ABSTRACT
Gas and particle emissions from R/V Robert Gordon Sproul were measured for ultra low sulfur diesel (ULSD) and hydrogenation derived renewable diesel (HDRD) during dedicated aerosol measurement cruises in 2014 (29 September–3 October) and 2015 (4–7 and 26–28 September). CO, CO2, and NOX were measured directly from the starboard stack from the 2-stroke, small bore, high speed engine, while number and mass size distributions for both particles and black carbon (BC) were measured by intercepting the ship plume. Measurements at constant engine speeds (1600 rpm, 1300 rpm, 1000 rpm, and 700 rpm) had emission factors of CO () and NOX
that were lower by 20% and 13%, respectively, for HDRD compared to ULSD at 700 rpm. However, at 1600 rpm,
and
were within one standard deviation for both ULSD (
: 4.0 ± 0.1 g [kg-fuel]−1;
: 51 ± 0.8 g [kg-fuel]−1) and HDRD (
: 3.9 ± 0.2 g [kg-fuel]−1;
: 51 ± 2 g [kg-fuel]−1). HDRD emission factors of particle number and mass concentrations were higher than ULSD by 46% to 107% and 36% to 150%, respectively, at 1600, 1300, and 1000 rpm, but the differences were smaller than the cycle-to-cycle variability at 700 rpm. BC mass emission factors were nearly 200% larger for 700, 1000, and 1300 rpm for HDRD compared to ULSD, but the mass differences were smaller than cycle-to-cycle variability at 1600 rpm. BC mass size distributions showed that the peak diameter of the BC mass mode for ULSD (∼120 nm) is about 20 nm larger than for HDRD (∼100 nm), even though the particle mass and number size distributions are quite similar.
Copyright © 2017 American Association for Aerosol Research
EDITOR:
1. Introduction
Gases and particles emitted in the engine exhaust of ocean-going vessels have been shown to have adverse impacts on air quality, climate, and public health, particularly near ports and coastal regions (Corbett et al. Citation2007; Vutukuru and Dabdub Citation2008; Fuglestvedt et al. Citation2009; Song Citation2014). Shipping emissions contributed nearly 15% of NOx and 13% of SO2 emissions globally (IMO Citation2014), and shipping-related particle emissions were responsible for nearly 60,000 deaths annually (Corbett et al. Citation2007). With accelerating global trade and continued reduction of on-road vehicle emissions, there could be a significant increase in the relative contribution of shipping emissions to total anthropogenic emissions worldwide. The Third International Maritime Organization (IMO) Greenhouse Gas (GHG) study forecasted that by 2050 CO2 emissions from international shipping could grow by between 50% and 250% depending on future economic and energy growth (IMO Citation2014).
To meet increasingly stringent regulatory standards and reduce dependence on fossil fuels (IMO Citation2016), renewable fuels such as biodiesel could be a viable alternative as fuel for ships. Emissions from on- and off-road diesel engines operating on biodiesel have been investigated for more than two decades, and recent studies have included testing on ships and in other marine diesel engines (Lapuerta et al. Citation2007; Jayaram et al. Citation2011; Petzold et al. Citation2011; Khan et al. Citation2012a,b; Gysel et al. Citation2014). These studies found that substitution of fossil fuels with biodiesel generally reduced soot and particle emissions but increased NOx emissions. As opposed to diesel fuels, biodiesels have largely been produced by transesterification of vegetable or waste oils resulting in a fuel that has high density, low heating value and poor cold flow properties (Hoekman et al. Citation2012). The low oxidation stability of biodiesel also limits its storage capability.
As a result of operational problems with these ester-based biodiesel fuels, hydrogenation-derived renewable diesel (HDRD) has been developed. HDRD is produced from vegetable oil and waste fats by a hydro-treating process similar to that used in oil and gas processing. Limited information is available on the influence of HDRD on diesel engine emissions (Aatola et al. Citation2008; Heikkila et al. Citation2012; Westphal et al. Citation2013; Kim et al. Citation2014; Prokopwicz et al. Citation2015; Bugraski et al. Citation2016), with no specific studies on marine applications of diesel engines. HDRD showed improved engine performance and reduction in NOx emissions compared to biodiesels from transesterification (Aatola et al. Citation2008; Westphal et al. Citation2013; Kim et al. Citation2014).
