ABSTRACT
The flat surface of Nuclepore filters is suitable for observing collected particles with a scanning electron microscope (SEM). However, experimental data on surface-collection efficiency are limited because surface-collection efficiencies cannot be measured directly using aerosol measuring instruments. In this study, the surface-collection efficiencies of Nuclepore filters were determined by establishing the ratio of the number of particles deposited on the surface of the filter visually counted with an SEM to the number of inflow particles counted by a condensation particle counter, using monodispersed polystyrene latex particles (30–800 nm) and silver particles (15–30 nm). Because Nuclepore filters with smaller pore sizes would be expected to produce higher minimum surface-collection efficiency and a higher pressure-drop, 0.08 and 0.2 µm Nuclepore filters were chosen as the test filters in view of both collection efficiency and pressure drop. The results showed that the minimum surface-collection efficiencies of the 0.08 µm pores at face velocities of 1.9 and 8.4 cm·s−1 were approximately 0.6 and 0.7, respectively, and those of the 0.2 µm pores at face velocities of 1.5 and 8.6 cm·s−1 were approximately 0.8 and 0.6, respectively. Because the pressure drop of the 0.2 µm pore filter was lower than that of the 0.08 µm pore filter under the same flow-rate conditions, the 0.2 µm pore filter would be more suitable considering the pressure drop and collection efficiency. The obtained surface collection efficiencies were quantitatively inconsistent with theoretical surface-collection efficiencies calculated using conventional theoretical models developed to determine the collection efficiency of filters with larger pores.
© 2016 American Association for Aerosol Research
EDITOR:
1. Introduction
An electron microscope is an effective tool for analyzing aerosol particles; it can accurately determine particle size and morphology. For example, in studies on the risks associated with nanomaterials, electron microscope observation is a reliable, and often the only way, to verify the presence and form of aerosolized nanomaterials, and to differentiate between nanomaterials and background particles (Methner et al. Citation2010, Citation2012). Both scanning electron microscope (SEM) and transmission electron microscopy (TEM) are often used for observing aerosolized nanomaterials (Brouwer et al. Citation2009; Kuhlbusch et al. Citation2011). The SEM has a cost advantage and produces three-dimensional (3D) images, which provide more information about the shape of features, while the TEM produces higher resolution (but 2 D flat) images.
The particle sampling methods for SEM observation are generally easier than those for TEM observation because in the latter case it is necessary to load the aerosol particles onto a specific TEM grid. A simple sampling method for SEM observation is to collect aerosol particles by means of a Nuclepore filter, which is a polycarbonate membrane having a flat surface with many circular holes (pores) of uniform size. The flat surface of Nuclepore filters is suitable for observing collected particles.
Aerosol particles are collected on the surface or inside the pores of Nuclepore filters. Many experimental studies have been conducted to determine the overall collection efficiency of Nuclepore filters (Spurny et al. Citation1969; Liu and Lee Citation1976; Smith et al. Citation1976; Montassier et al. Citation1996; Gentry et al. Citation1982; John et al. Citation1983). However, experimental data focused only on surface-collection efficiency are limited (Cyrs et al. Citation2010; Chen et al. Citation2013). This is because the surface-collection efficiency cannot be measured directly using aerosol measuring instruments. Even so, knowing the surface-collection efficiency is important, especially when the number concentration and size distribution of particles in the air are estimated from the number and size of particles deposited on the filter surface, determined with an SEM. In addition, when verifying the absence (and presence) of nanomaterials in the air, the lower limit of detection calculated using the minimum surface-collection efficiency is important.
Cyrs et al. Citation(2010) experimentally determined the surface collection efficiencies of Nuclepore filters using polydispersed potassium chloride (KCl) particles (). The collection efficiencies were derived from the surface loadings of particles on the filter, measured with an SEM, in consideration of the number and size of inflow particles measured with a scanning mobility particle sizer. The tested filters were 0.4 and 0.8 µm pore Nuclepore filters, which had actual pore sizes of 0.29 and 0.72 µm and pore densities of 9.7 ×107 and 1.8 ×107 pores·cm−2, respectively. The minimum surface collection efficiencies obtained experimentally at face velocities of 3.7 and 18.4 cm·s−1 were between 0.15 and 0.27 for a particle size of around 42 or 75 nm (). Although there was inconsistency between the experimental results of the surface-collection efficiency and theoretical model calculations, this could be attributed to the fact that collection by interception was not included in their model for calculating the filter surface collection.
Table 1. Structural characteristics of Nuclepore filters, test conditions for collection efficiency, and minimum surface-collection efficiency.
