ABSTRACT
Wind tunnel experiments examined the coupled effects of relative humidity (RH) and surface and particle properties on aerodynamically induced resuspension. Hydrophilic glass spheres and hydrophobic polyethylene spheres ∼20 μm in diameter, with nanoscale surface features, were resuspended from hydrophilic glass, hydrophobic chemical agent resistant coating (CARC), and gold surfaces. Roughness of the glass and gold surfaces was on the nanoscale, whereas CARC surfaces had microscale roughness. Different particle–surface combinations yielded van der Waals interactions that varied by a factor of 4, but these differences had a relatively minor effect on resuspension. Wind tunnel RH was varied between 7% and 78%. Overall, RH affected the resuspension of hydrophilic particles on hydrophilic surfaces most strongly and that of hydrophobic particles on hydrophobic surfaces the least. For each particle–surface combination there was a threshold RH value below which resuspension rates were essentially constant and in good agreement with a dimensionless model of particle resuspension.
Copyright © 2016 American Association for Aerosol Research
EDITOR:
1. Introduction
Particles present on a surface can be introduced into an air stream via resuspension. Resuspension is implicated in the lingering of airborne radioactive particles at nuclear weapon test sites or near nuclear power plants (Nicholson Citation1988) after an accidental release, as well as prolonged exposure to pesticides after spraying (Orgill et al. Citation1976). Human activities, such as walking and cleaning, induce resuspension of particulate matter and affect indoor air quality (Gomes et al. Citation2007; Corsi et al. Citation2008; Qian and Ferro Citation2008; Oberoi et al. Citation2010). During the anthrax attack in the Hart Senate Office building in 2001, the presence of airborne Bacillus anthracis spores 25 days after the initial release was attributed to resuspension of the spores from contaminated surfaces (Weis et al. Citation2002). Finally, resuspension of virus-infected, exfoliated skin cells is hypothesized to be a route for livestock infection (Dillon Citation2011). Thus, understanding and predicting the rate at which particles undergo resuspension is critical in a broad range of indoor and outdoor respiratory risk assessments.
Whether a particle will resuspend or not is determined by the sum of the adhesion and removal forces that act on it. The major adhesion forces include van der Waals, capillary, and electrostatic forces. The removal forces can be aerodynamic, vibrational, or mechanical. Understanding and predicting resuspension is complicated by the fact that both the interactions between the particle and a surface and those between the particle and the surrounding fluid influence these forces (Ziskind Citation2006).
For resuspension caused by fluid motion, early studies (Corn Citation1961; Corn and Stein Citation1965; Nicholson Citation1988; Nicholson Citation1993) demonstrated that increasing time, particle size, and velocity, in particular the friction velocity u* = (τw/ρ)1/2, increases particle resuspension as does increasing particle asperity (Goldasteh et al. Citation2012), turbulence intensity (Mukai et al. Citation2009), or turbulent kinetic energy (Lengweiler Citation2000). Flow acceleration was not found to influence resuspension over the range that was tested and within the experimental uncertainty limit (Ibrahim et al. Citation2004). Increasing surface roughness generally reduces resuspension (Loosmore Citation2003; Kassab et al. Citation2013). A higher particle surface density also increases resuspension, because particles moving along the surface after detachment collide with stationary particles on the surface inducing additional detachment (Ibrahim et al. Citation2004). Furthermore, multilayer deposits show significantly higher resuspension than single-layer deposits (Boor et al. Citation2011).
Relative humidity (RH) often affects adhesion forces (Corn and Stein Citation1965) in a nonlinear manner. In particular, high RH can lead to capillary condensation between a particle and a surface strongly enhancing adhesion and suppressing particle resuspension (Ibrahim et al. Citation2004; Hubbard et al. Citation2012). Ibrahim et al. Citation(2004) showed that the threshold velocity to detach 50% of the 70 μm stainless steel spheres on glass surfaces in a wind tunnel was increased from 3.6 m/s to 13.4 m/s as humidity increased from 18% to 67%. Likewise, resuspension of 1.7 – 14.4 μm poly(methyl methacrylate) (PMMA) spheres from TiO2 surfaces by mechanical impulse force (vibration) decreased monotonically with increasing RH (Hubbard et al. Citation2012).
In the past decade, adhesion force studies using atomic force microscope (AFM) have yielded a more complex picture of capillary forces (Bocquet et al. Citation1998; Halsey and Levine Citation1998; Ata et al. Citation2002; Jones et al. Citation2002; Rabinovich et al. Citation2002; Farshchi-Tabrizi et al. Citation2006; Paajanen et al. Citation2006; Farshchi-Tabrizi et al. Citation2008; Butt and Kappl Citation2009; Szori et al. Citation2010). These studies show that in addition to RH, capillary force strength is influenced by surface chemistry and geometry (Jones et al. Citation2002), especially the nanoscale roughness of both the particles and the surfaces (Rabinovich et al. Citation2002; Hubbard et al. Citation2012). These roughness studies are important, because most particles found in the environment are not smooth at the nanoscale (Goldasteh et al. Citation2012). Overall, studies to date speak to the complexity of the physics controlling resuspension and the many parameters that affect it.
The goal of our work is to investigate the effects of RH, as well as surface and particle properties, on particle resuspension by aerodynamic forces and to use these data to improve a semi-empirical model for particle resuspension. We measure the resuspension rates of hydrophilic glass and hydrophobic polyethylene (PE) particles on three different surfaces in a newly developed wind tunnel at RH levels as high as 80%. The ∼20 μm glass and PE particles have nanoscale bumps on the surfaces and, thus, resemble environmental particles. Furthermore, bacterial spore agglomerates are in the 5–10 μm range and fungal spores are 1–30 μm (Jones and Harrison Citation2004) and have densities comparable to the PE. Glass particles, in contrast, are more characteristic of mineral matter. Hydrophilic glass microscope slides exhibit roughness on the nanoscale, whereas the hydrophobic chemical agent resistant coating (CARC) on aluminum coupons have microscale roughness. Gold coated glass slides are only slightly less hydrophobic than CARC, but have surface roughness similar to the glass slides. Furthermore, the conductivity of the gold surfaces minimizes the electrostatic force contribution to the adhesion forces. Overall, different particle –surface combinations change the magnitude of the van der Waals interactions by about a factor of 4.
2. Materials and methods
2.1. Particles and surfaces
The 18 – 22 µm glass microspheres (S-SLGMS 18–22 µm, Cospheric, Santa Barbara, CA, USA) have a density of 2.5 g/cm3. The ∼20 µm PE microspheres (Equistar Microethene FN 519–00, Lyondell Basell Industries, Houston, TX) have a density of 0.923g/cm3. Microsphere surface roughness was characterized using an AFM (Dimension 3100, Bruker Nano, Santa Barbara, CA, USA) scanning a 6 μm × 6 μm area in tapping mode at a scan rate of ∼1 Hz. The TESP tips (Bruker Nano Probes, Camarillo, CA, USA) had a Mode 1 resonant frequency of ∼300 kHz and force constant approximately 40 N/m. The height images were second-order-flattened in the x-y direction.
