ABSTRACT
The properties and impacts of aggregated aerosol particles (i.e., soot, metal oxide fumes) depend on their morphology, as characterized by fractal dimension, prefactor, and primary particle diameter. The morphology may be measured directly by time-consuming ex situ microscopy or rapid but indirect in situ methods. Previously, it was found that particle mass and mobility measurements could be used for the estimation of the primary particle diameter of zirconia aggregates, using plausible assumptions related to the fractal structure (specifically, prefactor and exponent
). Since the formation and growth of zirconia aggregates are different from carbon soot, here we compare primary particle diameters measured directly from transmission electron microscopy analysis of soot particles with the diameters estimated from mass–mobility measurements. Performing extensive measurements on soot emissions from two reciprocating engines over a range of operating conditions, we found that there are no universal values of
and
that can be used for all conditions. However, new optimized values of
and
are estimated here for soot particles. The variation of the primary particle diameter with particle size is also taken into consideration and is shown to be essential to obtain physically realistic results. Using optimized values of
and
, the average primary particle sizing error is reduced for all soot types. This suggests that with some calibration, in situ sizing of the primary particle diameter, using mass and mobility measurements, can provide useful accuracy.
Copyright © 2016 American Association for Aerosol Research
EDITOR:
Introduction
Soot particles are typically aggregated structures composed of primary particles, and have negative impacts on human health (Maynard and Kuempel Citation2005; Franchini and Mannucci Citation2012) and environment (Jacobson Citation2010). Soot properties depend nonlinearly on the primary particle diameter, : for example, soot mass and surface area (important parameter in toxicity studies) are proportional to
and
, whereas scattering and absorption (Sorensen Citation2001) are proportional to
and
, respectively. It is difficult to measure
directly using any single aerosol instrument.
Transmission Electron Microscopy (TEM) is an ex situ method for direct characterization of soot morphology (Medalia and Heckman Citation1969; Samson et al. Citation1987; Brasil et al. Citation1999; Gaddam and Vander Wal Citation2013; Kholghy et al. Citation2013; Dastanpour and Rogak Citation2014; Johnson et al. Citation2015; Thajudeen et al. Citation2015). Statistically reliable primary particle sizes can only be acquired by time-consuming analysis of a great number of TEM images.
In an another approach, Eggersdorfer et al. (Citation2012a,Citationb) proposed an indirect method for the measurement of the primary particle diameter using particle mobility, particle mass and two constants, projected area exponent and prefactor
, estimated from either numerical simulations (Medalia Citation1967; Meakin et al. Citation1989; Pierce et al. Citation2006; Al Zaitone et al. Citation2009; Eggersdorfer et al. Citation2012a) or experiments (Megaridis and Dobbins Citation1990; Köylü and Faeth Citation1992; Köylü et al. Citation1995). Average primary particle diameters of flame-made zirconia particles estimated by this model have been shown to be in good agreement with those obtained from standard TEM analysis. However, the model was validated for aggregates with quite uniform primary particle sizes. Additionally, the model was developed for cluster–cluster aggregates formed by coagulation and is also shown to work for sintered structures. However, carbon soot does not sinter; and mainly grows by cluster–cluster collisions and surface growth. The validity of this method is investigated here for soot particles emitted from two reciprocating engines. Here, it is shown that using previously published values of
and
(Eggersdorfer et al. Citation2012a,Citationb), soot primary particle diameters estimated from mass and mobility measurements are inconsistent with those obtained directly from the analysis of TEM images.
In most engineered combustion systems, soot particles are typically formed in turbulent conditions with large variation in local stoichiometry (Mühlbauer et al. Citation2013) and time spent in conditions affecting soot formation, growth, and oxidation (Park and Rogak Citation2003; Park et al. Citation2005). Dastanpour and Rogak Citation(2014) have recently shown that the average diameter of the primary particles changes with the aggregate size in many combustion sources. They have also shown that the primary particles are more uniform in individual aggregates compared to ensembles of aggregates. These findings are consistent with the aggregates being formed in relatively homogenous microscopic regions; after formation and growth, aggregates formed in different regions, with different residence time and/or formation and growth patterns, are mixed. Although this explanation is plausible (indeed, there is no doubt that there are substantial spatial variations in soot formation conditions in combustion devices) (Mühlbauer et al. Citation2013), it is exceedingly difficult to model the impact of these variations on soot structure. In fact, quantitative predictions of soot mass and mean particle size remain very challenging (Boiarciuc, Foucher, and Mounaïm-Rousselle Citation2006).