Chen et al. Citation(2013) also experimentally determined the surface collection efficiency of 1 µm pore Nuclepore filters using monodispersed polystyrene latex (PSL) spherical particles at a face velocity of 5 cm·s−1 (. The surface collection efficiency for PSL particles with a diameter of 80 nm, which was around the minimum collection-efficiency diameter, was approximately 0.3. Their results showed that the experimentally determined surface-collection efficiency was in good agreement with the theoretical model calculations.
In this work, we conducted tests for the surface-collection efficiencies of Nuclepore filters with smaller pore sizes, which would be expected to produce higher minimum surface-collection efficiency. Because smaller pore sizes also cause a higher pressure-drop, we chose 0.08 and 0.2 µm Nuclepore filters as the test filters in view of both collection efficiency and pressure drop. Using monodispersed PSL and silver (Ag) particles, the surface-collection efficiency of each filter was determined by establishing the ratio of the number of particles deposited on the surface of the filter visually counted with an SEM to the number of inflow particles counted by a condensation particle counter (CPC). We compared conventional theoretical model calculations against the experimental results. The major purposes of this study are (1) to investigate the surface-collection efficiencies of 0.08 and 0.2 µm Nuclepore filters by particle size, including the minimum surface-collection efficiencies and the influence of face velocity and particle density on the collection efficiencies, (2) to understand the filtration mechanisms for particle collection by 0.08 and 0.2 µm Nuclepore filters by comparing the experimental and theoretical efficiencies to help interpret the experimental results, and (3) to examine whether conventional theoretical models are capable of estimating the surface-collection efficiencies of 0.08 and 0.2 µm Nuclepore filters.
2. Materials and methods
2.1. Filters and test conditions
We used 25 mm Whatman® Nuclepore track-etched polycarbonate membranes having pores with nominal pore sizes of 0.08 and 0.2 µm (110604 and 110606; GE Healthcare UK, Amersham Place, Little Chalfont, Buckinghamshire, England). The actual pore diameters for the two filter types measured with a field-emission SEM (Quanta 250 FEG, FEI Company, Hillsboro, OR, USA) were 0.059 ± 0.008 and 0.17 ± 0.01 µm, respectively (mean ± standard deviation). The measured pore densities were 7.0 ×108 and 2.9 ×108 pores·cm−2, respectively. Accordingly, the filter porosities were 1.9% and 6.3%, respectively. The filter thicknesses reported by the manufacturer are 6 and 10 µm, respectively. The structural characteristics of the filters are summarized in .
A 25 mm stainless steel in-line filter holder (effective filtration area of 3.7 cm2, P/N 1209, Pall Corporation, Port Washington, NY, USA) was used to mount the Nuclepore filters and test the surface-collection efficiency of particles. The flow rates were set at 0.3 or 1.0 L·min−1 for the 0.08 µm pore filter, and 0.3 or 1.5 L·min−1 for the 0.2 µm pore filter. The apparent face velocities (=flow rate/effective filtration area) at flow rates of 0.3, 1.0, and 1.5 L·min−1 were 1.4, 4.5, and 6.8 cm·s−1, respectively.
The filter support screen for the filter holder has circular pores with diameter of 175 µm and pore density of 1.6 × 103 pores·cm−2 (porosity of 38%). The filter support screen is necessary for reliable sampling because the Nuclepore filters are thin and soft. However, the filter support screen may block the exits of some pores. Therefore, differences in the pressure-drops for conditions with and without the filter support screen were checked in advance (). The pressure-drop of the filter with the filter support screen for each test condition was higher than that without the filter support screen. Note that the pressure-drop of the filter support screen itself was negligible. The ratio of the pressure-drops with the filter support screen to that without it increased as the flow rate increased. By multiplying the apparent face velocity by the ratio of the aforementioned pressure-drops, an effective face velocity for each test condition was estimated by assuming that the face velocity is almost proportional to the pressure-drop. The obtained effective face velocities (hereafter, simply referred to as face velocities) at flow rates of 0.3 and 1.0 L·min−1 for the 0.08 µm pore filter were 1.9 and 8.4 cm·s−1, respectively, and those at flow rates of 0.3 and 1.5 L·min−1 for the 0.2 µm pore filter were 1.5 and 8.6 cm·s−1, respectively.
Table 2. Pressure-drops of filters with and without filter support screenaFootnotea.
