced to graphite with hydrogen (H2) over iron (Fe), and its 14C/12C was measured with the AMS system. The 14C activities were determined with respect to the international oxalic acid standard. Final 14C activities are reported as the fraction of modern carbon (fm), which was determined by comparing the observed 14C content in a sample to that in a standard that has 14C content similar to atmospheric 14CO2 levels:
where (14C/12C)obs is the 14C to 12C ratio observed in a sample, and (14C /12C)std is the ratio calculated from the primary standard used during all 14C measurements. fm is then converted to contemporary carbon (fbiogenic) by correcting with a factor of 1.1 for an anthropogenic excess of 14C resulting from nuclear bomb tests during the 1950s and 1960s (Ding et al. Citation2008). No blank corrections were made for determination of the modern fraction of carbon because the organic carbon contribution from the blanks was less than 5% of the sample carbon and had a negligible effect on the calculated fraction of modern carbon.
3 RESULTS AND DISCUSSION
3.1 TC Contents in TSPs and Elemental Compositions of HA, KB, and BC
TSPs have a high content of TC. As shown in , it ranges from 14.5 to 19.0%, values are higher than those of dusts collected in the same areas (4.66 to 6.68%, Zhao et al. Citation2011). TC in the soils of Guangzhou ranged from 0.3 to 2.25% (Zhang Citation2006). In terms of TC content, carbonaceous material in our samples from the soil is not important. The blank run further rules out the possibility of organic contamination in the laboratory, even though sometimes large amounts of fluoride formed during the HCl/HF treatment. The carbon content of the fluoride was always under the detection limit of EA.
Table 1 TC, relative contents of organic fractions, and elemental (C, H, N) compositions
The relative contents of HA, KB, and BC were calculated as the percentages of their carbon mass in TC. The results are summarized in and .
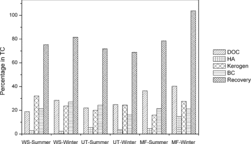
The carbon recovery of the four fractions ranged from 69 to 103% (). Recoveries as low as 69% demonstrate that we lost some carbon in the isolation procedures. The DOC produced in the HCl and HF treatment was not measured because of the large volume of water that was used. Actually, HCl and HF may cleave ether-bound constituents of macromolecules and release water-soluble organic matter to solution. This lost DOC would belong to the kerogen fraction (K).
The loss of fine particles during the decantation of supernatant after base extraction and oxidation of BC particles may also contribute to the low recovery. During the base extraction, dispersed, dark-colored, fine particles, which were presumably BC or K material, were sometimes observed on the surface of the solution, even after centrifugation for several hours, likely because of their surface hydrophobicity and low bulk density. However, elemental analyses of kerogen and BC standards after NaOH and HCl/HF treatments show that such particles contribute <4% of KB in the TC. The loss of fine particles during base extraction may, therefore, be neglected.
Incomplete digestion of K by dichromate may cause uncertainties in the relative contents of KB and BC. To assess the completion of kerogen oxidation, three isolated BC samples were retreated with dichromate/sulfuric acid solution. Less than 6% of TC loss of all isolated BC samples was observed in these repeats, suggesting that K was completely digested and that the oxidation of fine BC particles may be limited during the last step of the procedure.
The recovery as high as 103% may be ascribed to errors caused by formation of large amounts of fluorides in the treatment with HCl/HF. Formation of fluoride minerals during HF/HCl treatment is quite common. Co-existence of HCl with HF in solution can dissolve some minerals but not all of them. This problem sometimes is inevitable when a large amount of silicate (glass filter) is present. The low carbon content in the kerogen fraction demonstrates that the problem did occur in our case ().
The data listed in and indicated that DOC in the aqueous solution after NaOH extraction (after precipitation of HA) accounts for 18.8–40.3% of TC. HULIS lies in this fraction. A reasonable explanation for the higher DOC observed in MF, a forested area, is that volatile organic compounds (VOCs) in this region are dominated by terpenes and isoprenes that may transform more readily into DOC than into other fractions. The HA content is lowest among the four fractions, but we still can see that it reaches 14.8% in winter samples from the forested park. DOC and HA are hydrophilic and hydrophobic combined organic matter with hygroscopic property. The higher content of DOC and HA in aerosols may create more clouds and rainfall in forested areas.