Glass slides (75 mm × 25 mm, soda-lime glass, Catalog No. 2947–75×25, Corning Inc., Corning, NY), were cleaned with ethanol (200 proof, Pharmco, Brookfield, CT, USA) and wiped with a clean room wipe cloth (TX606, ITW Texwipe, Kernersville, NC, USA) at least 30 min before particle deposition. CARC surfaces were prepared by applying a chromate conversion coating (MIL-C-5541 Type 1 Class 1A) to bare aluminum coupons (2024T3, 75 mm × 25 mm × 0.8 mm thick), followed by a primer (MIL-DTL-53022) and CARC paint (MIL-DTL-53039 Type 3 polymeric 686A, Tan 33446, Sherwin Williams). The coating purity of the gold-coated slides (CA134, EMF Corp., Ithaca, NY, USA) was 99.9%. CARC and gold surfaces were not additionally cleaned.
Surface roughness was measured using a profilometer (XP-1High Resolution Surface Profiler, AmBios Technology, Inc., Santa Cruz, CA, USA) scanning at 0.01 mm/sec with a stylus force of 0.05 mg. Ten different sample surfaces were scanned for 1.5 mm (glass and gold) or 3.0 mm (CARC) and averaged to yield representative surface roughness. The contact angle with water was measured using a sessile drop method (FTA-1000B, First Ten Angstroms, Portsmouth, VA, USA) with 5 μL water droplets for three samples and averaged.
2.2. Wind tunnel
All experiments were conducted in the wind tunnel illustrated in . Design details and tunnel characterization results are available in the online supplemental information (SI). To summarize briefly, the tunnel has three 1 m working sections (0.25 m × 0.25 m cross-sectional area), and sand paper at the entrance to section 1 triggers the transition from laminar to turbulent boundary layers. A centrifugal blower (10 HP) with a variable frequency drive controls the air speed. When the blower is started, the freestream velocity increases almost linearly to its steady state value of 7.6 m/s, 10.1 m/s and 12.7 m/s in 11.2 s, 14.5 s, and 18.4 s, respectively.
Figure 1. A schematic diagram of the wind tunnel. Here, x = 0 denotes the beginning of the working section 1, y = 0 is the horizontal centerline, and z = 0 is the floor of the working section. More extensive documentation regarding the design is available in the SI.
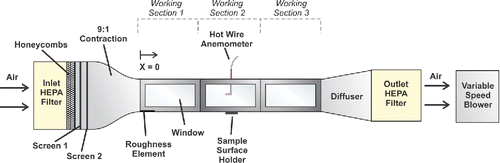
Free stream velocities and vertical velocity profiles were measured using constant temperature anemometry (MiniCTA, Dantec Dynamics, Ramsey, NJ, USA). The friction velocity was characterized using the Preston tube method (Bechert Citation1996).
2.3. Particle dispersion, characterization, and resuspension experiments
Microspheres were dispersed onto the test surfaces using the sample dispersion unit (SDU) available with the Morphologi G3S (Malvern Instruments, Westborough, MA, USA) particle size and shape image analyzer. For all microsphere experiments, ∼1 mm3 of powder was placed in the sample cartridge, and the open end of the SDU chamber was pressed against a glass plate (180 mm × 110 mm) with a sample surface (75 mm × 25 mm) placed on the top of the glass plate. Nitrogen, at 200 kPa, was introduced for 20 ms through the gas inlet located just above the sample cartridge to puncture the foil and disperse the powder. After 120 s, the chamber was raised to retrieve the particle laden sample surface. The Morphologi G3S instrument was used to characterize the particles with respect to size, morphology, and number. The magnification, lighting settings, and characterization area used for each surface-particle combination are summarized in Table S1 in the SI.
After characterizing the deposited particles, the sample surface was inserted into the wind tunnel through the bottom so that the sample surface was located at x = 1.5 m, and y = z = 0 m (see ). For a resuspension experiment, the blower was turned on for a predetermined period of time and the temperature and RH were monitored throughout. Typically, ∼20 min elapsed between starting particle deposition and starting the wind tunnel. The time period was as short as possible to avoid aging or residence time issues, since long residence times suppress resuspension especially at high humidity (Ibrahim et al. Citation2004). After the sample was exposed for 2, 5, or 10 minutes in the wind tunnel, it was retrieved and the same scan area was recharacterized using the Morphologi G3S. The experimental particle resuspension rate was calculated as[1]
The resuspension rate is reported along with the corresponding mid-point time tm = (te + t0)/2, (Kim et al., Citation2010).
3. Results and discussions
3.1. Wind tunnel characteristics
Resuspension experiments are usually characterized using the freestream velocity U∞ or the friction velocity u*. Since u* is related to the wall shear stress, this velocity is more relevant to resuspension and makes it possible to compare results from experiments with different flow configurations (Loosmore Citation2003; Ibrahim Citation2004). In our wind tunnel, U∞ varied between 2.7 m/s and 12.7 m/s, there was a linear relationship between u* and U∞ (u* = 0.0375U∞ + 0.0213; Figure S1), and the turbulence intensity of the freestream was always less than 1.1%. The velocity profile (Figure S2) was uniform in the central region of the duct, and decreased rapidly on approaching the wall. The turbulence intensity increased as measurements approached the floor (z = 0), reaching 13% at z = 0.003 m. Finally, the profile near the wall was in good agreement with the law of the wall.
3.2. Surface properties
The measured surface properties are summarized in . Glass and gold surfaces are smooth and exhibit only nanometer scale roughness. CARC, in contrast, has surface roughness that is three orders of magnitude higher. Based on the contact angle measurements, the glass surface is hydrophilic, while the CARC and gold surfaces are both hydrophobic. Although clean gold is hydrophilic, with a water contact angle <10°, gold surfaces readily become hydrophobic on exposure to water droplets or atmospheric air due to contamination by a partial monolayer of carbonaceous materials (Smith Citation1980). Water contact angles for gold surfaces are typically in the range of 30° to 80°, and, thus, the measured contact angle is reasonable for our air-exposed gold surface.
Table 1. The surface property values are reported as the average ± standard deviation based on ten samples for surface roughness and three samples for contact angle.
3.3. Particle roughness
Particles found in the environment are extremely diverse with respect to shape and roughness. Dust particles are highly irregular and rough (Goldasteh et al. Citation2012) whereas individual soot particles are spherical but aggregate to form fractal structures (Sander et al. Citation2010). Surface roughness measurements of environmental particles are rare, but most particle surfaces are not smooth. Dry B. atrophaeus spores have nanoscale ridges on the surface (Plomp et al. Citation2005), and clusters of spores have surface roughness similar to, or smaller than, the dimensions of a single spore (0.5 μm 1 μm, Aptowicz and Chang Citation2005). Although choosing truly representative particles is difficult, rough particles are more likely to mimic the behavior of environmental particles than highly smooth particles.
Scanning electron microscopy (Figure S3) showed that the surfaces of the glass and PE particles are not smooth. Edge views of the particles suggest that the roughness elements are almost always less than 100 nm. Scanning AFM experiments characterized the roughness of the particles more carefully (Figure S4) and found that the average surface roughness was 27.5 nm and 20.9 nm for the glass and PE spheres, respectively. The root-mean-square (RMS) surface roughness was 40.4 nm and 27.4 nm for glass and PE spheres, respectively.
3.4. Measuring resuspension rates
The resuspension rate (Λexperiment) is defined as the fraction of particles removed from the surface per unit time (Equation Equation(1)[1] ). One way to determine Λexperiment as a function of time is to continuously measure the particle concentration in air samples downstream of the surface (Nicholson Citation1993; Krauter and Biermann Citation2007). The challenges inherent in this approach include ensuring isokinetic sampling and carefully choosing the sampling locations so that the concentration measurements are representative. In our wind tunnel, computational fluid dynamics (CFD) simulations showed that, even at the highest velocity (12.7 m/s), homogenous particle concentrations are not achieved at the exit of the wind tunnel. Rather, there is a strong vertical concentration gradient with 99% of the resuspended particles located in the lower 20% of the wind tunnel. A wind tunnel ∼20 m long is required to ensure that the particles are uniformly dispersed in the flow.