2.2. Experimental setup for evaluating the collection efficiency
The experimental setup used for evaluating the collection efficiency of the Nuclepore filters is shown in . Conductive silicone tubing and stainless steel connectors were used to transmit the particles. The collection efficiencies of the Nuclepore filters were evaluated using monodispersed PSL and Ag particles. An atomizer (model ATM-226, Topas, Dresden, Germany) or an electrospray (model 3480, TSI, Shoreview, MN, USA) was used to generate PSL spherical particles ranging in size from 30 to 800 nm. A silicon nitride heater (Kyocera Corp., Kyoto, Japan) was used to generate Ag particles ranging in size from 15 to 30 nm, in a manner similar to that employed by Jung et al. Citation(2006). The aerosolized particles were neutralized by a radioactive source (americium (Am)-241) to establish an equilibrium charge distribution. These were then classified using a differential mobility analyzer (DMA; model 3081 or 3085, TSI) with a platform (model 3080, TSI) to obtain monodispersed particles, and to remove the residuals of ultrapure water used for the PSL dispersions. The charges on the monodispersed particles passing through the DMA were subsequently removed by another Am-241 source. The number concentration of particles upstream of the filter holder was measured using a CPC (model 3775, TSI). The number concentration of particles upstream of the filter holder was of the order of magnitude of 101–104 particles·cm−3, depending on the particle size. The sampling time ranged from a few minutes to a few hours, depending on the concentration and particle size. For each set of conditions, the test was repeated three times.
2.3. Determination of the collection efficiency
Prior to SEM observation, the Nuclepore filters with deposited particles were coated with platinum–palladium (∼2 nm thick) to avoid image charging. Using the field-emission SEM, more than 100 particles (mostly hundreds or thousands of particles) in ∼25 randomly selected fields were counted for each of three regions (central and two surrounding regions; of each filter sample. Representative SEM images of particles collected on the Nuclepore filters are presented in . The PSL particles had a spherical shape, whereas the Ag particles had a rounded shape.
Figure 3. SEM images of (a) 30 nm Ag particles collected on the 0.2 μm pore filter and (b) 30 nm PSL particles collected on the 0.08 μm pore filter.
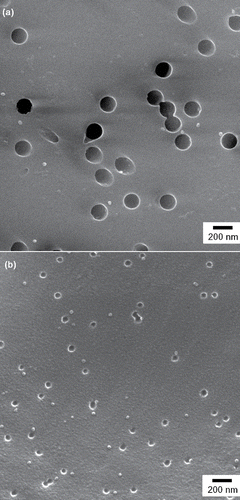
The surface-collection efficiency (ES) of the Nuclepore filter was determined by the ratio of particles on the front surface of the Nuclepore filter visually counted with the SEM to inflow particles counted by the CPC:[1] where Ndep represents the total number of particles deposited on the front surface of the filter and Nin is the total number of particles entering the filter holder. Then, Ndep and Nin were calculated as
[2]
[3] where Afilter is the effective filtration area of the filter holder (=3.7 cm2), NSEM is the total number of particles on the front surface of the Nuclepore filter counted with the SEM in the area of ASEM, ASEM is the total area of the Nuclepore filter observed with the SEM for obtaining the NSEM, V is the total sampling air volume (=flow rate × sample collection time), and C is the average concentration of particles upstream of the filter holder during the sample collection. Because the smaller particles were observed with the SEM at higher magnification, the values of ASEM decreased from ∼400,000 to ∼200 µm2 as the particle size decreased from 800 to 15 nm.
3. Theoretical calculation
The models for calculating the collection efficiencies of Nuclepore filters were developed mostly in 1960–1970 s (Pich Citation1964; Spurny et al. Citation1969; Smith et al. Citation1976; Manton Citation1978, Citation1979). Recently, Chen et al. Citation(2013) and Cyrs et al. Citation(2010) used those models to compare theoretical calculations with the experimental results of both surface and overall Nuclepore collection efficiencies of nano- and submicron sized particles. In addition, R'mili et al. Citation(2013) and Ogura et al. Citation(2014) used those models to calculate the collection efficiencies of holey carbon film-coated TEM grids, which have circular pores as Nuclepore filters do.