KB constitutes 37.4–53.6% of the TC ( and ), of which K and BC make up 16.0–32.1% and 16.1–27.0% of the TC, respectively. Compared with dust from Guangzhou reported by Zhao et al. (2011), K is lower while BC is more or less similar (41.5–60.8% and 25.2–33.3% of TC, respectively, for K and BC in the dust).
K is defined as solvent-insoluble and is distinct in being hydrophobic. Thus, the atmospheric behavior of K may be similar to that of BC. The fact that K is in the same concentration range as BC reminds us that we should pay the same attention to K as we do to BC.
The meaning of “BC” used in this study is not the same as it is in atmospheric chemistry. It comprises char and soot (Table S1). The BC contents presented in and are comparable to those reported in the literature. For instance, BC was reported to account for 4.14–17.3% of TC in Pearl River Delta sediments and soil in China (Ran et al. Citation2007; Sun et al. Citation2008). The relatively high BC in our samples indicates that anthropogenic input can be an important source of organic matter in TSP from industrialized regions.
The elemental compositions of organic substances may yield bulk chemical-structure information. The C, H, and N contents of HA, KB, and BC of our samples are listed in . The oxygen content could not be obtained because of insufficient sample or severe interference by oxygen released from fluoride minerals during the EA analysis.
clearly shows that the H/C atomic ratios change in the order K >HA > BC. HAs and Ks isolated from the WS, UT, and MF sites have H/C atomic ratios of 0.84–1.03 and 0.89–3.65, respectively. These values are similar to or higher than those reported in soils and sediments (Mafra et al. Citation2007). The BC fractions isolated from WS, UT, and MF are also similar in terms of atomic H/C ratios that are 0.13–0.28, 0.09–0.22, and 0.11–0.30, respectively. These ratios are slightly lower than those of BC in soil and sediment and also lower than those of modern BC and standard reference BC (Song et al. Citation2002; Hammes et al. Citation2008), but they are similar to those of charcoal plant debris (Goldberg Citation1985).
The nitrogen contents and N/C ratios listed in indicate that most of the nitrogen was contained in the HA fraction, with very little nitrogen present in the KB and BC fractions. Considering the loss of kerogen in the HF/HCl treatment, we assume that the low N/C in KB is due to the selective loss of nitrogen compounds in the isolation process.
The variations of the elemental contents of C, H, and N in the macromolecular fractions in TSP can be explained by the source and transformation process of organic matter. HA and K fractions in TSP may be derived mainly from a primary biogenic sources and photochemical oxidation of VOCs that lead to high H/C and N/C ratios. However, BC is predominantly emitted from combustion of fossil fuels and biomass, which have low H/C and N/C ratios.
Table 2 Percentage of carbon groups in the solid 13C NMR spectra
Zhao et al. (2011) reported atomic ratios of H/C and N/C in the dust from Guangzhou. It seems that the H/C atomic ratio of HA in dust is higher than that in TSP, while other fractions are similar in H/C and N/C. The TSP contains large amounts of DOC in comparison with dust. When TSP is transformed into dust, DOC may age into HA. This is a possible explanation for the differencesin H/C and N/C ratios between HAs in TSP and dust.
3.2 CP/MAS 13C NMR Spectroscopy Analysis
Typical 13C NMR spectra of the KB and BC fractions are shown in . For a comparative study, the resonant peaks were classified and grouped into seven catalogues. Peaks at 0–45, 45–63, 63–93, 93–148, 148–165, 165–187, and 187–220 ppm are aliphatic, methoxyl, hydroxyl, aromatic, oxygen-substituted aromatic, carboxylic, and carbonyl carbons, respectively. The areas of these peaks were integrated for calculation of relative percentage and aromaticity. The results are listed in .