The resuspension flux from a surface can also be determined by monitoring the surface in real time using an in situ imaging system. For example, Wu et al. Citation(1992) used a stereomicroscope and charge-coupled device (CCD) camera to measure the fraction of particles remaining on the surface as a function of time, and Ibrahim et al. Citation(2003) used a similar approach to observe that rolling was the primary mechanism of particle detachment. More recently, particle trajectories after detachment were studied by Kassab et al. Citation(2013) who observed three different types of particle motion: (1) rolling/bouncing, (2) immediate liftoff, and (3) rolling/bouncing for a certain distance before final liftoff. The field of view in these experiments varies depending on the camera and optical setup, and, for a fixed number of pixels, the resolution is correlated to the field of view, i.e., smaller particles require a finer resolution, and thus a more restricted field of view. Typically, the field of view is on the order of 100–150 mm2 (Ibrahim et al. Citation2003; Kassab et al. Citation2013).
Finally, the number of particles on a surface can be examined ex situ by measuring the number of particles on the surface before and after a resuspension experiment (Braaten Citation1994; Reeks and Hall Citation2001), and this is the approach we adopt here. The major disadvantage of this method over those discussed above is that the resuspension rate for a given sample is usually only obtained for a single time period rather than as a function of time. Although a sample surface can be characterized at intermediate times and placed back into the wind tunnel for further resuspension measurements, particle aging effects can complicate interpretation. The key advantage of the ex situ technique over in situ monitoring, however, is that the magnification of the image and the size of the viewing area can be decoupled by scanning many fields of view. Thus, in our experiments the total area scanned ranged from 385 mm2 to 880 mm2. Given the stochastic nature of resuspension events by turbulent bursts, examining a larger field of view should reduce the variability between nominally identical measurements. In this study, we use the motorized x-y-z sample table of the Morphologi G3S particle characterization system to count and characterize particles in more than a hundred fields of view per sample in a short time period (∼10 min for the characterization areas detailed in Table S1) from exactly the same areas before and after a resuspension experiment.
3.4.1. Glass particles
Representative images of glass particles taken at the same locations on the three sample surfaces of interest before and after a resuspension experiment are illustrated in Figure S5. For each experiment the software analyzed 125–252 frame images to obtain particle properties, including the size and aspect ratio, for all of the particles in the saved frame images. Particle analysis, , showed that although most glass particles were in the 18–24 μm size range, particles smaller than 10 μm were also present and some particles were deposited as planar doublets and triplets. Since averaging over all of the “particles,” i.e., singles, doublets, etc., would distort the experimental resuspension rates, particles were first classified based on size and aspect ratio, where the latter was used to differentiate single spheres from agglomerates. Examples of particles deposited on the glass surface, are shown in and S6, and the complete classification scheme for glass particles is summarized in Table S2. The same classification scheme was used for glass particles deposited on gold surfaces. For the CARC surfaces, the aspect ratio criterion was relaxed from 0.9 to 0.7 due to interference by the surface features. In addition, surface features initially identified as particles by the instrument software were manually discarded prior to determining the resuspension rates.
Figure 2. The particle size distribution of the glass spheres on a glass surface before and after a resuspension experiment. The images correspond to Classes 4, 5, and 6 glass particles.
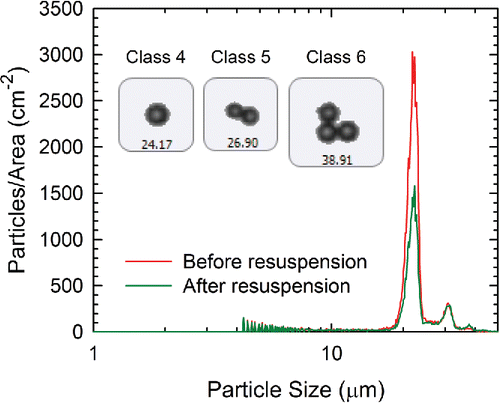
In , the peak at ∼22 μm corresponds primarily to single particles (Class 4), and the peaks at 31 and 38 μm correspond to particles deposited as doublets (Class 5) and triplets (Class 6), respectively. After the resuspension experiment, the number of particles near the ∼22 μm peak decreased significantly, whereas the numbers of agglomerates did not change. Normally, larger particles resuspend more readily than smaller particles because their height ensures that they experience higher velocities in the viscous sublayer of the boundary layer (Ibrahim et al. Citation2003; Soltani and Ahmadi Citation1994; Ziskind Citation2006). In the current study, however, larger particles are planar agglomerates that appear to be touching the surface as well as each other. As a result, the height of these composite particles remains the same, the area of contact between the particles and the surface increases, and their ability to resuspend is greatly reduced. Particles smaller than 16 μm (Classes 1–3) also did not resuspend under the conditions tested. Thus, only Class 4 glass particles were used to calculate resuspension rates. In most experiments, 6000–16000 Class 4 particles were observed in the characterization area (Table S1) on glass or gold surfaces. On CARC surfaces, 3000–7000 Class 4 particles were detected per experiment.
3.4.2. PE particles
Representative images of PE particles on the three different surfaces before and after resuspension are illustrated in Figure S7. As illustrated in , PE particles are quite polydisperse and particles with diameters greater than 50 μm are not all comprised of agglomerates. Both effects made it more challenging to establish the final classification scheme summarized in Table S3. For experiments on CARC surfaces, the aspect ratio criterion was, again, lowered to 0.7 from 0.9, and after the particles were classified manual inspection eliminated surface features that had been misidentified as particles.
Figure 3. The particle size distribution of the PE spheres on a glass surface before and after a resuspension experiment. The images correspond to typical Classes 2, 4, 6, and 8 PE particles.
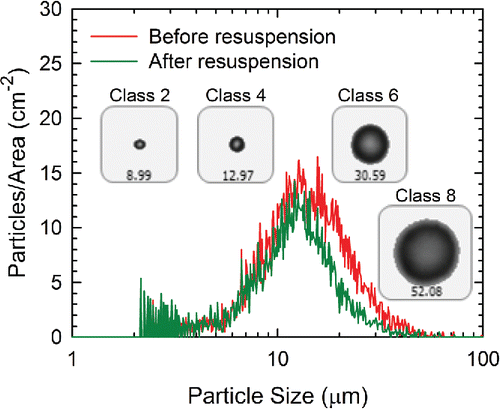
An advantage of the broad size distribution was that each resuspension experiment yielded information on the resuspension of particles in four different classes (Figure S8/Table S3). As expected, the largest (Class 8) spheres were readily resuspended (Figure S7), and even spheres as small as ∼6 μm disappeared from the images after resuspension experiments. In most experiments, the numbers of particles observed in the characterization area ranged from 800 to 3000 for Class 2, 1400 to 6000 for Class 4, 3000 to 8000 for Class 6, and 140 to 450 for Class 8 particles.
3.4.3. Handling losses
To ensure that handling did not induce resuspension, particles were deposited on a surface, analyzed using Morphologi G3S, placed in the quiescent wind tunnel, and then retrieved and reanalyzed. Two handling experiments were conducted for each combination of particles and surfaces. Particle coincidence on before and after images was greater than 99.9998%. Thus, handling did not result in particle loss.