Particle collection by a Nuclepore filter is generally described as a set of separate filtration mechanisms: (1) inertial impaction on the filter surface, (2) interception at the pore opening, (3) Brownian diffusion to the front surface of the filter, and (4) Brownian diffusion to the pore wall of the filter (Cyrs et al. Citation2010). The first three mechanisms involve the surface-collection efficiency of Nuclepore filters (Chen et al. Citation2013), and it is often assumed that these three mechanisms act independently. In this case, the overall surface collection efficiency (EOS1) is expressed as[4] where EI, ER, and ED are the collection efficiencies due to impaction, interception, and diffusion to the front surface of the filter, respectively. The EI and ED can be calculated using the popular models proposed by Pich Citation(1964) and Manton Citation(1979), respectively. The details of those equations were summarized in Ogura et al. Citation(2014). For calculating ER, various expressions have been proposed (Spurny et al. Citation1969; Smith et al. Citation1976; John et al. Citation1978; Marre and Palmeri Citation2001). Interception occurs when the center of a particle following a streamline passes within a distance of the particle radius from the rim of the hole. If uniform flow is assumed, ER can be expressed as (Spurny et al. Citation1969)
[5]
[6] where Nr is the particle radius (rp) normalized for the pore radius (r0) (i.e., Nr = rp/r0). If Poiseuille flow is assumed, ER can be expressed as (Smith et al. Citation1976; Manton Citation1978)
[7]
If flow slip is considered in Poiseuille flow, ER can be expressed (Marre and Palmeri Citation2001) as[8]
[9] where Ng is the slip parameter (=lg/r0), lg is the slip length (=1.126λ; Marre et al. Citation2004), and λ is the mean free path of gas molecules (≈0.065 µm for air). If the slip effects are negligible, Equation (Equation8
[8] ) can be expressed as ER = (2NR)2, which is consistent with Equation (Equation7
[7] ). In any of the cases, the equations are valid when Nr < 1. When Nr > 1, the efficiency equals ‘‘1.” presents the collection efficiencies due to interception in terms of Nr for each of the assumptions. The assumption of uniform flow leads to the highest intercept efficiency. Spurny et al. Citation(1969) empirically found that a uniform flow-based ER was overestimated, while Chen et al. Citation(2013) indicated that the experimentally determined -collection efficiencies were in good agreement with the theoretical model calculations, when using a uniform flow-based ER. On the other hand, the assumption of Poiseuille flow without flow slip leads to the lowest intercept efficiency. John et al. Citation(1978) empirically found that an intermediate intercept efficiency expressed as ER = (2NR)1.5 made a good estimation. The assumption of Poiseuille flow with flow slip leads to an intermediate intercept efficiency, depending on the pore size of the filter. While the difference between Poiseuille flow with and without flow slip is almost negligible when the pore size is larger than 1 µm, the effect of flow slip becomes important for filters with smaller pores, as in the case of the 0.08 and 0.2 µm pore filters. Therefore, we used the model proposed by Marre and Palmeri Citation(2001) (i.e., Equations (Equation8
[8] ) and (Equation9
[9] )) to calculate ER.
Manton Citation(1978) pointed out a problem when considering impaction and interception separately. The former process is calculated without accounting for the finite size of a particle, while the latter ignores the inertia of a particle relative to the flow. Manton Citation(1978) therefore calculated the collection efficiency due to the combined mechanisms of impaction and interception on the filter (EIR) from the computed trajectories of particles, and proposed an approximate expression for EIR as a function of an inertia parameter I. The details of the equations with a slight modification (i.e., with consideration for the Cunningham slip correction factor) were summarized in Ogura et al. Citation(2014).
If EIR and ED are independent of each other, the overall surface collection efficiency (EOS2) can be expressed as[10]
We calculated the individual collection efficiencies, EI, ER, EIR, and ED, of the 0.08 and 0.2 µm pore filters for the individual test conditions, and then calculated both overall surface-collection efficiencies using Equations (Equation4[4] ) and (Equation10
[10] ). For calculating EI and EIR, particle densities were set as 1 and 10 g·cm−3, corresponding to the true densities of PSL and Ag particles, respectively.
Unfortunately, these conventional theoretical models were not intended for the estimation of the efficiencies of Nuclepore filters with smaller pores, such as the 0.08 and 0.2 µm pore filters. The values of I for the 0.08 and 0.2 µm pore filters were lower than 0.25 in most situations, which exceeds the range of I (0.25 ≤ I ≤ 100) suitable for Manton's Citation(1978) equation for calculating EIR. In addition, the 0.08 µm pore filter has a filter porosity (P) of 0.019, which exceeds the limits of validity for both Manton's Citation(1978) equation for calculating EIR (0.04 ≤ P ≤ 0.36) and Manton's Citation(1979) equation for calculating ED (0.05 ≤ P ≤0.64). Therefore, EIR for the 0.08 and 0.2 µm pore filters and ED for the 08 µm pore filter are not necessarily valid.