Only five fractions isolated from TSPs were analyzed by NMR (). The others (especially for HAs) had insufficient sample quantities for NMR analyses. The spectra of the BC fractions are characterized by relatively high oxygen-substituted aromatic and carboxyl carbon contents with aromaticity of 34.6–62.7%. A small amount of carbohydrate carbon was also present in the BC spectra. These characteristics differ slightly from those of modern BC derived from the combustion of fossil fuels, which completely lacks carbohydrate carbon (Petsch et al. Citation2001). The presence of this type of carbon in the BC fraction also indicates that some parts of the BC are from biomass burning. The higher carbohydrate carbon in UT-W-BC was further evidence that biomass burning was also a major contributor of BC in suburban areas.
The NMR spectra of the KB fractions, shown in and , are characterized by alkyl-substituted aromatic and hydroxylcarbons with aromaticity fractions of 25.5–36.8%. If aromatic and aliphatic carbon form the basis of the BC structure, the hydroxyl or methoxylcarbons in KB may be important for the structure of K. The presence of this structure is not unexpected because K in the TSP may partially comprise oxidation products formed by intermolecular combination processes and contain its precursors such as HULIS and HA. Multihydroxyl and ether functional groups are characteristic of oxidation products from VOCs (Monod et al. Citation2005).
3.3 FTIR Spectroscopy Analysis
The FTIR analysis of HA, KB, and BC may provide information about the chemical structure, which is complementary to the 13C NMR results. shows the FTIR spectra of selected samples, indicating that there are slight but distinct differences among the samples.
The broad band O-H peak (3600–3200 cm−1) can be attributed to hydroxyl and amide stretching vibration absorption. All fractions show this absorption peak at 3400–3600 cm−1. The relatively high wave number in the HA fraction demonstrates weak inter- or intramolecular interactions of O-H (). This coincides with the lower molecular weight of HA than that of KB and BC.
The absorption peaks at 2980 cm−1 and 2862 cm−1 can be identified as asymmetric and symmetric stretching vibrations of alkyl compounds. All samples contain these absorption peaks; however, KB and BC have lower and HAs have higher absorption at 2980 cm−1 and 2862 cm−1, indicating more aliphatic entities in HA ().
The peak at 1700 cm−1 is the C&dbnd;O stretching vibration associated with ketones, aromatic carbon, and aliphatic acids. The peak is much weaker in KB and BC than in HAs. The higher C&dbnd;O group in HAs indicates that HAs have more contribution of oxidation products from photochemical reactions.
The band at 1,600 cm−1 is attributed to the C&dbnd;C stretching vibration of aromatic rings and is strongest in KB and BC samples, reflecting the essential aromatic structure in KB and BC. The absorption peak at 1454 cm−1 is mainly due to CH2 and CH3 asymmetric bending vibrations. The absorption peak in BC at 1400 cm−1 can be attributed to C-H bending vibrations of C-(CH3)2 or C-(CH3)3 groups, indicating that there were branched-chain alkanes in the BC samples. The origin of this structure in BC is unknown.
The 1000–1100 cm−1 band is indicative of ether-bond or sulfate absorption (Maria et al. Citation2002). The intensification of this peak observed in the HA fraction means that HA contains more C-O or C-S bearing compounds. These features are in close agreement with the origin of HA partially from photochemical reaction of VOCs.
Overall, the FTIR spectra indicate that HA is enriched in hydroxyl, carbonyl, carboxyl, and aliphatic groups and is relatively lacking in aromatic groups, while KB and BC are enriched in aromatic and hydroxyl groups and have lower amounts of other groups. These features are in close agreement with the chemical structures of HA, KB, and BC elucidated by EA and NMR.
3.4 δ13C Values of Macromolecular Fractions
The δ13C values were measured for individual macromolecular organic fractions in TSP samples collected from WS, UT, and MF to investigate variations in the isotopic compositions in different seasons and at different sampling sites. Differences in sources and formation pathways may be reflected in the δ13C values as HAs and K may derive from a biogenic primary source and oxidation products of VOCs (Fisseha et al. 2009; Zhao et al. Citation2011), while BC is mainly emitted from fossil-fuel and biomass combustion. Theoretically, severe photochemical processes may cause heavy isotopic composition of organic matter in TSP (Ma et al. Citation2010b).