To study the impacts of HDRD on emissions from an in-use marine diesel engine, exhaust emissions from R/V Robert Gordon Sproul were measured while operating alternately on ULSD and HDRD. NOx, CO, and CO2 were measured from the starboard engine stack. Particle number and black carbon size distributions were measured by intercepting the emitted plume downwind of the stacks for test cycles consisting of 1 h each at four constant engine speeds. These measurements were conducted at-sea in a variety of conditions on two different dedicated research cruises (in 2014 and 2015) in the northeastern Pacific Ocean near the Channel Islands just northwest of San Diego. In addition, CO, CO2, and NOx emissions were monitored during the scheduled research cruises of R/V Sproul in 2015 to study the variability of stack emissions in a wider variety of conditions and operations. In this article, we compare the emission factors of CO and NOx, total particle number, mass, size distributions, and black carbon for HDRD and ULSD while operating at constant engine speeds.
2. Experimental methods
2.1. Vessel and engine specifications
Built in 1981, the R/V Robert Gordon Sproul is a 355-ton, fixed-pitch, twin propeller oceanographic research vessel. It has two main propulsion engines and two auxiliary generators. The main engine, serial number 12EO6361, is a 2-stroke, 12-cylinder Detroit Diesel 12V-149 engine with 5.75 in. bore and 5.75 in. stroke. It has a continuous rating of 675 BHP (504 kW) at 1800 RPM and operates with a compression ratio of 17 to 1.
2.2. Fuels
Both ULSD and HDRD were used as fuels in the existing main and auxiliary diesel engines, which did not have dynamometers to measure engine load. Properties of the fuels used in this study and those of biodiesel produced through transesterification are shown in . HDRD used in this study was purchased from Neste Oil Corporation and was analyzed for C, H, N, and FAME content by Southwest Research Institute (San Antonio, TX, USA).
Table 1. Properties of HDRD and ULSD in this study as well as other biodiesels.
In 2014, ULSD was stored in the main fuel tanks and HDRD was loaded in the smaller day tanks. After the 2014 cruise, R/V Sproul transitioned to using 100% HDRD, and HDRD was carried in the main tanks. For all 2015 cruises, HDRD was carried in the main tanks, and ULSD was loaded in the day tank for the first part of the 2015 dedicated research cruise. Records of engine temperature showed less than 2°C differences between all of the cruise days in 2014 and 2015.
2.3. Sampling
Gas and particle emissions from the R/V Sproul were measured using two sets of real-time instruments (Table S1), with gas analyzers sampling directly from the stack and particle instruments intercepting the “fresh” plume, directly after emission into the atmosphere. The first set of detailed emission measurements was carried out from 29 September to 3 October 2014 (2014 cruise). The second set was carried out in two parts: from 4 to 7 September and from 26 to 28 September 2015 (2015 cruise).
2.3.1. Measurements of stack gases
CO, CO2, and NOx emissions were measured from the stack using a custom-designed probe, heated inlet, and water-removal system (GK Associates, Simi Valley, CA, USA; Universal Analyzers, Carson City, NV, USA). The engine exhaust was sampled through a probe inserted into the main stack vent of the starboard engine (). Particles were removed from the exhaust after the probe at the upstream end of the heated sampling line by a Teflon filter element with 2 μm nominal cut size (Universal Analyzers). The insulated line was 5 m long embedded with 1/2″ OD Teflon tube and heating elements controlled to 150°C. The cooling unit chilled the line to ca. 20°C, removing condensed water to a trap and filtering any remaining particles. The flow was set to 4 L min−1, providing 850 cm3 min−1 to the CO Analyzer (Model: T300 with CO2 module, Teledyne API, San Diego, CA, USA) and 250 cm3 min−1 (diluted by a measured ratio of zero air) to the NOx analyzer (Model: T200M, Teledyne API), with the excess vented.
2.3.2. Particle measurements in aerosol sampling van
During the dedicated 2014 and 2015 sampling cruises, cooled and diluted particle emissions from the R/V Sproul were measured using the plume intercept method with the inlet of the aerosol sampling van. Particles were measured in the plume approximately 20 m downwind of the engine stacks (as shown in ) by heading directly into the wind. Atmospherically relevant dilution factors and temperatures were achieved by the time the plume was sampled. An isokinetic inlet with a motorized horizontal shroud mounted on the sampling van was used to sample the plume (; Bates et al. Citation1998). Air pulled through the inlet was then distributed through a sampling plenum to the particle instruments housed in the van.