4. Results
4.1. Experimental surface collection by region of filter
First, we checked whether there was a difference in the number of particles deposited per unit area (NSEM/ASEM) between the central and surrounding regions of the filter. The experimental surface collection efficiencies (ES) calculated from the values of NSEM/ASEM for each of the central and surrounding regions are summarized in . The results indicate that there were no obvious trends in the difference in ES between the central and surrounding regions. Therefore, the means of ES for all the regions of the filters (i.e., with no distinction between the central and the surrounding regions) for the individual test conditions are used hereafter.
Table 3. Results of experimentally measured surface collection efficiencyaFootnotea.
4.2. Theoretical surface collection efficiencies
The calculated theoretical surface-collection efficiencies are presented in as lines. Diffusion (ED) was the prevailing mechanism for the collection of smaller particles, whereas interception (ER) was the prevailing mechanism for the collection of larger particles in most cases. Impaction (EI) was as significant as ER only for particles with high densities at high face velocities (). The effect of the combined mechanisms of impaction and interception (EIR) was less than that of interception (ER) (i.e., EOS1 > EOS2).
Figure 5. Surface-collection efficiency of the 0.08 μm pore filter at a face velocity of 1.9 cm·s−1 (n = 9; shown as mean ± standard deviation).
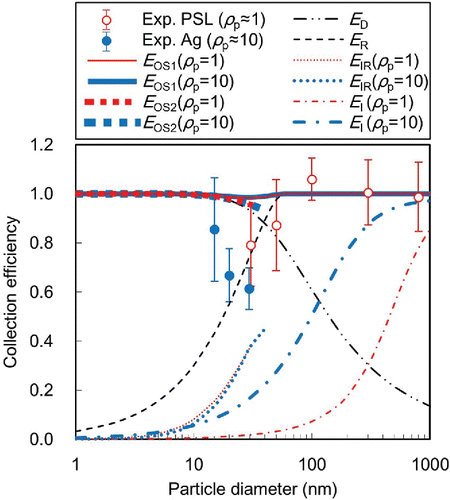
Figure 6. Surface-collection efficiency of the 0.08 μm pore filter at a face velocity of 8.4 cm·s−1 (n = 9; shown as mean ± standard deviation).
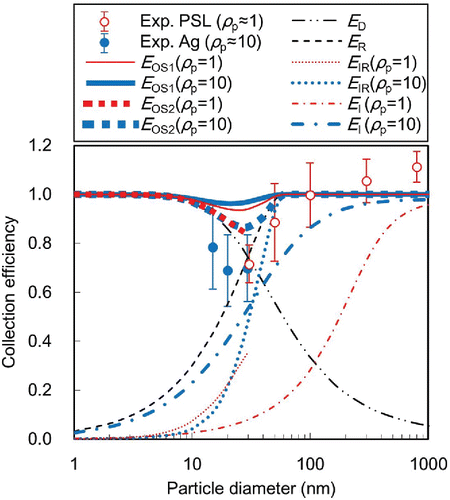
4.3. Comparison between experimental and theoretical surface-collection efficiencies
The means and standard deviations of the experimental surface-collection efficiency for the individual test conditions are presented in as symbols (circles) and error bars, respectively. There were discrepancies between the experimental and theoretical surface collection efficiencies. The experimental surface-collection efficiencies for the 0.2 µm pore filter were comparable to or lower than the theoretical efficiencies EOS1 and EOS2 (). In contrast, the experimental surface-collection efficiencies for the 0.08 µm pore filter were lower than both EOS1 and EOS2 ().
4.4. Surface collection efficiency by face velocity
For the 0.2 µm pore filter, the experimental surface collection efficiencies at a face velocity of 1.5 cm·s−1 () were higher than those at a face velocity of 8.6 cm·s−1 (). This trend was consistent with the theoretical estimation, in which ED decreased as the face velocity increased and ER was independent of face velocity. Although EI increased with face velocity, the contribution of EI to the overall efficiency was limited. For the 0.08 µm pore filter, the difference in the experimental surface-collection efficiency by face velocity was small ().
4.5. Surface collection efficiency by particle density
For the 0.2 µm pore filter at face velocities of 1.5 and 8.6 cm·s−1 (), and for the 0.08 µm pore filter at a face velocity of 8.4 cm·s−1 (), there were small differences in the experimental surface-collection efficiencies between 30 nm PSL and Ag particles. This trend was consistent with theoretical estimation, in which both ED and ER were independent of particle density. Although EI increased as particle density increased, the contribution of EI to the overall efficiency was limited. In contrast, for the 0.08 µm pore filter at a face velocity of 1.9 cm·s−1 (), the experimental surface-collection efficiency for the 30 nm Ag particle was lower than that for the 30 nm PSL particle. This trend was inconsistent with the theoretical estimations of ED, ER, and EI.