The δ13C values of HAs, KBs, and BCs varied from −26.6‰ to −26.0‰, −26.4‰ to −23.9‰, and −26.3‰ to −23.3‰ with mean values of −26.2‰, −25.4‰ and −24.8‰, respectively ( and Table S2). The order of δ13C values, BC≈KB>HA, seems generally to reflect the differences in combustion and photochemical processes.
HAs fall in a narrow range, indicating that they are derived mainly from primary or secondary biogenic sources. C3 plants, thriving in tropical areas, generally yield VOCs and plant debris with δ13C around −26‰, supporting this assumption (Simoneit Citation1997; Ma et al. Citation2010b).
KB and BC have relatively broad ranges of δ13C values. This indicates that they derive from the mixing of sources, e.g., biogenic and anthropogenic. δ13C values of anthropogenic organic matter from fossil fuel combustion range from −24‰ to −25‰ (Zhang et al. Citation2003; Kelly et al. Citation2005). From the biogenic and anthropogenic δ13C values, we can see that KBs have two sources while BCs are mainly from the combustion of biomass and fossil fuels.
Seasonal variation of δ13C values for a given fraction is not very pronounced. We speculate that the mixing processes may give relatively constant δ13C values. Taking HA and K fractions as examples, large emissions of biogenetic VOCs in summer will yield light δ13C values. This effect may be compensated for by strong photochemical degradation in summer, which will theoretically lead to heavier δ13C values. Overall, no obvious seasonal change was observed.
3.5 14C/12C Ratio Measurements of Macromolecular Fractions
Radiocarbon (14C) is a unique indicator of contemporary carbonaceous material because atmospheric carbon particles have two primary origins, contemporary and fossil carbon sources (Masiello et al. Citation2002; Bench and Herckes Citation2004; Gelencsér et al. Citation2007; Zencak et al. 2007). It also helps us to understand the distribution, transformation, and fate of organic pollutants in the environment. Therefore, radiocarbon analysis was employed to investigate the origin of the macromolecular organic matters isolated from the TSPs ().
Table 3 Δ14C(‰) of macromolecular organic matters in TSP and the contribution of contemporary carbon (fbiogenic)
A simple isotopic mass-balance equation, based on the Δ14C data, was applied to quantify the fractional contributions of biogenic carbon (fbiogenic) and fossil-fuel (ffossil = 1-fbiogenic) sources to the HA, KB, and BC isolates (Reddy et al. Citation2002; Mandalakis et al. Citation2005):
where Δ14Csample is the measured radiocarbon content of the isolated HAs, KB, and BC in TSP and Δ14Cfossil is the characteristic radiocarbon abundance in fossil material (−1000‰). fbiogenic and 1-fbiogenic are the fractions of HAs, KB, and BC derived from biogenic sources and fossil-fuel sources, respectively.
The calculations show that fbiogenic of HAs was 46–61% in summer and 55–65% in winter. Biogenic VOC is thought to be generally more reactive than anthropogenic VOC (Chameides et al. Citation1988), and larger loss of biogenic VOCs is expected. However, the biogenic sources are still dominant in sources of HAs from the three sampling sites. Indeed, biogenic species of VOCs are abundant in the air and have been well studied in the past; they include nonmethane hydrocarbons and other low molecular weight compounds. Guenther et al. (Citation1995) and Klouda et al. (Citation1996) also suggest that primary emissions of poorly characterized, oxygenated VOCs, such as methanol, formaldehyde, 3-hexen-1-ol, and others, may be of major importance in biogenic emissions inventories. Such species are generally more soluble than ordinary hydrocarbons. Oxidation products of these biogenic VOCs may possibly give rise to HA.