Table S2 lists the instruments used in this study and the particle properties measured by each. Size distributions of particles in the plume were measured over a large size range (0.01–10 μm) using three particle-sizing instruments. Sub-micrometer particles (0.01–1 μm) were measured with a Scanning Electrical Mobility Spectrometer (SEMS, Model 138 2002, Brechtel Manufacturing Inc., Hayward, CA, USA). Larger particles were measured using an Aerodynamic Particle Sizer (APS, Model 3321, TSI Inc., Shoreview, MN; size range 0.5–20 μm) and an Optical Particle Sizer (OPS, Model 330, TSI Inc., Shoreview, MN; size range 0.3–10 μm). The Single Particle Soot Photometer (SP2, Model SP2-C, DMT, Boulder, CO, USA) was used to measure refractory black carbon (BC, or soot) mass and number concentrations, as well as size distributions in the 0.07 to 0.4 μm range. CO2 concentrations in the plume were measured using an infrared CO2 analyzer (Model LI-840, LI-COR, Lincoln, NE, USA). Particle chemical composition was measured by High Resolution Time of Flight Aerosol Mass spectrometer (Model: HR-ToF-AMS with ET, Aerodyne, Billerica, MA, USA) and by collecting 37 mm Teflon filters (Pall Corporation, San Diego, CA, USA) for Fourier Transform Infrared (FTIR) spectroscopy, as described by Price et al. (Citation2017). Filters were collected to measure characteristics related to particle toxicity, including trace metal content and ability of the particles to produce hydroxyl radicals (Kuang et al. Citation2017).
2.4. Calculation of emission factors
Emission factors (EFs) describe the gas or particle quantities emitted per kg-fuel consumed; the fuel consumed was calculated by using the carbon in the CO2 measured in the stack or sampling van to calculate the amount of fuel burned (using the fuel carbon fraction in ). For CO and NOx, which were measured from the engine stack, EFs were estimated using Equation (Equation1[1] ),
[1] where i is the gas (CO or NOx); C(i) is its mixing ratio in ppm; and M(i) is the molecular weight of gas i.
is the emission factor of CO2 in g (kg-fuel)−1.
for HDRD and ULSD are estimated to be ∼3100 g (kg-fuel)−1 for both, based on C content in , assuming all the carbon in the fuel is converted to CO2 during combustion.
To estimate EFs for measurements in the aerosol sampling van, the measurements were synchronized to the LI-COR CO2, which has 1 s resolution. The time difference between instruments included a small difference in plumbing time and a calibrated difference in computer clock time. The delay times were calibrated by minimizing variability for SEMS and by lagged correlation for SP2. For the 5 min size scans of SEMS, the delay for each 5 s particle size bin and the 5 s average CO2 was used to match the CO2 concentration to the measured particle concentration for each bin. For the 1 s size scans of SP2, the delay between each 1 s BC size scan and the 1 s CO2 was used to align the CO2 and each size scan.
After measurements were synchronized, background concentrations were subtracted from measured particle and CO2 concentrations. EFs were estimated using Equation (Equation2[2] )
[2] where p and pbgd are particle concentrations (total or black carbon) in the ship plume and background, respectively; CO2 and CO2,bgd (background CO2 concentrations) are in ppm; f is the conversion factor (1.8×10−9) to convert CO2 from ppm to g cm−3. Average and standard deviations of SEMS size scans were normalized to CO2 separately for each size bin for which the CO2 exceeded background by 10 ppm, then all bins meeting these criteria were aggregated. This approach enabled inclusion of the parts of size scans that were in plume even if the entire scan was not in the plume, as well as normalizing to the simultaneous CO2. The fast size scans (1 s) of SP2 meant that those measurements were averaged based on entire scans rather than bin-by-bin. Mean wind speeds during different engine cycles are shown in Table S5 for reference.
3. Results and discussion
This section summarizes the CO, NOx, particle, and black carbon emissions measured during the dedicated 2014 and 2015 research cruises. Gas, particle, and black carbon number concentrations measured during the 2014 and 2015 aerosol sampling cruises are shown in . These time series show the sharp differences in concentration that were sampled at each engine speed during the engine cycle tests for HDRD and ULSD. Data used in this study are available at UC San Diego Library Digital Collections (Russell et al. Citation2016).