Considering this size variation, it is shown in the online supplementary information (SI) of this article that using the model constants and prefactor
determined by Eggersdorfer et al. Citation(2012a) results in inaccurate estimation of the primary particle size for carbon soot. Here, it is shown for the first time that their power-law correlation is also true for clusters grown by surface growth (instead of sintering) if correct values of parameters
and
are used. These parameters are determined for a broad range of experimental conditions of two reciprocating engines. Although values of
and
are found to be sensitive to the operating conditions, soot primary particle diameter can still be estimated with reasonable accuracy by mass–mobility measurements using new constant values for
and
.
Experimental
Two types of reciprocating engines were considered for this study. The first was a high pressure direct injection (HPDI) natural gas compression-ignition engine operating with Westport Innovations HPDI natural gas combustion system. Natural gas ignition is provided by a diesel pilot. The engine was operated on a single cylinder at seven different operating conditions according to the European Stationary Cycle (ESC-13; EU Directive 1999/96/EC). Most test points were operated at 15% exhaust gas recirculation (EGR) while the lower-load test points were operated at 20% EGR. The engine was run at 25%, 37%, 50%, and 75% of maximum load at an engine speed of 1500 RPM (denoted as B25, B37, B50, and B75, respectively). The highest load (B75) test point was run at 0%, 20%, and 25% exhaust gas recirculation. The HPDI engine was also run at 63% maximum load and a lower speed of 1200 RPM at 0% EGR (denoted as A63). At this operating condition, the majority (˜80%) of the natural gas was injected into the combustion chamber during the intake stroke, allowing the bulk of the gas to premix before ignition. The second engine used Gasoline Direct Injection (GDI) and was operated at simulated highway cruise condition (2600 RPM and 58 N.m of torque), and a higher speed and lower torque setting (3000 RPM and 38 N.m), denoted by Highway and Speed thereafter, respectively. The GDI engine was fueled by mixtures of gasoline and 0% (E0), 10% (E10), and 30% (E30) ethanol by volume.
All measurements were carried out downstream of a thermodenuder operating at approximately 200°C (Patychuk Citation2013). For HPDI tests, higher TD temperatures were also tested and no significant change was observed in particle number and size distributions. Although this is not a precise way to determine the mass of organics on the soot, but a change in the size distribution (Graves et al. Citation2015) will be observed for semi-volatile mass fractions of 10% or more. Even if 10% of the particle mass was composed of volatile material, primary particle diameter would have approximately decreased by only 5% under the vacuum condition of the TEM (considering densities of the particle core and coating to be 1800 and 1200
, respectively).
A TSI 379020A two-stage rotary disk diluter was also used upstream of the TD in GDI campaign (Dastanpour and Rogak Citation2014). The first and second stages of the diluter were heated to 80°C and 300°C, respectively. The thermodenuder and heated dilution removed volatile components from the particulate sample, and what remained were non-volatile particles, predominantly composed of elemental carbon. More information on the effects of denuding and heated dilution on particle volatility is presented in the SI (Section S1).
Soot samples were collected on TEM grids using a thermophoretic precipitator and images were produced by a Hitachi H7600 transmission electron microscope operating at 80 kV under high-resolution mode. Soot particles were size classified using a TSI differential mobility analyser (Model 3081) and their masses were measured by a cambustion centrifugal particle mass analyser (CPMA) (Olfert and Collings Citation2005).
Details of the experimental methods are further described in Dastanpour and Rogak Citation(2014) and Graves et al. Citation(2015).