The contribution of biogenic material to K is much less, ranging from 18.8 to 37.7%. It seems that the less reactive fossil fuel-related primary or secondary material prefers to transform to the K fraction. As for the BC fraction, biogenic sources seem not to be important. They account for only 3.46 to 18.1% of total BC. Overall, we can conclude that fossil-fuel-related sources dominate the insoluble organic substances in TSPs from the air of Guangzhou. These results coincide with heavy air pollution in Guangzhou but are inconsistent with those of organic carbon in TSPs reported by Szidat et al. (Citation2004), who found a 51–80% biogenic contribution to organic carbon-particle formation in Zurich, Switzerland.
The explanation of the Δ14C data above agrees with that of the δ13C data. shows that a high percentage of fbiogenic corresponds to negative δ13C values, which are derived mainly from biogenic sources.
4 CONCLUSIONS
Operationally-defined fractions of organic macromolecules, such as humic acid, kerogen, and black carbon, in total suspended particulate can be isolated by chemical treatments with NaOH, HCl/HF, and K2Cr2O7/H2SO4. We found that contents of HA, KB, and BC are 2.39–14.8, 37.4–53.5, and 16.0–32.1% of total organic carbon in the TSP, respectively. These macromolecular fractions were mixtures of components having biogenic and anthropogenic origins as indicated by H/C and N/C ratios, δ13C values and radiocarbon (Δ14C) data. However, humic acids are dominated by materials of biogenic origin, while KB and BC are composed of more fossil-fuel-related materials. Kerogen is a new isolated organic macromolecule in TSP with the same concentration as BC. It merits further investigation because of its possible impacts on climate. The one of the most important investigation in the future is to confirm whether kerogen presents in the fine particles, such as PM2.5 and PM1. It will give us further evidence for origin of kerogen in the air, and certainly has more scientific significance than the researches on TSP.
Supplemental_Information.zip
Download Zip (62 KB)REFERENCES
- Ayrault, S., Senhou, A., Moskura, M., and Gaudry, A. (2010). Atmospheric Trace Element Concentrations in Total Suspended Particles Near Paris, France. Atmos. Environ., 44:3700–3707.
- Bench, G., and Herckes, P. (2004). Measurement of Contemporary and Fossil Carbon Contents of PM2.5 Aerosols: Results from Turtleback Dome, Yosemite National Park. Environ. Sci. Technol., 38:2424–2427.
- Bi, X., Simoneit, B.R. T., Sheng, G., Ma, S., and Fu, J. (2008). Composition and Major Sources of Organic Compounds in Urban Aerosols. Atmos. Res., 88:256–265.
- Blanchard, C.L., Hidy, G.M., Tanenbaum, S., Edgerton, E., Hartsell, B., and Jansen, J. (2008). Carbon in Southeastern US Aerosol Particles: Empirical Estimates of Secondary Organic Aerosol Formation. Atmos. Environ., 42:6710–6720.
- Chameides, W.L., Lindsay, R.W., Richardson, J., and Kiang, C.S. (1988). The Role of Biogenic Hydrocarbons in Urban Photochemical Smog—Atlanta as a Case-Study. Science, 241:1473–1475.
- Chung, S.H., and Seinfeld, J.H. (2005). Climate Response of Direct Radiative Forcing of Anthropogenic Black Carbon. J. Geophys. Res.-Atmos. 110(D11), doi: D11102 10.1029/2004jd005441.
- Dinar, E., Taraniuk, I., Graber, E.R., Katsman, S., Moise, T., Anttila, T., et al. (2006). Cloud Condensation Nuclei Properties of Model and Atmospheric HULIS. Atmos. Chem. Phys., 6:2465–2481.
- Ding, X., Zheng, M., Edgerton, E.S., Jansen, J.J., and Wang, X. (2008). Contemporary or Fossil Origin: Split of Estimated Secondary Organic Carbon in the Southeastern United States. Environ. Sci. Technol., 42:9122–9128.
- Duarte, R.M. B. O., Santos, E.B. H., Pio, C.A., and Duarte, A.C. (2007). Comparison of Structural Features of Water-Soluble Organic Matter from Atmospheric Aerosols with Those of Aquatic Humic Substances. Atmos. Environ., 41:8100–8113.