Figure 2. (a) CO, CO2, and NOx gas concentrations measured for the 2015 cruise, measured at the starboard stack. (b) Particle and black carbon number concentrations and LI-COR CO2 concentrations in the ship plume during the 2014 and 2015 cruises, measured in the aerosol sampling van.
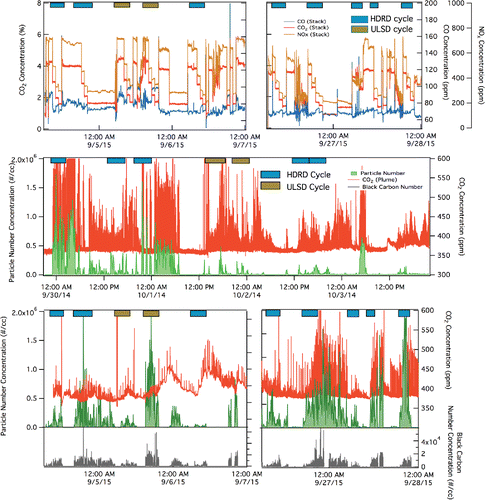
3.1. CO and NOx emission factors
Emission factors of CO () and NOx (
) ( and Table S4) were calculated from the continuous stack measurements of CO2, CO, and NOx concentrations. The starboard and port engines were operated at the same speed, with engine speed held constant at four fixed values: 1600 rpm, 1300 rpm, 1000 rpm, and 700 rpm. At engine speeds of 700 rpm to 1600 rpm,
varied between 3.9 and 9.3 g (kg-fuel)−1 for HDRD and between 4 and 11.7 g (kg-fuel)−1 for ULSD. For the same range of engine speeds,
varied between 47 and 55 g (kg-fuel)−1 for HDRD and between 51 and 64 g (kg-fuel)−1 for ULSD.
Figure 3. Emission factors (EF) of (a) NOx, (b) CO, (c) particle mass, (d) black carbon mass, (e) particle number, and (f) black carbon number for the engine emissions of the R/V Robert Gordon Sproul at different engine speeds for ULSD and HDRD. Each mean (shown as thick bar) represents the average of 1 h cycles with individual cycles shown as narrow bars. The data included in each cycle were selected based on the criteria in . The number of cycles that met these criteria for CO and NOx were seven for HDRD and two for ULSD at all engine speeds. For particle number and mass, the number of cycles that met these criteria were 5, 6, 7, and 8 for HDRD and 2, 3, 4, and 8 for ULSD at 1600, 1300, 1000, and 700 rpm, respectively. For black carbon number and mass, the number of cycles that met these criteria were six for HDRD and two for ULSD at all engine speeds. The cycles included measurements during the 2014 cruise (9/29/14–10/3/14) and the 2015 cruise (9/4/15–9/6/15 and 9/26/15–9/28/15). CO, NOx, and BC measurements are not available for 2014 cruise. Table S3 provides the fraction of in-plume measurements for each engine cycle included in this analysis.
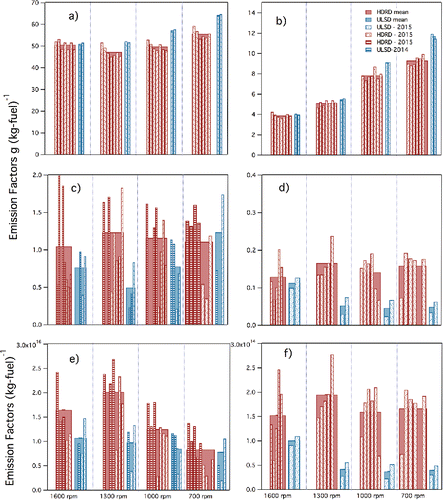
In addition, stack gas emissions were measured on 24 other cruises scheduled for oceanographic research studies on R/V Sproul during 2015. EFs for HDRD on these cruises (Figure S1, see the online supplementary information [SI]) are in the range of 1.26–13.1 g (kg-fuel)−1 for and 29–142 g (kg-fuel)−1 for
. While these emissions were comparable to the values measured during the dedicated sampling cruises, they showed a greater variability over a wider range of operating and sea conditions.