Theory
As discussed by Eggersdorfer et al. Citation(2012a), the average projected area () of fractal-like aggregates can be related to the number of their constituent primary particles
by the following power-law (Medalia Citation1967) correlation:
[1] where
is the projected area of a primary particle with surface area mean (Sauter) diameter of
, and
and
are the prefactor and projected area exponent that depend on aggregate morphology and collision type. For aggregates of monodisperse point-touching primary particles,
and
are the actual number and diameter of the monomers in the cluster, respectively. However, real soot aggregates are composed of polydisperse primary particles and are partially coalesced. Surface-area equivalent diameter (
) and number (
) of the primary particles in polydisperse aggregates are defined as following (Kruis et al. Citation1993):
[2]
[3] where
and
are volume and surface area of the aggregate, respectively, and
and
are usually extracted from fitting Equation (1) to the results obtained from numerical simulations (Medalia Citation1967; Meakin et al. Citation1989; Köylü et al. Citation1995; Pierce et al. Citation2006; Al Zaitone et al. Citation2009; Eggersdorfer et al. Citation2012b) or experimental measurements (Köylü and Faeth Citation1992; Köylü et al. Citation1995). Values reported in literature for
and
are in the range of 1.0–1.18 and 1.07–1.15, respectively (summary of the reported values is reported in ). These values were obtained for cases where average
is statistically independent of the aggregate size.
Table 1. and
values reported in literature.
The number of the primary particles in individual aggregates can also be correlated to the mass of the aggregate, m, by,[4] where
, and
is the material density. Different values in the range of 1770–2100 kg/m3 are reported for soot density (Dobbins at al. Citation1994; Park et al. Citation2004a, Citation2004b); here
= 1800 kg/m3 is used.
The mobility diameter () of aggregates in the free molecular (Meakin et al. Citation1989) and transition (Rogak et al. Citation1993) regimes is approximately equal to the projected area equivalent diameter (
) of the aggregates (
) (Eggersdorfer et al. Citation2012a). Park et al. Citation(2004a,Citationb) showed that this approximation is accurate for diesel aggregates of up to
nm. Considering this approximation, and by combining Equations (1) and (2),
can be written in terms of
and
(Eggersdorfer et al. Citation2012a):
[5]
Substituting Equation (4) into Equation (1) and taking the natural logarithm, Equation (6) can be derived. This equation can be used for the estimation of and
from combined mass, mobility, and electron microscopy measurements.
[6]
As shown by Dastanpour and Rogak Citation(2014), primary particle diameter scales with aggregate size in many soot sources, including those considered here. Based on examination of individual aggregates, there is substantial variation about the mean trend, so it is difficult to determine an exact functional relation between the primary particle and aggregate diameters. A power-law relation similar to Equation (7) is consistent with the measurements (see Figures S2 and S3 and Tables S1 and S2 in the online SI), and is mathematically convenient.[7]
Sauter diameters of the primary particles estimated by power-law correlations are used for mass and mobility diameter normalizations in Equation (6).
Results and discussion
Model accuracy using Dα =1.07 and ka = 1.0
Sample TEM images produced in this study are shown in . Particles were stable under the electron beam for all cases considered here. Images shown here are samples of the images taken for each operating condition and their characteristic dimensions do not necessarily represent the averaged parameters obtained from the analysis of tens to hundreds of images produced for each test point.
Figure 1. Sample TEM images taken from different operating conditions of HPDI and GDI engines. Images are selected randomly. Illustrated images have the same magnification. Scale bar is 100 nm.