- Dutkiewicz, V.A., Alvi, S., Ghauri, B.M., Choudhary, M.I., and Husain, L. (2009). Black Carbon Aerosols in Urban Air in South Asia. Atmos. Environ., 43:1737–1744.
- Fisseha, R., Saurer, M., Jaggi, M., Siegwolf, R.T. W., Dommen, J., Szidat, S., et al. (2009). Determination of Primary and Secondary Sources of Organic Acids and Carbonaceous Aerosols Using Stable Carbon Isotopes. Atmos. Environ., 43:431–437.
- Garcette-Lepecq, A., Derenne, S., Largeau, C., Bouloubassi, I., and Saliot, A. (2000). Origin and Formation Pathways of Kerogen-Like Organic Matter in Recent Sediments off the Danube Delta (Northwestern Black Sea). Org. Geochem., 31:1663–1683.
- Gelencsér, A., May, B., Simpson, D., Sanchez-Ochoa, A., Kasper-Giebl, A., Puxbaum, H., et al. (2007). Source Apportionment of PM2.5 Organic Aerosol Over Europe: Primary/Secondary, Natural/Anthropogenic, and Fossil/Biogenic Origin. J. Geophys. Res.-Atmos. 112.
- Gill, P.S., Graedel, T.E., and Weschler, C.J. (1983). Organic Films on Atmospheric Aerosol-Particles, Fog Droplets, Cloud Droplets, Raindrops, and Snowflakes. Rev.Geophys., 21:903–920.
- Goldberg, D.E. (1985). Black Carbon in the Environment: Properties and Distribution. John Wiley & Sons, New York.
- Graber, E.R., and Rudich, Y. (2006). Atmospheric HULIS: How Humic-Like are They? A Comprehensive and Critical Review. Atmos. Chem. Phys., 6:729–753.
- Guenther, A., Hewitt, C.N., Erickson, D., Fall, R., Geron, C., Graedel, T., et al. (1995). A Global Model of Natural Volatile Organic-Compound Emissions. J. Geophys. Res. Atmos., 100 (D5):8873–8892.
- Hammes, K., Smernik, R.J., Skjemstad, J.O., and Schmidt, M.W. I. (2008). Characterisation and Evaluation of Reference Materials for Black Carbon Analysis Using Elemental Composition, Colour, BET Surface Area and C-13 NMR Spectroscopy. Appl. Geochem., 23:2113–2122.
- Hoffer, A., Kiss, G., Blazso, M., Gelencsér, A. (2004). Chemical Characterization of Humic-Like Substances (HULIS) Formed from a Lignin-Type Precursor in Model Cloud Water. Geophys. Res. Lett., 31(L06115):1–4.
- Hoyos, A., Cobo, M., Aristizabal, B., Cordoba, F., and de Correa, C.M. (2008). Total Suspended Particulate (TSP), Polychlorinated Dibenzodioxin (PCDD) and Polychlorinated Dibenzofuran (PCDF) Emissions from Medical Waste Incinerators in Antioquia, Colombia. Chemosphere, 73:S137–S142.
- Jacobson, M.Z. (2001). Strong Radiative Heating Due to the Mixing State of Black Carbon in Atmospheric Aerosols. Nature, 409:695–697.
- Kelly, S.D., Stein, C., and Jickells, T.D. (2005). Carbon and Nitrogen Isotopic Analysis of Atmospheric Organic Matter. Atmos. Environ., 39:6007–6011.
- Kiss, G., Tombacz, E., Varga, B., Alsberg, T., and Persson, L. (2003). Estimation of the Average Molecular Weight of Humic-Like Substances Isolated from Fine Atmospheric Aerosol. Atmos. Environ., 37:3783–3794.
- Klouda, G.A., Lewis, C.W., Rasmussen, R.A., Rhoderick, G.C., Sams, R.L., Stevens, R.K., et al. (1996). Radiocarbon Measurements of Atmospheric Volatile Organic Compounds: Quantifying the Biogenic Contribution. Environ. Sci. Technol., 30:1098–1105.