4.6. Minimum surface-collection efficiency
The particle sizes having the minimum surface-collection efficiency were theoretically estimated to be 30–100 nm, which corresponds to the transition region between the prevailing mechanisms for the collections of smaller particles (ED) and larger particles (ER, EI, EIR). The experimental results show that the minimum surface-collection efficiencies occurred for particles of approximately 30 nm diameter, which was very near to the theoretical estimate. Those of the 0.08 µm pores at face velocities of 1.9 and 8.4 cm·s−1 were approximately 0.6 and 0.7, respectively, and those of the 0.2 µm pores at face velocities of 1.5 and 8.6 cm·s−1 were approximately 0.8 and 0.6, respectively.
5. Discussion
5.1. Errors and uncertainty in experimental collection efficiencies
The standard deviations in (also the error bars in ) indicate uncertainties in experimental measurements. The deviations of the experimental surface-collection efficiency mainly derived from those related to Ndep because the measurements of Ndep were based on SEM counting of particles deposited on limited portions of filters (). Although the observation area of the Nuclepore filter (ASEM) decreased from ∼400,000 to ∼200 µm2 as the particle size decreased from 800 to 15 nm, there were only small differences in the standard deviations () due to difference in the particle sizes.
In addition to the random errors expressed by the standard deviations, there might be systematic errors. In fact, although the collection efficiencies for PSL particles larger than the pore of the filter should be ‘‘1,” their means were between 0.99 and 1.11 for the individual test conditions. This indicates the tendency to overestimate the collection efficiencies by about 10% or less.
Thus, there would be errors and uncertainty in the experimental collection efficiencies. However, they do not seem to be able to explain the differences between the experimental and theoretical efficiencies well enough.
5.2. Errors and uncertainty in theoretical collection efficiencies
Possible sources of errors and uncertainty in the theoretical collection efficiencies are as follows.
1. | Exceeding the limits of validity for the theoretical models As previously mentioned, the values of I for the 0.08 and 0.2 µm pore filters are relatively low and exceeded the valid range of I for Manton's Citation(1978) equation for calculating EIR in most situations. Chen et al. Citation(2013) indicated that Manton's Citation(1978) equation produced better estimations when the value of I was high. In addition, the porosity P of the 0.08 µm pore filter exceeds the limits of validity for both equations for calculating EIR and ED. Therefore, the calculated values of EIR for the 0.08 and 0.2 µm pore filters, and ED for the 0.08 µm pore filter, are not necessarily valid and may include some error. | ||||
2. | False assumption that each filtration mechanism acts independently The overall surface collection efficiency EOS1 is obtained under the assumption that the three filtration mechanisms (EI, ER, and ED) act independently. Similarly, the overall surface-collection efficiency EOS2 is obtained under the assumption that EIR and ED are independent of each other. However, since the minimum surface-collection efficiencies of the 0.08 and 0.2 µm pore filters are relatively high, the collection of particles with a size near the transition region could be affected by multiple filtration mechanisms. In this case, the overall surface collection efficiencies could be overestimated. In addition, as Manton Citation(1978) pointed out, the inertia of a particle relative to the flow affects the efficiency of interception. As shown in , while a particle with a high Stokes number (Stk) collides with the surface of a filter, a particle with a moderate Stk does not collide but tends to pass through farther from the edge of a pore by an inertia effect. Hence, the collection efficiency of a particle with a moderate Stk (e.g., Stk = 0.05 in conditions of P = 0.04) was estimated to be lower than that of a particle with a low Stk (≈0), which passes through with the flow (Manton Citation1978). Thus, the moderate inertia effect could decrease the efficiency of interception, suggesting that the values of ER(hence also EOS1) might be overestimated. Similarly, a moderate inertia effect could decrease the efficiency of diffusion as well. Therefore, the values of ED for particles might also be overestimated. Actually, the moderate inertia effect may explain the difference in the experimental surface collection efficiencies between 30 nm PSL and Ag particles shown in . The value of Stk for the 30 nm Ag particle under the test conditions in seemed to be moderate (Stk = 0.02; from Equation (18) of Ogura et al. Citation2014), while the value of Stk for the 30 nm PSL particle seemed to be low (Stk = 0.002). For this reason, it is considered that the 30 nm Ag particle was affected by the moderate inertia effect while the 30 nm PSL particle was almost unaffected. This may have resulted in lower collection efficiency of the 30 nm Ag particle. | ||||
3. | Underestimation of EIR due to the assumption of non-slip Poiseuille flow |
Manton's equation to calculate EIR is based on the assumption of non-slip Poiseuille flow inside a pore (Manton Citation1978). However, as shown in , the assumption of non-slip Poiseuille can lead to an underestimation of intercept efficiency for filters with smaller pores, such as the 0.08 and 0.2 µm pore filters. Therefore, EIR (hence also EOS2) possibly leads to underestimation of collection efficiency. This could partly explain the discrepancy between the experimental surface collection efficiency and EOS2 for the 0.2 µm pore filter ().