3.5. Resuspension rate measurements
Resuspension rates reported in the literature vary widely, and this variation has been attributed to the broad range of environmental conditions (Sehmel Citation1980; Nicholson Citation1988) or the broad distribution of particle/surface interactions (Phares et al. Citation2000) used in the experiments. Although micro-scale features of the particle-surface interface, including the local water content and chemistry, affect the resuspension process (Loosmore Citation2003), many studies do not report RH present during the experiments (Ibrahim et al. Citation2004; Kim et al. Citation2010). In those studies that do report RH, Corn and Stein Citation(1965) found that adhesion forces were constant up to RH = 30% and increased rapidly after that. Rabinovich et al. Citation(2002) demonstrated both theoretically and experimentally that a critical RH exists where capillary forces are first observed and below which adhesion forces are constant. The critical RH increases with the nanoscale roughness: for a very smooth glass sphere (dia = 20–40 μm, RMS roughness = 0.2 nm), the critical RH increased from ∼20% on a 0.2 nm RMS roughness silica surface to ∼60% on a 0.7 nm RMS roughness silica surface.
Of the more than 400 resuspension rates determined in this study, we will first discuss the largest dataset, i.e., experiments corresponding to tm = 150 s. illustrates the resuspension rates of the ∼22 μm glass particles on all three surfaces as a function of RH. Here, the error bars are based on the uncertainty of ±1.5% in RH only, since the uncertainties in time and particle number on the surface are negligible. At low RH, the resuspension rates are all within an order of magnitude of 10−3 s−1 irrespective of the surface. The data for glass surfaces exhibit the most scatter, those on gold the least scatter. On glass surfaces, resuspension rates start to decrease at ∼50–60% RH and are reduced by ∼2 orders of magnitude at ∼70% RH. For the CARC and gold surfaces, the region of constant resuspension rate extends to somewhat higher RH, ∼ 60%, and the decrease is smaller, ∼1 order of magnitude for CARC and slightly less than 2 orders of magnitude for gold.
Figure 4. Resuspension rates of Class 4 glass spheres as a function of RH. For all experiments, tm = 150 s. Experiments on the glass and the gold surfaces were performed with u* = 0.41 m/s (U∞ = 10.1 m/s), while u* = 0.49 m/s (U∞ = 12.7 m/s) for the CARC surfaces.
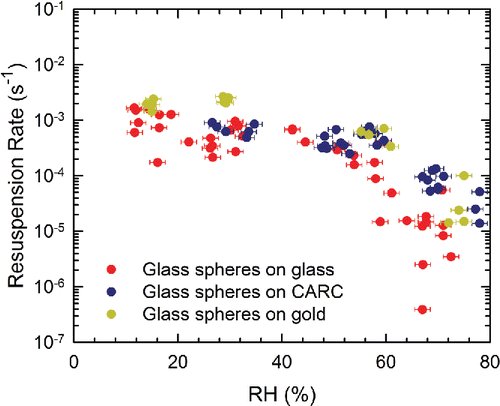
These trends with RH are consistent with Rabinovich et al.'s Citation(2002) concept of a critical RH. In contrast, we expected resuspension rates from the CARC surface, with its micron size roughness elements, would be significantly lower than from the smooth glass or gold surfaces. The fact they are not cannot be explained by the slightly higher friction velocity used during the experiments with CARC surface, because there was no different in the resuspension rates measured on glass surfaces when the friction velocity was increased from 0.41 and 0.49 m/s (Figure S9). Although the Hamaker constant for the glass-CARC combination is ∼25% lower than that for glass-glass and lower van der Waals interactions should lead to higher resuspension, our previous results (Kim et al. Citation2010) suggest that the effect of such a small decrease in the Hamaker constant should not compensate for the 3 order of magnitude increase in surface roughness. In fact, the experiments on glass and gold surfaces emphasize the limited effect that changing the Hamaker constant had on the experiments. Here, both surfaces are of comparable roughness, and the friction velocity was constant. The Hamaker constant for glass-gold is, however, more than twice of that for glass-glass, and, thus, we expected higher resuspension rates on glass surfaces than on gold. Under dry conditions (RH < 40%), however, resuspension rates from gold seem higher than those from glass surfaces.
One factor contributing to this discrepancy may be that the van der Waals force on gold surfaces was substantially reduced by surface contamination arising from exposure to ambient conditions (Kweon et al. Citation2011). Thus, the van der Waals force between the glass particles and contaminated gold surfaces may not have been much higher than that between particles and glass surfaces. Another possible explanation for this counterintuitive result may be that gold surfaces are conductive and electrostatic adhesion forces should be suppressed relative to the glass surfaces, thereby increasing resuspension. Unfortunately, the magnitude of the electrostatic force contribution to resuspension is not clear (Ziskind Citation2006). According to Bowling Citation(1988), van der Waals forces dominate over electrostatic forces for particles smaller than 50 μm, whereas Hays Citation(1991) suggested that the electrostatic image force is comparable to the van der Waals force for a 5 μm toner particle that is deliberately charged. Hubbard et al. Citation(2012) showed that PMMA microspheres did not resuspend from SiO2 surfaces by vibration but resuspended readily from TiO2 surfaces whose conductivity is three orders of magnitude higher than SiO2. They attributed their results to inadequate neutralization of the aerosolized particles despite using a radioactive neutralizer to reduce the induced triboelectric charging of the spheres. They also postulated that electrostatic charge patches are responsible for the lack of resuspension on SiO2 surfaces. Ziskind Citation(2006) suggested that if particles are not deliberately charged and deposited at relatively low velocities, the electrostatic forces are small compared to the van der Waals force. Surface roughness, however, reduces the van der Waals interactions while the electrostatic forces are not affected. The deposition method used here occurs at high velocity (due to the use of a high pressure), and the particles have nanoscale bumps. Although surface and particle charges and, therefore, electrostatic effects were not directly controlled or measured in the current experiments, our results suggest they may play a critical and underexplored role in particle resuspension studies.
For PE particles, we determined the resuspension of single spheres in Classes 2, 4, 6, and 8. Although resuspension rates for Class 2 (∼8 μm) and Class 4 (∼11 μm) particles ranged from 10−4 to 10−3 s−1, the data (Figure S10) are quite scattered, and we suspect saltation by larger particles played a significant role in small particle movement (see the SI). , therefore, only presents the resuspension rates for Class 6 and 8 particles as a function of RH and deposition surface. For these hydrophobic particles there was remarkable little dependence on RH especially when the surface was also hydrophobic. Only Class 6 particles (∼19 μm) on glass showed resuspension rates that decreased significantly when RH > ∼60%. Class 8 particles (∼37 μm) resuspended at higher rates than Class 6 particles on all surfaces consistent with these larger particles penetrating further into the viscous sublayer, and therefore, experiencing higher hydrodynamic removal forces. The observation that RH had little influence on the resuspension of any Class 8 particles is also consistent with the fact that as the particle size increases the ratio of the total surface area in contact with the underlying surface decreases. Thus, changes in the interactions between the particle and the surface due to the presence of water are proportionately less important. If we compare the ∼19 μm Class 6 PE spheres to the ∼22 μm Class 4 glass particles, the effect of RH on resuspension of PE particles was much lower, with some influence on glass surfaces, but almost none for CARC.
Figure 5. Resuspension rates of Classes 6 and 8 PE particles as a function of RH. For all experiments, tm = 150 s. Experiments on glass and the gold surfaces were performed with u* = 0.41 m/s (U∞ = 10.1 m/s), while u* = 0.49 m/s (U∞ = 12.7 m/s) for the CARC surfaces.