During the controlled engine speed cycle tests on the 2014 and 2015 cruises, both mean and
decreased consistently with increasing engine speed for ULSD and HDRD from 700 to 1000 to 1300 rpm, although the differences at 1300 and 1600 rpm are smaller than twice the standard deviation of the cycles. From 1300 to 1600 rpm, there is a small increase in mean
(smaller than twice the standard deviation of the cycles at each speed), but
continues to decrease for both HDRD and ULSD. The difference in emission factors with engine speed was larger for
than for
, with
decreasing by nearly a factor of 3 but with
decreasing by only 10% for HDRD and 25% for ULSD when engine speed increased from 700 rpm to 1600 rpm. Cappa et al. (Citation2014) observed a threefold increase in
with decreasing vessel speed, similar to this study, for emissions from a research vessel that was also equipped with a 2-stroke, diesel engine powered by low sulfur diesel, although that engine was rated for medium rather than high speed.
For the R/V Sproul, and
were both lower for HDRD compared to ULSD.
was lower for HDRD compared to ULSD by 14% and 20% at 1000 rpm and 700 rpm, respectively, whereas
was lower for HDRD compared to ULSD by 13% at both 1000 rpm and 700 rpm. However, at 1300 and 1600 rpm, the differences in mean
and
between HDRD and ULSD were smaller than the standard deviations of the cycles.
The HDRD used in this study contains straight chain paraffinic hydrocarbons that are more similar to petroleum-based diesel fuels than first generation FAME-type biofuels, rating a higher cetane number (75.2) than most biofuels and having very low aromatic and oxygen content (). Given these differences between HDRD and non-hydrogenated biodiesels, it is not surprising that diesel engine studies (using a variety of 2-stroke and 4-stroke engine types) have reported a decrease in CO emissions and both increases and decreases in NOx emissions for non-hydrogenated biodiesels compared to diesel (Lapuerta et al. Citation2007; Betha and Balasubramanian Citation2011; Khan et al. Citation2012b; Gysel et al. Citation2014). The higher cetane number of HDRD used in this study compared to ULSD suggests that HDRD has smaller pressure and temperature gradients, and thereby lower NOx formation. While there are no published reports of EFs for HDRD in marine diesel engines that are directly comparable to the engine on the R/V Sproul, several studies have shown a reduction in CO and NOx emissions for HDRD compared to conventional diesel in other types of diesel engines (Westphal et al. Citation2013; Kim et al. Citation2014; Prokopowicz et al. Citation2015).
3.2. Particle number and mass size distributions
In addition to measurements of stack gases, particle number size distributions were measured in the plume directly after dilution and cooling in the atmosphere about 20 m downwind from the stack. The selection criteria used to identify times when the plume was being sampled are shown in Table S2 (see the SI). Since particle density for the plume was not measured, EFM is estimated from number particle size distributions assuming a density of 1 g cm−3. Particles larger than 400 nm were associated primarily with other (non-ship) particle sources and are not shown here, since their limited variability reflected differences in the background atmospheric aerosol concentrations rather than in particles from ship emissions.
Number and mass emission factors for the R/V Sproul indicate that particle emissions were higher when running on HDRD than on ULSD ( and Table S4). Mean submicron particle number emission factors (EFN) varied from 0.9 to 2.1×1016 (kg-fuel)−1 (with standard deviations between 0.4 and 0.6 × 1016 (kg-fuel)−1) for HDRD and from 0.6 to 1.1 × 1016 (kg-fuel)−1 (with standard deviations between 0.4 and 0.5 × 1016 (kg-fuel)−1) for ULSD for the four measured engine speeds. Mean particle mass emission factors (EFM) varied from 1.0 to 1.2 g (kg-fuel)−1 for HDRD and from 0.5 to 1.2 g (kg-fuel)−1 for ULSD. At all engine speeds, EFN was higher for HDRD than for ULSD, but the differences were smaller than two standard deviations. EFM was also higher for HDRD than for ULSD at all speeds except 700 rpm, but the differences were smaller than two standard deviations of the cycles at every speed. The particle emissions observed in this study were lower when compared to global average EFM of 4.2 to 7.3 g (kg-fuel)−1 from a compilation of ocean-going vessels for both heavy fuel oil (HFO) and low sulfur fuel oil (IMO Citation2014).