- Krivácsy, Z., Kiss, G., Varga, B., Galambos, I., Sarvári, Z., Gelencsér, A., et al. (2000). Study of Humic-Like Substances in Fog and Interstitial Aerosol by Size-Exclusion Chromatography and Capillary Electrophoresis. Atmos. Environ., 34:4273–4281.
- Li, L., Jia, W., Peng, P., Sheng, G., Fu, J., and Huang, W. (2006). Compositional and Source Characterization of Base Progressively Extracted Humic Acids Using Pyrolytic Gas Chromatography Mass Spectrometry. Appl. Geochem., 21:1455–1468.
- Lin, C.W., Yeh, J.F., and Kao, T.C. (2008). Source Characterization of Total Suspended Particulate Matter Near a Riverbed in Central Taiwan. J. Hazard. Mat., 157:418–422.
- Lin, P., Engling, G., and Yu, J.Z. (2010). Humic-Like Substances in Fresh Emissions of Rice Straw Burning and in Ambient Aerosols in the Pearl River Delta Region, China. Atmos. Chem. Phys. 10:6487–6500.
- Lopes, C.B., Abreu, S., Valega, M., Duarte, R., Pereira, M.E., and Duarte, A.C. (2006). The Assembling and Application of an Automated Segmented Flow Analyzer for the Determination of Dissolved Organic Carbon Based on UV-Persulphate Oxidation. Anal. Let., 39:1979–1992.
- Ma, S., Peng, P., Song, J., Bi, X., Zhao, J., He, L., et al. (2010a). Seasonal and Spatial Changes of Free and Bound Organic Acids in Total Suspended Particles in Guangzhou, China. Atmos. Environ., 44:5460–5467.
- Ma, S., Peng, P., Song, J., Zhao, J., He, L., Sheng, G., et al. (2010b). Stable Carbon Isotopic Compositions of Organic Acids in Total Suspended Particles and Dusts from Guangzhou, China. Atmos. Res., 98:176–182.
- Mafra, A.L., Senesi, N., Brunetti, G., Miklos, A.A. W., and Melfi, A.J. (2007). Humic Acids from Hydromorphic Soils of the Upper Negro river Basin, Amazonas: Chemical and Spectroscopic Characterisation. Geoderma, 138:170–176.
- Mandalakis, M., Gustafsson, O., Alsberg, T., Egeback, A.L., Reddy, C.M., Xu, L., et al. (2005). Contribution of Biomass Burning to Atmospheric Polycyclic Aromatic Hydrocarbons at Three European Background Sites. Environ. Sci. Technol., 39:2976–2982.
- Maria, S.F., Russell, L.M., Turpin, B.J., and Porcja, R.J. (2002). FTIR Measurements of Functional Groups and Organic Mass in Aerosol Samples Over the Caribbean. Atmos. Environ., 36, 5185–5196.
- Masiello, C.A., Druffel, E.R. M., and Currie, L.A. (2002). Radiocarbon Measurements of Black Carbon in Aerosols and Ocean Sediments. Geochim. Cosmochim. Acta, 66:1025–1036.
- Monod, A., Poulain, L., Grubert, S., Voisin, D., and Wortham, H. (2005). Kinetics of OH-Initiated Oxidation of Oxygenated Organic Compounds in the Aqueous Phase: New Rate Constants, Structure-Activity Relationships and Atmospheric Implications. Atmos. Environ., 39:7667–7688.
- Mukai, H., and Ambe, Y. (1986). Characterization of a Humic Acid-Like Brown Substance in Airborne Particulate Matter and Tentative Identification of its Origin. Atmos. Environ., 20:813–819.
- Petsch, S.T., Smernik, R.J., Eglinton, T.I., and Oades, J.M. (2001). A Solid State C-13-NMR Study of Kerogen Degradation During Black Shale Weathering. Geochim. Cosmochim. Acta, 65:1867–1882.
- Posfai, M., Xu, H.F., Anderson, J.R., and Buseck, P.R. (1998). Wet and Dry Sizes of Atmospheric Aerosol Particles: An AFM-TEM Study. Geophys. Res. Lett., 25:1907–1910.