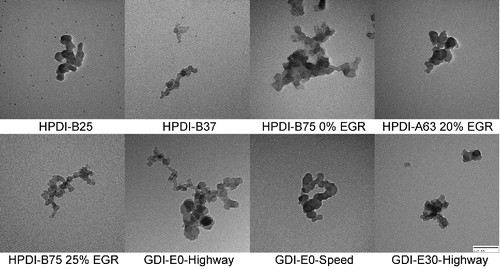
Average Sauter diameters of the primary particles were estimated at different mass–mobility test points using the prefactor and projected area exponent parameters obtained by Eggersdorfer et al. Citation(2012b) ( and
) and Equation (5). These diameters are compared with those directly obtained from the analysis of the TEM images as shown in Figures S2 and S3 in the online SI. On average, primary particle diameters determined by this algorithm were 41% and 21% different from those obtained from the analysis of TEM images produced for HPDI and GDI engines, respectively. TEM analysis provides scattered and discrete information on the primary particle and aggregate sizes. Consequently, TEM-based Sauter diameters of the primary particles corresponding to each mobility diameter,
, were estimated using Equation (7) fitted to TEM results obtained at different operating conditions. Details of this procedure and values obtained for
and
are discussed in online SI (Section S2).
As shown in Figures S2 and S3, although this model predicts an increase in primary particle size with aggregate size, qualitatively consistent with TEM measurements (Dastanpour and Rogak Citation2014), it does not estimate primary particle size accurately, especially for large aggregates.
Estimation of Dα and ka for soot particles using experimental measurements and optimization
The accuracy of primary particle size measurement from mass–mobility measurements is sensitive to model parameters and
. Here, two approaches are used for the calculation of these parameters at different operating conditions of the engines considered in this study.
First, TEM-determined Sauter diameters of the primary particles were estimated for all mass–mobility measurement test points with the application of Equation (7) on TEM image processing data. Optimum values of and
(referred to as
and
thereafter) were calculated using regression to minimize the difference between TEM-determined
and the results of Equation (5). Using this method, the average primary particle sizing error was reduced to approximately 11% and 5% for HPDI and GDI soot samples, respectively. These primary particle sizing errors are the minimums that could be achieved from mass–mobility measurements; these include measurement uncertainties of instruments, TEM image processing uncertainties, and regression accuracy.
Second, mass–mobility data (obtained from CPMA and differential mobility analyser measurements) and TEM-obtained (and
) were used in Equation (6); and model parameters
and
were determined at each operating condition using regression.
Model constants derived from both approaches are reported in ; prefactor and projected area exponent change considerably (up to 22% for and 82% for
) from one test point to another. Additionally, means of these parameters are different for the combustion sources considered here. Consequently, accurate estimation of the primary particle diameter requires this model to be calibrated for each operating condition or at least soot source. Furthermore, both approaches used for the estimation of
and
(“optimized” and “measured”) from combined mass–mobility and TEM measurements result in approximately similar parameter values.
Table 2. Prefactor and projected area exponent measured with different methods.
It should be mentioned that the variation of the primary particle diameter with the mobility diameter of the aggregate has to be considered when Equation (6) is used. Assuming average to be constant and independent of the mobility diameter (set to the arithmetic mean TEM-based Sauter primary particle diameter), normalized particle mass (
) can be measured from Equation (6) using
and
parameters suggested by Eggersdorfer et al. Citation(2012a,Citationb). As shown in , negative values are measured for logarithms of normalized mass and mobility diameters for many test points in this case. This means that the aggregates had mass and/or mobility diameters smaller than a single primary particle, which is physically impossible. The assumption of a constant average
for the whole size distribution of the aggregates is erroneous for systems having a considerable variation in average primary particle diameter with aggregate size.
Figure 2. Logarithmic plot of normalized aggregate mass in relation to the normalized mobility-equivalent diameter when average is assumed to be constant and independent of the aggregate size. Left panel: HPDI engine. Right panel: GDI engine.
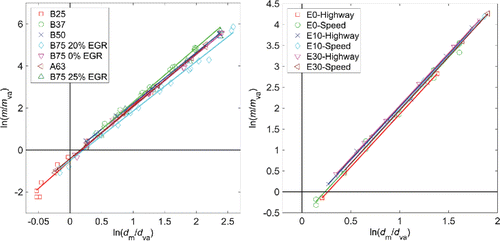
Considering correlation between primary particle and aggregate sizes as described by Equation (7) and discussed in Section S2 of the online SI, was measured for each mobility diameter. Results obtained for the normalized mass and mobility of soot aggregates with this adjustment are shown in . As illustrated, logarithms of normalized mass and mobility diameter are no longer negative for most points (except for two which could be ascribed by the measurement uncertainties).