Because the conventional theoretical models were not intended for estimation of the efficiencies of Nuclepore filters with smaller pores (e.g., 0.08 and 0.2 µm), they have plenty of room for improvement in the estimation of the collection efficiencies of filters with smaller pores. Among the aspects that might be improved are the validity range of the model, the interaction among filtration mechanisms, and consideration of flow slip. The errors and uncertainty in the theoretical collection efficiencies would greatly contribute to the discrepancies between the experimental and theoretical efficiencies.
5.3. Quantitative evaluation of aerosol particles with SEM
A filter with higher minimum surface-collection efficiency is suitable for quantitative evaluation of aerosol particles with an SEM. The minimum surface-collection efficiencies of the 0.08 and 0.2 µm pore filters were approximately 0.6–0.8 for particles around 30 nm diameter, and were higher than the reported minimum surface-collection efficiencies of the 0.4, 0.8, and 1 µm pore filters, which were approximately 0.2–0.3 (). There was little difference in the minimum surface-collection efficiency between the 0.08 and 0.2 µm pore filters. Because the pressure drop of the 0.2 µm pore filter was lower than that of the 0.08 µm pore filter under the same flow-rate conditions, the 0.2 µm pore filter would be more suitable considering the pressure drop and collection efficiency. Moreover, the 0.2 µm pore filter is less expensive.
As a simple method for quantitative evaluation of aerosol particles, the values of the minimum surface-collection efficiency can be used to evaluate the number concentration of particles smaller than the pore of the filter on a safe side, while the collection efficiencies of “1” can be used to estimate the number concentration of particles larger than the pore of the filter.
6. Conclusions
In this study, tests were conducted to determine the surface-collection efficiencies of 0.08 and 0.2 µm Nuclepore filters using monodispersed PSL and Ag particles. The surface-collection efficiency of a 0.08 µm pore filter was almost constant with respect to changes in face velocity from 1.9 to 8.4 cm·s−1, while the surface-collection efficiency of the 0.2 µm pore filter decreased as face velocity increased from 1.5 to 8.6 cm·s−1. There was little difference in the surface-collection efficiency of 30 nm PSL and Ag particles, except for the 0.08 µm pore filter at a face velocity of 1.9 cm·s−1. In this case, the surface-collection efficiency for the 30 nm Ag particle was lower than that for the 30 nm PSL particle. This was possibly due to the moderate inertia effect of the 30 nm Ag particle. The minimum surface-collection efficiencies occurred for particles around 30 nm diameter. Those of the 0.08 µm pores at face velocities of 1.9 and 8.4 cm·s−1 were approximately 0.6 and 0.7, respectively, and those of the 0.2 µm pores at face velocities of 1.5 and 8.6 cm·s−1 were approximately 0.8 and 0.6, respectively. Because the pressure drop of the 0.2 µm pore filter was lower than that of the 0.08 µm pore filter under the same flow-rate conditions, the 0.2 µm pore filter would be more suitable considering the pressure drop and collection efficiency.
Although the theoretically estimated particle sizes having the minimum surface-collection efficiency were very near to those in the experimental results, the theoretical surface-collection efficiencies were quantitatively inconsistent with the experimental results. Conventional theoretical models were not intended for estimation of efficiencies of Nuclepore filters with smaller pores (e.g., 0.08 and 0.2 µm). Therefore, there is plenty of room for improvement in the models for estimation of the collection efficiencies of filters with smaller pores; including aspects such as the validity range of the model, the interaction among filtration mechanisms, and consideration of flow slip.
Acknowledgments
This article is based on results obtained from a project commissioned by the New Energy and Industrial Technology Development Organization (NEDO).
References
- Brouwer, D. H., van Duuren-Stuurman, B., Berges, M., Jankowska, E., Bard, D., and Mark, D. (2009). From Workplace Air Monitoring Results Towards Estimates of Exposure: Development of a Strategy to Assess Exposure to Manufactured Nano-Objects. J. Nanopart. Res., 11:1867–856.