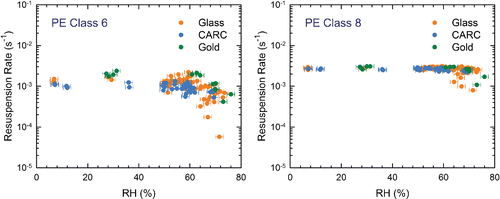
Taken together, the results of and demonstrate that for hydrophilic ∼20 μm particles humidity starts to lower the particle resuspension at ∼50–60% RH, and the biggest effects are observed on hydrophilic surfaces. In contrast, resuspension of the hydrophobic particles on hydrophobic surfaces was hardly affected by humidity.
Our RH-dependent resuspension rates are also consistent with adhesion force measurements using AFM. Pull-off forces measured for 2 μm and 5 μm silica spheres on silica surface showed that adhesion force was constant up to 30% RH before increasing with increasing RH (Paajanen et al. Citation2006). Biggs et al. Citation(2002) measured adhesion force between 15.5 μm glass spheres on a glass surface and found that the pull-off force was steady when RH < 60%. Above 60%, the pull-off force increased by factors of 5–7. Jones et al. Citation(2002) showed that the pull-off force for 40 μm glass spheres (20 μm radius) on hydrophilic glass surface was almost constant up to ∼40% RH and then monotonically increased with RH. In contrast, the pull-off force between 40 μm glass spheres on glass or silicon surfaces treated to be hydrophobic was constant over the entire range of RH. The influence of humidity on sliding or rolling particles (140 μm < dp < 260 μm) has also been demonstrated by the avalanche angle of granular media. These experiments showed that the kinetics of humidity-induced capillary condensation was responsible for aging response (increased avalanche angle) with time and a large hysteresis (Bocquet et al. Citation1998; Restagno et al. Citation2002).
Under the standard approximations, the capillary force model predicts that that RH plays no role (Israelachvili Citation1991) when the radius of the particle is larger than 1 μm and the particle and the surfaces are in contact (Pakarinen et al. Citation2005; Paajanen et al. Citation2006). As noted above, however, some studies have found that adhesion forces for particles lager than 2 μm are influenced by RH. This discrepancy has been explained by the presence of nanoscale asperities on surfaces that affects capillary bridge formation (Jones et al. Citation2002; Butt and Kappl Citation2009). At low humidity, a single capillary bridge is formed at the outmost asperities. At intermediate humidity more capillary bridges are formed, and the capillary force is linearly dependent on the liquid volume of all the bridges combined. At high humidity, the menisci merge into one continuous capillary bridge, the macroscopic geometry determines the total capillary forces, and the surface roughness will no longer play a significant role (Bocquet et al. Citation1998; Halsey and Levine Citation1998; Farshchi-Tabrizi et al. Citation2006; Butt and Kappl Citation2009). The precise shape of the surfaces on the length scale of the Kelvin length will determine whether all three regimes exist and the dependence on humidity (Butt and Kappl Citation2009). The Kelvin length, which characterizes the range of capillary forces, of water at 25°C is 0.54 nm (Butt and Kappl Citation2009). Therefore, surface roughness of ∼1 nm affects the capillary force, and even for the very smooth glass and gold surfaces, the roughness is greater than 1 nm. For hydrophobic surfaces, a continuous water film may not be formed even at high humidity (Jones et al. Citation2002; Szori et al. Citation2010). Instead localized water films will form as favored by heterogeneity of the surface chemistry or geometry (Jones et al. Citation2002).
3.6. Comparing resuspension rates and further analyses
Particle resuspension rates depend on many parameters including particle and surface properties, friction velocity, and time. To further test the consistency of all of our data and to account for the differences in time between experiments we turned to the semiempirical resuspension model previously developed by Kim et al. Citation(2010)[2] and examined the behavior of Λpredicted/Λexperiment. In Equation Equation(2)
[2] , Λpredicted is a function of the particle, surface, and flow field properties whose values are summarized in Table S3 in the SI. The model parameters were derived in our earlier work by fitting to the data of Ibrahim Citation(2004) and Nicholson Citation(1993), and further details regarding model development and the data used to test the model are available in Kim et al. Citation(2010). As discussed by Reeks et al. Citation(1988), the inverse relationship between t and Λ should be valid for times between 10−2 s and 105 s. Furthermore, the inverse relationship between z0 and Λ, comparable to that found by Loosmore Citation(2003), may be understood by recognizing that it is the result of competing phenomena. In particular, resuspension should increase as the surface roughness increases because the van der Waals adhesion forces are greatly reduced (Ziskind Citation2006). Surface roughness, however, also affects fluid dynamics, and particles are increasingly shielded from the flow as the surface roughness increases (Loosmore Citation2003) especially when z0 > dp.
and summarize Λpredicted/Λexperiment as a function of RH for all of the experiments conducted here with glass spheres and PE spheres, respectively. The horizontal error bars reflect the uncertainty in RH while the vertical error bars are based on the uncertainties in the particle size, the aerodynamic surface roughness, the friction velocity, and the air density. Particle size and surface roughness account for more than 90% of the uncertainty. Particle density (reported by the manufacturer) and the Hamaker constant are assumed to have negligible uncertainty. The larger variability in the glass on glass experiments may be due to variability in the strength of the capillary forces as liquid bridges form between the irregularly, rough spheres and the smooth surfaces that exhibit nanoscale asperities (Bocquet et al. Citation1998; Riedo et al. Citation2002; Prokopovich and Starov Citation2011).
Figure 6. The ratio of Λpredicted to Λexperiment as a function of RH for Class 4 glass particles (Cospheric 18 – 22 μm, Class 4) resuspended from glass, CARC, and gold surfaces. For the glass surfaces, tm = 60, 150, or 300 s, and u* was either 0.41 m/s (U∞ = 10.1 m/s) or 0.49 m/s (U∞ = 12.7 m/s). For the CARC surfaces, tm was also 60, 150, or 300 s, but u* was fixed at 0.49 m/s. For the gold surfaces, tm was 150 s and u* was 0.41 m/s. The solid line shows a correlation between Λpredicted/Λexperiment and RH for the combined data of glass, CARC, and gold surfaces when RH > 55%.
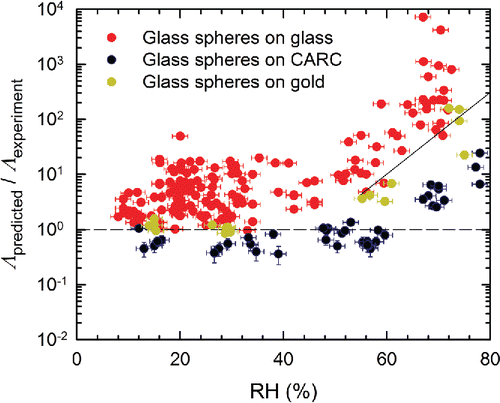
Figure 7. The ratio Λpredicted/Λexperiment as a function of RH for PE particles resuspended from glass, CARC, and gold surfaces. For all experiments, tm = 150 s. Experiments on glass and the gold surfaces used u* = 0.41 m/s (U∞ = 10.1 m/s), while u* = 0.49 m/s (U∞ = 12.7 m/s) for the CARC surfaces.