Modified model parameters
Average (
) and
(
) measured for the HPDI engine were used for the calculation of the primary particle sizes in both engines. Using
and
instead of
and
(Eggersdorfer et al. Citation2012b), the average error in the estimation of the primary particle size was reduced from 41% and 21% to 19% and 12% for different operating conditions of HPDI and GDI engines, respectively. The accuracy of the model using these “modified parameters” is compared to cases where optimized parameters (minimum error) and parameters governed by Eggersdorfer et al. Citation(2012b) were used; results are reported for individual operating conditions in .
Figure 4. Average Δdva measured for different sets of and
at multiple operating conditions. Eggersdorfer et al. Citation(2012b) model uses
= 1.0 and
= 1.07;
and
parameters are determined for each operating condition separately. Modified model uses
= 1.13 and
= 1.1.
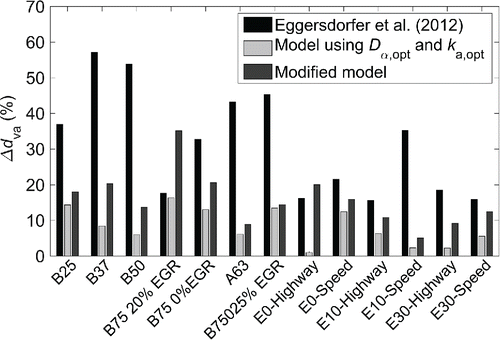
Primary particle diameter estimated from Equation (3) (for the original and modified values of and
) for a particular mobility diameter is plotted against the TEM-obtained diameter,
, for the same particle projected area equivalent diameter (i.e., assuming
) in .
is measured from the application of Equation (6), where
and
are determined from regressions to the whole TEM analysis data (described in the online SI, Section S3). As shown, using newly proposed values of
and
, the difference between estimated Sauter diameters and those obtained from TEM analysis, especially at large mobility diameters, was reduced at all operating conditions of both soot sources. At each operating condition, primary particle size has a considerable wide size distribution that is associated with variations in the aggregate size. Note that in all cases, the original parameters (
and
) resulted in the primary particle diameter increasing faster with
than indicated by TEM. As shown in this , for some operating conditions such as B75 20% EGR, and B75 0% EGR, neither the old nor the new models agree well with TEM measurements. After re-examining these measurements, it appears that they are similar to other points except for especially low values of
—that is, primary particle diameter has a weak correlation with aggregate size. However, a clear trend was not observed between the accuracy of the model and
.
Figure 5. Sauter primary particle diameter measured from Equation (5) using parameters suggested by Eggersdorfer et al. Citation(2012b) and those obtained in this study versus TEM data for both HPDI and GDI engines.
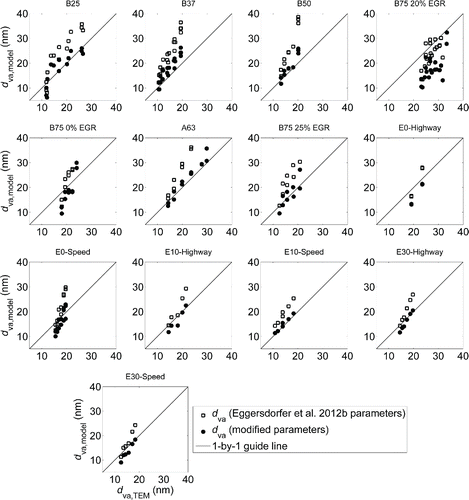
Conclusions
Models developed for in situ estimation of Sauter diameters of the primary particles with mass–mobility measurements were compared with TEM measurements. We have found that good agreement between TEM and in situ estimations can be obtained using model parameters that depend on source and operating conditions. Variation of the primary particle diameter with aggregate size is also taken into account in the estimation of the new model parameters. Consideration of this size correlation is essential in order to obtain physically realistic mass and mobility. We have also found that for nearly all cases, agreement can be improved with the application of new, constant values for the model parameters and
, but it remains to be seen whether these values will work for a broad range of soot sources.