The mean EFN and EFM for HDRD were higher than those for ULSD, especially at higher speeds. The differences were larger for number than mass emission factors, but both are less than two standard deviations of the results for the cycles, meaning that further studies would be needed to obtain statistically significant results. However, the more similar mass emission factors are consistent with the similar straight chain paraffinic hydrocarbon composition of both ULSD and HDRD since the similar composition would produce a similar flame front and hence a similar particle mass fraction. The slightly larger differences in particle number emission factors may result from the higher cetane number of the HDRD compared to ULSD (75.2 for HDRD compared to 41–50 for ULSD), since the higher cetane numbers have shorter ignition delays that result in less time for premixing and more smaller particles.
shows that the particle emission factors reported here for a high-speed, 2-stroke engine are within the range of values reported for slow-speed and medium-speed 2-stroke engines operating on a range of fuels (heavy fuel oil [HFO], low sulfur fuel [LSF], and marine gas oil [MGO]). Even though a direct comparison is not appropriate, EFN for both the ULSD and HDRD are within a factor of 2 of those for the HFO and LSF. Decreased EFM for conventional biodiesels in a variety of 4-stroke engines (Petzold et al. Citation2011; Jayaram et al. Citation2011; Khan et al. Citation2012b; Gysel et al. Citation2014) has been attributed to the higher oxygen content (<0.16 for HDRD but >11 for other biofuels) and absence of aromatics, neither of which applies to the HDRD used here. On the other hand, several studies reported an increase in EFN for biodiesel compared to ULSD for variety of engine types (Jayaram et al. Citation2011; Lackey and Paulson Citation2012), similar to the results of the engine studied here.
Table 2. Comparison of emission factors reported for different 2-stroke ship engines and fuel types.
The increase in EFN observed in other studies (Jayaram et al. Citation2011; Lackey and Paulson Citation2012) is primarily attributed to the increase in nucleation mode particles (diameter > 50 nm) for biodiesel. In this study, at each of the four measured engine speeds, the emission factor of nucleation mode particles with diameters between 10 and 50 nm was in the range of 0.6–1.8 × 1016 (kg-fuel)−1 (approximately 77–89% of EFN) for HDRD and 0.3–1 × 1016 (kg-fuel)−1 (approximately 67–92% of EFN) for ULSD. The fraction of nucleation mode particles was higher for HDRD compared to ULSD at all engine speeds except 1600 rpm, for which the difference in the emission factor of nucleation mode particles with diameters between 10 and 50 nm was smaller than two standard deviations of the cycles.
Emission factors of particle mass and number distributions are shown in . For HDRD and ULSD, the number size distributions were bimodal with one clear mode below 100 nm and one broad or two more narrow modes above 100 nm. The <100 nm number mode peaked at approximately 40 ± 5 nm for both ULSD and HDRD for all engine speeds. For ULSD, the peak diameter of the <100 nm mass mode generally increased with decreasing engine speed, but for HDRD there was no consistent change with engine speed (). The larger mode was more evident in the mass size distributions with peaks ranging between 100 and 300 nm diameter for HDRD and ULSD. For both HDRD and ULSD, the >100 nm mode with the largest mass has peak diameter below 200 nm at 700 rpm but above 200 nm at 1600 rpm. These trends in the mass size distributions are generally consistent with the observed changes in organic aerosol size distributions measured by HR-ToF-AMS that are reported by Price et al. (Citation2017).
Figure 4. Mass (a, b) and number (c, d) size distributions of particles measured in the plume at 1600 1300, 1000, and 700 rpm for HDRD (a, c) and ULSD (b, d). A five point box smoothing was applied for the size distribution plots. The shaded area indicates the standard error for each cycle at each diameter. The number of cycles available for each engine speed are 5, 6, 7, and 8 for HDRD and 2, 3, 4, and 8 for ULSD at 1600, 1300, 1000, and 700 rpm, respectively. The reported size distributions were measured during the 2014 cruise (9/29/14–10/3/14) and the 2015 cruise (9/4/15–9/6/15 and 9/26/15–9/28/15). Table S3 provides the fraction of in-plume measurements for each engine cycle included in this analysis.