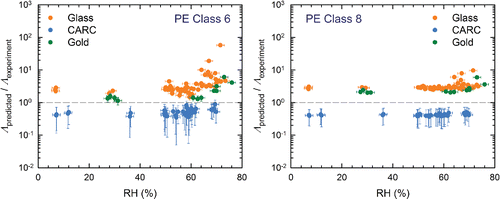
For glass spheres (), Λpredicted/Λexperiment is close to 1 at lower RH, increasing drastically with RH once RH > ∼55%. As indicated by the black line, these data suggest that a simple correction factor to Equation Equation2[2] should improve the model for glass spheres independent of the surface. Since a power law relationship for RH similar to Equation Equation(2)
[2] , i.e., (Λpredicteddp/u*) ∝ (1+αRHβ), did not fit well, we derived the following simplified correction factor,
[3] by fitting all of the glass particle data. Although resuspension of Class 6 PE spheres on glass and gold surfaces () shows some dependency on RH above ∼70%, it is neither severe enough, nor consistent enough to warrant an additional correction factor.
Considering that the semi-empirical model (Equation (2)) was developed independently of this study, and was based on the experiments of other researchers, the current experimental resuspension rates are generally well predicted at low RH. The relatively large scatter in the data for glass spheres on glass surfaces, suggests that there is still room for improvement—both in the model and the experiments. The values of Λpredicted/Λexperiment on CARC surfaces are also consistently lower than those a glass—an effect that is pronounced for PE particles but can also be seen for glass particles. Since the Hamaker constants for glass and CARC are not much different, the shift in the values of Λpredicted/Λobtained from glass and CARC could be due to an inadequate correction for surface roughness. Our observation is also supported by the data of Kassab et al. Citation(2013) where Equation Equation(2)[2] underpredicted the resuspension rates for rough, but not a smooth, surfaces. It also suggests that the dependence of Λ on z0 in Equation Equation(2)
[2] should be decreased to more accurately reflect the lower adhesion forces between particles and rough surfaces.
Finally in we compare our data to other measurements available in the literature—both in terms of measured resuspension rates and Λpredicted/Λexperiment. Since measurements were only made for tm = 60, 150, and 300 s, many of the current data points overlap when the resuspension rates are plotted as a function of time. Including all of the data in our resuspension rates at fixed time cover a range comparable to those of Nicholson Citation(1993), Ibrahim Citation(2004), and Reeks and Hall Citation(2001). When we only consider the data for RH less ∼55%, the scatter in our data are significantly reduced.
Figure 8. (a) Experimental resuspension rates and (b) Λpredicted/Λexperiment obtained in this study are compared to existing literature data. For PE particles, the tm values are shifted either +30% or −25% so that the glass particle data are visible. In (a) for glass spheres, only those points for the current experiments where RH is less than 55% are shown as filled circles, and the range of values corresponding to the high humidity conditions is indicated by a dashed vertical line. In (b) we have included the humidity correction, Equation Equation(3)[3] , for our glass sphere data, thus both high and low RH points appear as solid circles. corresponding to the high humidity conditions is indicated by a dashed vertical line. In (b) we have included the humidity correction, Equation Equation(3)
[3] , for our glass sphere data, thus both high and low RH points appear as solid circles.
![Figure 8. (a) Experimental resuspension rates and (b) Λpredicted/Λexperiment obtained in this study are compared to existing literature data. For PE particles, the tm values are shifted either +30% or −25% so that the glass particle data are visible. In (a) for glass spheres, only those points for the current experiments where RH is less than 55% are shown as filled circles, and the range of values corresponding to the high humidity conditions is indicated by a dashed vertical line. In (b) we have included the humidity correction, Equation Equation(3)[3] , for our glass sphere data, thus both high and low RH points appear as solid circles. corresponding to the high humidity conditions is indicated by a dashed vertical line. In (b) we have included the humidity correction, Equation Equation(3)[3] , for our glass sphere data, thus both high and low RH points appear as solid circles.](/cms/asset/a299e23e-8472-4e29-9c7b-53b1d728078b/uast_a_1152350_f0008_oc.gif)
illustrates the ratio Λpredicted/Λexperiment with respect to time evaluated using Equation Equation(2)[2] for the existing literature data and Equations Equation(2)
[2] and (Equation3
[3] ) for our data. Values of the parameters required to evaluate Λpredicted for the data of Giess et al. Citation(1997) through Wen and Kasper Citation(1989) are available in Kim et al. Citation(2010), with the exception that here we reduced the value of z0 for the glass surfaces of Braaten et al. Citation(1990) and Wu et al. Citation(1992) from 10−6 m to 10−9 m since the lower value is more consistent with our own measurements as well as those of Ibrahim et al. Citation(2003). The parameters used to evaluate Equation Equation(2)
[2] for the conditions studied in this article, Reeks and Hall Citation(2001), and Ould-Dada and Baghini Citation(2001) can be found in the SI (Table S3).
Applying the modified model to our data we find that 97% of our points lie in the range 0.3 < Λpredicted/Λexperiment < 30. For the data of Braaten et al., Wu et al., and Reeks and Hall, >60% of the data fall with the range 1 < Λpredicted/Λexperiment < 100. Thus, the model predicts these experimental data relatively well. We note that these four sets of experiments all used particles in the 10—30 μm range. Since the model was derived by fitting the 30—70 μm particle data of Ibrahim Citation(2004), and some 4—22 μm particle data of Nicholson Citation(1993), it is perhaps not so surprising that the model does best for the 10—30 μm particles. This has been also corroborated by the recent study by Kassab et al. Citation(2013). Furthermore, it is encouraging that the results from experiments lasting between 1 and 1000 s agree so well after accounting for time.
The remaining four studies yield Λpredicted/Λexperiment < ∼0.1. The most extreme cases are the resuspension rates measured by Krauter and Biermann Citation(2007) and Ould-Dada and Baghini Citation(2001), where resuspension rates are 3–4 orders of magnitude higher than predicted by the model or obtained in this study. The high resuspension rates measured by Krauter and Biermann, may reflect their use of fluidized bacterial spores (dp = 0.6–1.1 μm) with aerodynamic characteristics similar to weaponized spores specifically designed to remain airborne for prolonged periods of time and to resuspend easily. In contrast, Ould-Dada and Baghini measured resuspension rates of ∼1 μm silica particles from ∼40 cm tall Norway spruce saplings in a 1.2 m high and 0.8 m wide wind tunnel. The turbulence in the wind tunnel induced by the presence of these large objects is quite different than the turbulence found in our more conventional wind tunnel. Indeed, the turbulence intensity within the canopy was 20–38%, levels that are much higher than those we measured ∼3 mm above the surface. Turbulence bursts contribute significantly to particle resuspension (Cleaver and Yates Citation1975; Soltani and Ahmadi Citation1994; Zhang et al. Citation2013) as does increasing turbulence intensity (Lengweiler Citation2000; Mukai et al. Citation2009). Thus, higher turbulence levels are consistent with the higher resuspension rates measured by Ould-Dada and Baghini.
The results presented here clearly demonstrate how RH and particle and surface properties influence resuspension. For hydrophobic surfaces, such as CARC painted vehicles or most plastic surfaces, the effect of RH can be neglected for RH < 80%. For other surfaces, limiting the RH to below a critical RH ensures good agreement between experiments and the model. Finally, our results show that incorporating the dependence on RH on resuspension rates above a critical RH value can improve the model.
4. Summary and conclusions
We measured resuspension rates of hydrophilic glass spheres and hydrophobic PE spheres from clean glass, CARC coated aluminum, and gold coated glass surfaces in a wind tunnel. The particles had nanoscale bumps on their surfaces comparable to environmental particles. The surfaces ranged from smooth and hydrophilic to rough and hydrophobic.