UAST_1130796_Supplementary_file.zip
Download Zip (1.6 MB)Acknowledgments
The authors would like to thank James Wallace, Phillip Mireault, and Manuel Ramos (University of Toronto), and Bronson Patychuk (Westport Innovations, Inc.) for their contributions to the sampling campaigns for GDI and HPDI engines, respectively.
References
- Al Zaitone, B., Schmid, H., and Peukert, W. (2009). Simulation of Structure and Mobility of Aggregates Formed by Simultaneous Coagulation, Sintering and Surface Growth. J. Aerosol Sci., 40(11):950–964.
- Boiarciuc, A., Foucher, F., and Mounaïm-Rousselle, C. (2006). Soot Volume Fractions and Primary Particle Size Estimate by Means of the Simultaneous Two-Color-Time-Resolved and 2D Laser-Induced Incandescence. Appl. Phys. B, 83(3):413–421.
- Brasil, A. M., Farias, T. L., and Carvalho, M. G. (1999). A Recipe for Image Characterization of Fractal-Like Aggregates. J. Aerosol Sci., 30(10):1379–1389.
- Dastanpour, R., and Rogak, S. N. (2014). Observations of a Correlation between Primary Particle and Aggregate Size for Soot Particles. Aerosol Sci. Technol., 48(10):1043–1049.
- Dobbins, R. A., Mulholland, G. W., and Bryner, N. P. (1994). Comparison of a Fractal Smoke Optics Model with Light Extinction Measurements. Atmos. Environ., 28(5):889–897.
- Eggersdorfer, M. L., Gröhn, A. J., Sorensen, C. M., McMurry, P. H., and Pratsinis, S. E. (2012a). Mass-Mobility Characterization of Flame-Made ZrO2 Aerosols: Primary Particle Diameter and Extent of Aggregation. J. Colloid Interface Sci., 387(1):12–23.
- Eggersdorfer, M. L., Kadau, D., Herrmann, H. J., and Pratsinis, S. E. (2012b). Aggregate Morphology Evolution by Sintering: Number & Diameter of Primary Particles. J. Aerosol Sci., 46:7–19.
- Franchini, M., and Mannucci, P. M. (2012). Air Pollution and Cardiovascular Disease. Thromb. Res., 129(3):230–234.
- Gaddam, C. K., and Vander Wal, R. L. (2013). Physical and Chemical Characterization of SIDI Engine Particulates. Combust. Flame., 160(11):2517–2528.
- Graves, B., Olfert, J., Patychuk, B., Dastanpour, R., and Rogak, S. N. (2015). Characterization of Particulate Matter Morphology and Volatility from a Compression-Ignition Natural Gas Direct-Injection Engine. Aerosol Sci. Technol., 49(8):589–598.
- Jacobson, M. Z. (2010). Short-Term Effects of Controlling Fossil-Fuel Soot, Biofuel Soot and Gases, and Methane on Climate, Arctic Ice, and Air Pollution Health. J. Geophys. Res., 115:D14209.
- Johnson, T. J., Olfert, J. S., Symonds, J. P. R., Johnson, M., Rindlisbacher, T., Swanson, J. J., Boies, A. M., Thomson, K. , Smallwood, G., Walters, D., Sevcenco, Y., Crayford, A., Dastanpour, R., Rogak, S. N., Durdina, L. , Bahk, Y. K., Brem, B., and Wang, J. (2015). Effective Density and Mass-Mobility Exponent of Aircraft Turbine Particulate Matter. J. Propul. Power, 31(2):573–582.
- Kholghy, M., Saffaripour, M., Yip, C., and Thomson, M. J. (2013). The Evolution of Soot Morphology in a Laminar Coflow Diffusion Flame of a Surrogate for Jet A-1. Combust. Flame, 160(10):2119–2130.
- Köylü, Ü. Ö., and Faeth, G. M. (1992). Structure of Overfire Soot in Buoyant Turbulent Diffusion Flames at Long Residence Times. Combust. Flame, 89(2):140–156.