- Ran, Y., Sun, K., Yang, Y., Xing, B., and Zeng, E. (2007). Strong Sorption of Phenanthreneby Condensed Organic Matter in Soils and Sediments. Environ. Sci. Technol., 41:3952–3958.
- Reddy, C.M., Pearson, A., Xu, L., McNichol, A.P., Benner, B.A., Wise, S.A., et al. (2002). Radiocarbon as a Tool to Apportion the Sources of Polycyclic Aromatic Hydrocarbons and Black Carbon in Environmental Samples. Environ. Sci. Technol., 36:1774–1782.
- Sannigrahi, P., Sullivan, A.P., Weber, R.J., and Ingall, E.D. (2006). Characterization of Water-Soluble Organic Carbon in Urban Atmospheric Aerosols Using Solid-State C-13 NMR Spectroscopy. Environ. Sci. Technol., 40: 666–672.
- Salma, I., Ocskay, R., Varga, I., and Maenhaut, W. (2006). Surface Tension of Atmospheric Humic-Like Substances in Connection with Relaxation, Dilution, and Solution pH. J. Geophys. Res. Atmos., 111(D23), doi: 10.1029/2005jd007015.
- Simoneit, B.R. T. (1997). Compound-Specific Carbon Isotope Analyses of Individual Long-Chain Alkanes and Alkanoic Acids in Harmattan Aerosols. Atmos. Environ., 31:2225–2233.
- Simpson, M.J., and Hatcher, P.G. (2004). Overestimates of Black Carbon in Soils and Sediments. Naturwissenschaften, 91:436–440.
- Song, J., He, L., Peng, P., Zhao, J., and Ma, S. (2012). Chemical and Isotopic Composition of Humic-Like Substances (HULIS) in Ambient Aerosols in Guangzhou, South China. Aerosol Sci. Technol., 46:533–546.
- Song, J., Peng, P., and Huang, W. (2002). Black Carbon and Kerogen in Soils and Sediments. 1. Quantification and Characterization. Environ. Sci. Technol., 36:3960–3967.
- Sun, X., Peng, P., Song, J., Zhang, G., and Hu, J. (2008). Sedimentary Record of Black Carbon in the Pearl River Estuary and Adjacent Northern South China Sea. Appl. Geochem., 23:3464–3472.
- Szidat, S., Jenk, T.M., Gaggeler, H.W., Synal, H.A., Fisseha, R., Baltensperger, U., et al. (2004). Radiocarbon (C-14)-Deduced Biogenic and Anthropogenic Contributions to Organic Carbon (OC) of Urban Aerosols from Zurich, Switzerland Atmos. Environ., 38:4035–4044.
- Tan, J., Duan, J., Chen, D., Wang, X., Guo, S., Bi, X., et al. (2009). Chemical Characteristics of Haze During Summer and Winter in Guangzhou. Atmos. Res., 94:238–245.
- Zappoli, S., Andracchio, A., Fuzzi, S., Facchini, M.C., Gelencsér, A., Kiss, G., et al. (1999). Inorganic, Organic and Macromolecular Components of Fine Aerosol in Different Areas of Europe in Relation to Their Water Solubility. Atmos. Environ., 33:2733
- Zencak, Z., Elmquist, M., and Gustafsson, O. (2007). Quantification and Radiocarbon Source Apportionment of Black Carbon in Atmospheric Aerosols Using the CTO-375 Method. Atmos. Environ., 41:7895–7906.
- Zhao, J., Peng, P., Song, J., Ma, S., Sheng, G., and Fu, J. (2011). Characterization of Macromolecular Organic Matter in Atmospheric Dust from Guangzhou, China, Atmos. Environ., 45:5612–5620.
- Zhang, C., Chen, F., and Jin, M. (2003). Study on Modern Plant C-13 in Western China and Its Significance. Chinese J. Geochem., 22:97–106.
- Zhang, S. (2006). The Spatial Distribution of PCDDs/PCDFs of Soilsand Sediments in the Pearl River Delta of China and Source Identification . Ph.D. thesis, the Graduate School of The Chinese Academy of Sciences, Beijing.