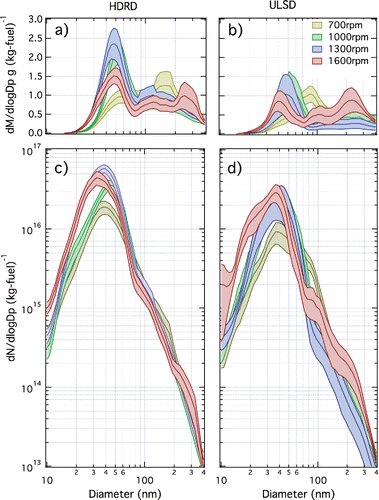
3.3. Black carbon number and mass size distributions
(and Table S4) shows the mean emission factors of refractory black carbon number (EFBCN) and mass (EFBCM) concentrations. The criteria for selecting the measurements from the plume are shown in Table S2. EFBCN and EFBCM were higher for HDRD (1.5 to 1.9 × 1014 (kg-fuel)−1; 0.13 to 0.17 g (kg-fuel)−1) compared to ULSD (0.4 to 1 × 1014 (kg-fuel)−1; 0.05 to 0.1 g (kg-fuel)−1) at all engine speeds, but the difference was within one standard deviation at 1600 rpm. At 700, 1000, and 1300 rpm, EFBCN is more than four times higher and EFBCM is approximately three times higher for HDRD compared to ULSD. However, at 1600 rpm, the difference between HDRD and ULSD for EFBCM and EFBCN were less than two standard deviations. This similarity in emissions at 1600 rpm is consistent with the difference in between HDRD and ULSD at 1600 rpm being within two standard deviations.
BC number and mass size distributions for particles in the size range of 70 to 400 nm are shown in . The peak diameter of the BC mass mode measured by the SP2 for ULSD (∼120 nm) is about 20 nm larger than for HDRD (∼100 nm). The fraction of BC particles below 100 nm for HDRD (80–85%) is slightly larger than for ULSD (∼75%). Nearly 50% of BC mass is contributed by soot particles in the 70–100 nm diameter range for HDRD, while 34% mass is contributed by soot particles in the 70–100 nm size range for ULSD. In general, HDRD emissions produced smaller and more numerous BC particles than ULSD.
Figure 5. Black carbon mass and number size distributions from SP2 during the 2015 cruise (9/4/15–9/6/15 and 9/26/15–9/28/15). A five point box smoothing was applied for the size distribution plots. The shaded area indicates standard error for the time in plume for each cycle at each diameter; the cycles available at all engine speeds were six for HDRD and two for ULSD. Table S3 provides the fraction of in-plume measurements for each engine cycle included in this analysis. This instrument was not available during the 2014 cruise.
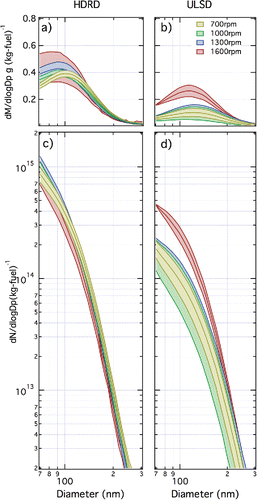
4. Conclusions
The measurements reported here illustrate the differences in gas and particle emissions for HDRD compared to ULSD during at-sea operations of marine diesel engine emissions for the R/V Robert Gordon Sproul. CO and NOx emissions ( and
) were 20% and 13% lower, respectively, for HDRD compared to ULSD at 700 rpm, but were indistinguishable at 1600 rpm (
: 50 ± 2 g (kg-fuel)−1 for HDRD and 50 ± 0.8 g (kg-fuel)−1 for ULSD;
: 3.9 ± 0.2 g (kg-fuel)−1 for HDRD and 4.0 ± 0.1 g (kg-fuel)−1 for ULSD).
EFM was similar for both HDRD and ULSD at 700 rpm (1.1 ± 0.3 g (kg-fuel)−1 for HDRD and 1.2 ± 0.2 g (kg-fuel)−1 for ULSD) but was 140% higher for HDRD than for ULSD at 1600 rpm. EFN was higher for HDRD than for ULSD at all engine speeds. For HDRD and ULSD, the submicron number and mass size distributions had two clear submicron modes, with one below 100 nm (peaking at 40+/−5 nm) and the other above 100 nm in number peak diameter. There were no consistent differences between the sizes of either mode for HDRD and ULSD.
Black carbon mass-based emissions (EFBCM) were 200% higher for HDRD at 700 rpm compared to ULSD but were nearly the same for both fuels at 1600 rpm (0.12 g (kg-fuel)−1 for both ULSD and HDRD). These BC emission factors (EFBCM) for HDRD are higher than for ester-based biodiesel fuels and comparable to HFO and low sulfur fuel (), which is consistent with the fact that BC emissions depend on engine type, age, and operating parameters. HDRD emissions produced smaller BC particles than ULSD at all engine speeds, even though the particle size distributions were quite similar. A larger fraction (80–85%) of BC number was below 100 nm diameter for HDRD compared to ULSD (∼75%).