Resuspension experiments were conducted as a function of RH, and at low RH resuspension rates were reasonably similar for ∼20 μm glass and PE particles on all the surfaces. When RH increased, the resuspension rates of glass particles from glass surfaces decreased by almost three orders of magnitude between ∼55% and ∼70% RH. With glass particles on gold and CARC surfaces a two order of magnitude reduction in resuspension rate was observed when the RH approached 80%. PE particles on glass or gold surfaces exhibited less dependency on RH than glass particles on the same surfaces. Resuspension rates of PE particles on CARC were almost independent of RH.
In summary, resuspension rates of particles from surfaces were affected both by RH and surface properties. Resuspension rates of hydrophilic particles on hydrophilic surfaces were affected by RH the most and those of hydrophobic particles on hydrophobic surfaces the least. Furthermore, as proposed by Rabinovich et al. Citation(2002), there was a critical RH, where capillary forces are first observed and below which adhesion forces are constant. The critical RH is a function of particle and surface properties, and the macroscopic phenomenon of resuspension clearly reflects the underlying microscopic processes. For glass particles, incorporating the effect of RH on resuspension rates improved the model.
Nomenclature
A132 | = | Hamaker constant |
N(t) | = | the number of particles on surface at time t |
dp | = | particle diameter |
t0 | = | time at the start of a resuspension experiment |
te | = | time at the end of a resuspension experiment |
tm | = | time at the mid-point of a resuspension experiment |
u* | = | friction velocity |
U∞ | = | freestream velocity |
x | = | axial position in the wind tunnel |
y | = | cross-stream position in the wind tunnel |
z | = | vertical position in the wind tunnel |
z0 | = | aerodynamic surface roughness length |
Λexperiment | = | experimental resuspension rate |
Λpredicted | = | predicted resuspension rate |
ρ | = | fluid density |
ρa | = | air density |
ρp | = | particle density |
τw | = | wall shear stress |
Supplementary_Information.docx
Download MS Word (5 MB)Funding
Support for this work was provided by the Defense Threat Reduction Agency under contract HDTRA1–10-C-0069. The views expressed herein are those of the authors and not necessarily those of the Department of Defense, the Defense Threat Reduction Agency, or any other agency or component of the US Government.
References
- Aptowicz, K. B., and Chang, R. K. (2005). Angularly-Resolved Elastic Scatter from Single Particles Collected over a Large Solid Angle and with High Resolution. J. Phys.: Conf. Ser., 6:90–96.
- Ata, A., Rabinovich, Y. I., and Singh, R. K. (2002). Role of Surface Roughness in Capillary Adhesion. J. Adhesion Sci. Tech., 16:337–346.
- Bechert, D. W. (1996). Calibration of Preston Tubes. AIAA J., 34:205–206.
- Biggs, S., Cain R. G., Dagastine, R.R., and Page, N. W. (2002). Direct Measurements of the Adhesion Between a Glass Particle and a Glass Surface in a Humid Atmosphere. J. Adhesion Sci. Technol., 16: 869–885.
- Bocquet, L., Charlaix, E., Ciliberto, S., and Crassous J. (1998). Moisture-Induced Ageing in Granular Media and the Kinetics of Capillary Condensation. Nature, 396:735–737.
- Boor, B. E., Siegel, J. A., and Novoselac, A. (2011). Development of an Experimental Methodology to Determine Monolayer and Multilayer Particle Resuspension from Indoor Surfaces. ASHRAE Trans., 117:434–441.
- Bowling, R.A. (1988). A Theoretical Review of Particle Adhesion, in Particles on Surfaces I: Detection, Adhesion and Removal, K. L. Mittal, ed., Plenum Press, New York. pp. 129–142.
- Braaten, D. A. (1994). Wind Tunnel Experiments of Large Particle Reentrainment-Deposition and Development of Large Particle Scaling Parameters. Aerosol Sci. Technol., 21:157–169.
- Braaten, D. A., Paw, U. K. T., and Shaw, R. H. (1990). Particle Resuspension in a Turbulent Boundary Layer – Observed and Modeled. J. Aerosol Sci., 21:613–628.
- Butt, H. J., and Kappl, M. (2009). Normal Capillary Forces. Adv. Coll. Interface Sci., 146:48–60.
- Cleaver, J. W., and Yates, B. (1975). A Sub-Layer Model for the Deposition of Particles from a Turbulent Flow. Chem. Eng. Sci., 30:983–992.
- Corn, M. (1961). The Adhesion of Solid Particles to Solid Surfaces, I. A Review. J. Air Pollut. Cont. Assoc., 11:523–528.
- Corn, M., and Stein, F. (1965). Re-entrainment of Particles from a Plane Surface. Am. Ind. Hyg. Assoc. J., 26:325–336.
- Corsi, R. L., Siegel, J. A., and Chiang, C. (2008). Particle Resuspension during the Use of Vacuum Cleaners on Residential Carpet. J. Occup. Environ. Hygiene., 5: 232–238.
- Dillon, M. B. (2011). Skin as a Potential Source of Infectious Foot and Mouth Disease Aerosols. Proc. R. Soc. B, 278:1761–1769.
- Farshchi-Tabrizi, M., Kappl, M., and Butt, H. J. (2008). Influence of Humidity on Adhesion: An Atomic Force Microscope Study. J. Adh. Sci. Technol., 22: 181–203.
- Farshchi-Tabrizi, M., Kappl, M., Cheng, Y., Gutmann, J., and Butt, H. J. (2006). On the Adhesion Between Fine Particles and Nanocontacts: an Atomic Force Microscope Study. Langmuir, 28:2171–2184.
- Giess, P., Goddard, A. J. H., and Shaw, G. (1997). Factors Affecting Particle Resuspension from Grass Swards. J. Aerosol Sci., 28:1331–1349.
- Goldasteh, I., Ahmadi, G., and Ferro, A. (2012). A Model for Removal of Compact, Rough, Irregularly Shaped Particles from Surfaces in Turbulent Flows. J. Adh., 88:766–786.
- Gomes, C., Freihaut, J., and Bahnfleth, W. (2007). Resuspension of Allergen-Containing Particles Under Mechanical and Aerodynamic Disturbances from Human Walking. Atmos. Environ., 41:5257–5270.
- Halsey, T. C., and Levine, A. J. (1998). How Sandcastles Fall. Phys. Rev. Lett., 80:3141–3144.
- Hays, D. (1991). Role of Electrostatics in Adhesion, in Fundamentals of Adhesion, L. H. Lee, ed., Plenum Press, New York, pp. 249–278.
- Hubbard, J. A., Brockmann J. E., Rivera D., and Moore D. G. (2012). Experimental Study of Impulse Resuspension with Laser Doppler Vibrometry. Aerosol Sci. Technol., 46:1303–1312.
- Ibrahim, A. H. (2004). Microparticle Detachment from Surface by Fluid Flow. Ph.D. thesis, University of Notre Dame, Notre Dame, Indiana. (Available for download from http://etd.nd.edu/ETD-db/theses/available/etd-01302004–105607/)
- Ibrahim, A. H., Dunn, P. F., and Brach, R. M. (2003). Microparticle Detachment from Surfaces Exposed to Turbulent Air Flow: Controlled Experiments and Modeling. J. Aerosol Sci., 34:765–782.
- Ibrahim, A. H., Dunn, P. F., and Brach, R. M. (2004). Microparticle Detachment from Surfaces Exposed to Turbulent Air Flow: Effects of Flow and Particle Deposition Characteristics. J. Aerosol Sci., 35:805–821.