- Chen, S. C., Wang, J., Fissan, H., and Pui, D. Y. H. (2013). Use of Nuclepore Filters for Ambient and Workplace Nanoparticle Exposure Assessment–Spherical Particles. Atmos. Environ., 77:385–393.
- Cyrs, W. D., Boysen, D. A., Casuccio, G., Lersch, T., and Peters, T. M. (2010). Nanoparticle Collection Efficiency of Capillary Pore Membrane Filters. J. Aerosol Sci., 41:655–664.
- Gentry, J. W., Spurny, K. R., and Schoermann, J. (1982). Diffusional Deposition of Ultrafine Aerosols on Nuclepore Filters. Atmos. Environ., 16:25–40.
- John, W., Hering, S., Reischl, G., Sasaki, G., and Goren, S. (1983). Characteristics of Nuclepore Filters with Large Pore Size–II. Filtration Properties. Atmos. Environ., 17:373–382.
- John, W., Reischl, G., Goren, S., and Plotkin, D. (1978). Anomalous Filtration of Solid Particles by Nuclepore Filters. Atmos. Environ., 12:1555–1557.
- Jung, J. H., Oh, H. C., Noh, H. S., Ji, J. H., and Kim, S. S. (2006). Metal Nanoparticle Generation Using a Small Ceramic Heater with a Local Heating Area. J. Aerosol Sci., 37:1662–1670.
- Kuhlbusch, T. A., Asbach, C., Fissan, H., Göhler, D., and Stintz, M. (2011). Nanoparticle Exposure at Nanotechnology Workplaces: A Review. Part. Fibre Toxicol., 8:22.
- Liu, B. Y. H., and Lee, K. W. (1976). Efficiency of Membrane and Nuclepore Filters for Submicrometer Aerosols. Environ. Sci. Technol., 10:345–350.
- Manton, M. J. (1978). The Impaction of Aerosols on a Nuclepore Filter. Atmos. Environ., 12:1669–1675.
- Manton, M. J. (1979). Brownian Diffusion of Aerosols to the Face of a Nuclepore Filter. Atmos. Environ., 13:525–531.
- Marre, S., and Palmeri, J. (2001). Theoretical Study of Aerosol Filtration by Nucleopore Filters: The Intermediate Crossover Regime of Brownian Diffusion and Direct Interception. J. Colloid Interf. Sci., 237:230–238.
- Marre, S., Palmeri, J., Larbot, A., and Bertrand, M. (2004). Modeling of Submicrometer Aerosol Penetration through Sintered Granular Membrane Filters. J. Colloid Interf. Sci., 274:167–182.
- Methner, M., Beaucham, C., Crawford, C., Hodson, L., and Geraci, C. (2012). Field Application of the Nanoparticle Emission Assessment Technique (NEAT): Task-Based Air Monitoring During the Processing of Engineered Nanomaterials (ENM) at Four Facilities. J. Occup. Environ. Hyg., 9:543–555.
- Methner, M., Hodson, L., Dames, A., and Geraci, C. (2010). Nanoparticle Emission Assessment Technique (NEAT) for the Identification and Measurement of Potential Inhalation Exposure to Engineered Nanomaterials–Part B: Results from 12 Field Studies. J. Occup. Environ. Hyg., 7:163–176.
- Montassier, M., Dupin, L., and Bouland, D. (1996). Experimental Study on the Collection Efficiency of Membrane Filters. J. Aerosol Sci., 27(Suppl. 1):S637–S638.
- Ogura, I., Hashimoto, N., Kotake, M., Sakurai, H., Kishimoto, A., and Honda, K. (2014). Aerosol Particle Collection Efficiency of Holey Carbon Film-Coated TEM Grids. Aerosol Sci. Technol. 48:758–767.
- Pich, J. (1964). Impaction of Aerosol Particles in the Neighbourhood of a Circular Hole. Collect. Czech. Chem. Commun., 29:2223–2227.
- R'mili, B., Le-Bihan, O. L. C., Dutouquet, C., Aguerre-Charriol, O., and Frejafon, E. (2013). Particle Sampling by TEM Grid Filtration. Aerosol Sci. Technol., 47:767–775.
- Smith, T. N., Phillips, C. R., and Melo, O. T. (1976). Diffusive Collection of Aerosol Particles on Nuclepore Membrane Filter. Environ. Sci. Technol., 10:274–277.
- Spurny, K. R., Lodge, J. P., Frank, E. R., and Sheesley, D. C. (1969). Aerosol Filtration by Means of Nuclepore Filters: Structural and Filtration Properties. Environ. Sci. Technol., 3:453–464.