- Köylü, Ü. Ö., Faeth, G. M., Farias, T. L., and Carvalho, M. G. (1995). Fractal and Projected Structure Properties of Soot Aggregates. Combust. Flame, 100(4):621–633.
- Kruis, F. E., Kusters, K. A., Pratsinis, S. E., and Scarlett, B. (1993). A Simple Model for the Evolution of the Characteristics of Aggregate Particles Undergoing Coagulation and Sintering. Aerosol Sci. Technol., 19(4):514–526.
- Maynard, A. D., and Kuempel, E. D. (2005). Airborne Nanostructured Particles and Occupational Health. J. Nanopart. Res., 7(6):587–614.
- Meakin, P., Donn, B., and Mulholland, G. W. (1989). Collisions between Point Masses and Fractal Aggregates. Langmuir, 5(2):510–518.
- Medalia, A. I. (1967). Morphology of Aggregates. J. Colloid Interface Sci., 24(3):393–404.
- Medalia, A. I., Heckman, F. A. (1969). Morphology of Aggregates—II. Size and Shape Factors of Carbon Black Aggregates from Electron Microscopy. Carbon, 7(5):567–582.
- Megaridis, C. M., and Dobbins, R. A. (1990). Morphological Description of Flame-Generated Materials. Combust. Sci. Technol., 71(1–3):95–109.
- Mühlbauer, W., Leidenberger, U., Lorenz, S., and Brüggemann, D. (2013). Optical Studies About the Influence of Diesel Engine Operating Parameters on the Physicochemical Properties of Emitted Soot Particles. SAE Int. J. Engines 6(3):1866-1876.
- Olfert, J. S., and Collings, N. (2005). New Method for Particle Mass Classification—the Couette Centrifugal Particle Mass Analyzer. J. Aerosol Sci., 36(11):1338–1352.
- Park, K., Kittelson, D. B., and McMurry, P. H. (2004a). Structural Properties of Diesel Exhaust Particles Measured by Transmission Electron Microscopy (TEM): Relationships to Particle Mass and Mobility. Aerosol Sci. Technol., 38(9):881–889.
- Park, K., Kittelson, D. B., Zachariah, M. R., and McMurry, P. H. (2004b). Measurement of Inherent Material Density of Nanoparticle Agglomerates. J. Nanopart. Res., 6(2/3):267–272.
- Park, S. H., and Rogak, S. N. (2003). A One-Dimensional Model for Coagulation, Sintering, and Surface Growth of Aerosol Agglomerates. Aerosol Sci. Technol. 37(12):947–960.
- Park, S. H., Rogak, S. N., Bushe, W. K., Wen, J. Z., and Thomson, M. J. (2005). An Aerosol Model to Predict Size and Structure of Soot Particles. Combust. Theor. Model. 9(3):499–513.
- Patychuk, B. D. (2013). Particulate Matter Emission Characterization from a Natural Gas High-Pressure Direct-Injection Engine. Master's thesis, University of British Columbia, Vancouver, Canada.
- Pierce, F., Sorensen, C. M., and Chakrabarti, A. (2006). Computer Simulation of Diffusion-Limited Cluster-Cluster Aggregation with an Epstein Drag Force. Phys. Rev. E, 74(2):021411.
- Rogak, S. N., Flagan, R. C., and Nguyen, H. V. (1993). The Mobility and Structure of Aerosol Agglomerates. Aerosol Sci. Technol., 18(1):25–47.
- Samson, R. J., Mulholland, G. W., and Gentry, J. W. (1987). Structural Analysis of Soot Agglomerates. Langmuir, 3(2):272–281.
- Sorensen, C. M. (2001). Light Scattering by Fractal Aggregates: A Review. Aerosol Sci. Technol., 35(2):648–687.
- Thajudeen, T., Jeon, S., and Hogan, C. J. (2015). The Mobilities of Flame Synthesized Aggregates/Agglomerates in the Transition Regime. J. Aerosol Sci., 80:45–57.