- Israelachvili, J. (1991). Intermolecular and Surface Forces, 2nd ed., Academic Press, London.
- Jones, A. M., and Harrison, R. M. (2004). The Effects of Meteorological Factors on Atmospheric Bioaerosol Concentrations - A Review. Sci. Total Environ. 326:151–180.
- Jones, R., Pollock, H. M., Cleaver, J. A. S., and Hodges, C. S. (2002). Adhesion Forces Btween Glass and Silicon Surfaces in Air Studied by AFM: Effects of Relative Humidity, Particle Size, Roughness, and Surface Treatment. Langmuir, 18:8045–8055.
- Kassab, A. S., Ugaz, V. M., King, M. D., and Hassan, Y. A. (2013). High Resolution Study of Micrometer Particle Detachment on Different Surfaces. Aerosol Sci. Technol., 47:351–360.
- Kim, Y., Gidwani, A., Wyslouzil, B. E., and Sohn, C. W. (2010). Source Term Models for Fine Particle Resuspension from Indoor Surfaces. Build. Environ., 45:1854–1865.
- Krauter, P., and Biermann, A. (2007). Reaerosolization of Fluidized Spores in Ventilation Systems. Appl. Environ. Microbiol., 73:2165–2172.
- Kweon, H., Yiacoumi, S., and Tsouris, C. (2011). Friction and Adhesion Forces of Bacillus thuringiensis Spores on Planar Surfaces in Atmospheric Systems. Langmuir, 27:14975–14981.
- Lengweiler, P. (2000). Modelling Deposition and Resuspension of Particles on and from Surfaces. Ph.D. thesis, Swiss Federal Institute of Technology, Zürich, Switzerland. (Available for download from http://e-collection.ethbib.ethz.ch/eserv/eth:23702/eth-23702–02.pdf)
- Loosmore, G. A. (2003). Evaluation and Development of Models for Resuspension of Aerosols at Short Times after Deposition. Atmos. Environ., 37:639–647.
- Mukai, C., Siegel, J. A., and Novoselac, A. (2009). Impact of Airflow Characteristics on Particle Resuspension from Indoor Surfaces. Aerosol Sci. Technol., 43: 1022–1032.
- Nicholson, K. W. (1988). A Review of Particle Resuspension. Atmos. Environ., 22:2639–2651.
- Nicholson, K. W. (1993). Wind Tunnel Experiments on the Resuspension of Particulate Material. Atmos. Environ., 27A:181–188.
- Oberoi, R. C., Choi, J.-I., Edwards, J. R., Rosati, J. A., Thornburg, J., and Rodes, C. E. (2010). Human-Induced Particle Re-Suspension in a Room. Aerosol Sci. Technol., 44: 216–229.
- Orgill, M. M., Sehmel, G. A., and Petersen, M. R. (1976). Some Initial Measurements of Airborne DDT over Pacific Northwest Forests. Atmos. Environ., 10:827–834
- Ould-Dada, Z., and Baghini, N. M. (2001). Resuspension of Small Particles from Tree Surfaces. Atmos. Environ., 35:3799–3809.
- Paajanen, M., Katainen, J., Pakarinen, O. H., Foster, A. S., and Lahtinen, J. (2006). Experimental Humidity Dependency of Small Particle Adhesion on Silica and Titania. J. Colloid Interface Sci., 304:518–523.
- Pakarinen, O. H., Foster, A. S., Paajanen, M., Kalinainen, T., Katainen, J., Makkonen, I., Lahtinen, J., and Nieminen, R. M. (2005). Towards an Accurate Description of the Capillary Force in Nanoparticle-Surface Interactions. Modelling Simul. Mater. Sci. Eng., 13:1175–1186.
- Phares, D. J., Smedley, G. T., and Flagan, R. C. (2000). Effect of Particle Size and Material Properties on Aerodynamic Resuspension from Surfaces. J. Aerosol Sci., 31:1335–1353.
- Plomp, M., Leighton, T. J., Wheeler, K. E., and Malkin, A. J. (2005). The High-Resolution Architecture and Structural Dynamics of Bacillus Spores. Biophys. J., 88:603–608.
- Prokopovich, P., and Starov, V. (2011). Adhesion Models: From Single to Multiple Asperity Eontacts. Adv. Colloid Interface Sci. 168:210–222.
- Qian, J., and Ferro, A. R. (2008). Resuspension of Dust Particles in a Chamber and Associated Environmental Factors. Aerosol Sci. Technol., 42: 566–578.
- Rabinovich, Y. I., Adler, J. J., Esayanur, M. S., Ata, A., Singh, R. K., and Moudgil, B. M. (2002). Capillary Forces Between Surfaces with Nanoscale Roughness. Adv. Coll. Interf. Sci., 96:213–230.
- Reeks, M. W., and Hall, D. (2001). Kinetic Models for Particle Resuspension in Turbulent Flows: Theory and Measurement. J. Aerosol Sci., 32:1–31.
- Reeks, M. W., Reed, J., and Hall, D. (1988). On the Resuspension of Small Particles by a Turbulent Flow. J. Phys. D: Appl. Phys., 21:574–589.
- Riedo, E., Levy, F., Brune, H. (2002). Kinetics of Capillary Condensation in Nanoscopic Sliding Friction. Phys. Rev. Lett., 88:1855051–1855054.
- Restagno, F., Bocquet, L., Crassous, J., and Charlaix, E. (2002). Slow Kinetics of Capillary Condensation in Confined Geometry: Experiment and Theory. Colloids Surf. A, 206:69–77.
- Sander, M., Patterson, R. I. A., Raj, A., and Kraft, M. (2010). Comment on “Low Fractal Dimension Cluster-Dilute Soot Aggregates from a Premixed Flame.” Phys. Rev. Lett., 104:119601.
- Sehmel, G. A. (1980). Particle Resuspension: A Review. Envir. Int., 4:107–127.
- Smith, T. (1980). The Hydrophilic Nature of a Clean Gold Surface. J. Colloid Interf. Sci., 75:51–55.
- Soltani, M., and Ahmadi, G. (1994). On Particle Adhesion and Removal Mechanisms in Turbulent Flows. J. Adh. Sci. Technol., 8:763–785.
- Szori, M., Jedlovszky, P., and Roeselová, M. (2010). Water Adsorption on Hydrophilic and Hydrophobic Self-Assembled Monolayers as Proxies for Atmospheric Surfaces. A Grand Canonical Monte Carlo Simulation Study. Phys. Chem. Chem. Phys., 12:4604–4616.
- Weis, C. P., Intrepido, A. J., Miller, A. K., Cowin, P. G., Durno, M. A., Gebhardt, J. S., and Bull, R. (2002). Secondary Aerosolization of Viable Bacillus anthracis Spores in a Contaminated US Senate Office. J. Am. Med. Assoc., 288:2853–2858.
- Wen, H. Y., and Kasper, G. (1989). On the Kinetics of Particle Reentrainment from Surfaces. J. Aerosol Sci., 20:483–498.
- Wu, Y.-L., Davidson, C. I., and Russell, A. G. (1992). Controlled Wind Tunnel Experiments for Particle Bounceoff and Resuspension. Aerosol Sci. Technol., 17:245–262.
- Zhang, F., Reeks, M., and Kissane, M. (2013). Particle Resuspension in Turbulent Boundary Layers and the Influence of Non-Gaussian Removal Forces. J. Aerosol Sci., 58:103–128.
- Ziskind, G. (2006). Particle Resuspension from Surfaces: Revisited and Re-evaluated. Rev. Chem. Eng., 22:1–123.