Many conventional biodiesels produced through trans-esterification have been shown to reduce particle emissions and increase NOx from combustion engines compared to ULSD. However, here HDRD had slightly lower NOx emissions but higher particle number emissions compared to ULSD. The lower density and higher cetane number of HDRD compared to ULSD likely explain the decrease in NOx. The higher particle emissions may result from characteristics specific to the R/V Sproul engine as well as the higher cetane number of the HDRD compared to ULSD.
Measuring particle and BC emissions during at-sea vessel operations provides information on the effects of sea state on emissions at constant engine speed. Additional at-sea sampling along with direct measurements of engine load would be needed to quantify the effects of sea state on particle and BC emissions.
UAST_1238034_Supplemental_File.zip
Download Zip (207.6 KB)Acknowledgments
The authors thank Bruce Applegate, Christopher Welton, Bud Hale, Paul Mauricio, Ernie Bayer, Lee Ellett, Drew Cole, Matt Durham, Josh Manager, Keith Saddle, Meghan Donohue, Xinze Peng, Kylee Chang, Judith Garfield, and others who served aboard the R/V Robert Gordon Sproul for their helpful assistance in the set up and maintenance of instruments during this study.
Funding
The authors appreciate support from the U.S. Department of Transportation, including the guidance of Sujit Ghosh and funding from grant DTMA-91-H-2013-0001.
References
- Aatola, H., Larmi, M., Sarjovaara, T., and Mikkonen, S. (2008). Hydrotreated Vegetable Oil (HVO) as a Renewable Diesel Fuel: Trade-Off between NOx, Particulate Emission, and Fuel Consumption of a Heavy Duty Engine. SAE Paper 2008-01-2500.
- Agrawal, H., Malloy, Q. G. J., Welcj, W. A., Miller, J. W., and Cocker, D. R. (2008). In-Use Gaseous and Particulate Matter Emissions from a Modern Ocean Going Container Vessel. Atmos. Environ., 42:5504–5510.
- Bates, T. S., Huebert, B. J., Gras, J. L., Griffiths, F. B., and Durkee, P. A. (1998). International Global Atmospheric Chemistry (IGAC) Project's First Aerosol Characterization Experiment (ACE 1): Overview. J. Geophys. Res., 103:16297–16318.
- Betha, R., and Balasubramanian, R. (2011). Particulate Emissions from a Stationary Engine Fueled with Ultra-Low-Sulfur Diesel and Waste-Cooking-Oil-Derived Biodiesel. J. Air Waste Manage. Assoc., 61:1063–1069.
- Bugraski, A. D., Hummer, J. A., and Vanderslice, S. (2016). Effects of Hydrotreated Vegetable Oil on Emissions of Aerosols and Gases From Light-Duty and Medium-Duty Older Technology Engines. J. Occup. Environ. Hygiene, 13(4):297–306.
- Cappa, C. D., Williams, E. J., Lack, D. A., Buffaloe, G. M., Coffman, D., Hayden, K. L., Herndon, S. C., Lerner, B. M., Li, S.-M., Massoli, P., McLaren, R., Nuaaman, I., Onasch, T. B., and Quinn, P. K. (2014). A Case Study Into the Measurement of Ship Emissions from Plume Intercepts of the NOAA ship Miller Freeman. Atmos. Chem. Phys., 14:1337–1352.
- Corbett, J. J., Winebrake, J. J., Green, E. H., Kasibhatla, P., Eyring, V., and Lauer, A. (2007). Mortality from Ship Emissions: A Global Assessment. Environ. Sci. Technol., 41(24):8512–8518.
- Fridell, E., and Salo, K. (2016). Measurements of Abatement of Particles and Exhaust Gases in Marine Gas Scrubber. Proc. IMechE. Part M: J. Engineer. Maritime. Environ., 230(1):154–162. DOI: 10.1177/1475090214543716. doi:10.1177/1475090214543716
- Fuglestvedt, J., Berntsen, T., Eyring, V., Isaksen, I., Lee, D. S., and Sausen, R. (2009). Shipping Emissions: From Cooling to Warming of Climate-and Reducing Impacts on Health. Environ. Sci. Technol. 43(24):9057–9062.
- Graboski, M. S., McCormick, R. L., Alleman, T. L., and Herring, A. M. (2003). The Effect of Biodiesel Composition on Engine Emissions from a DDC Series 60 Diesel Engine. Final report 2, National Renewable Energy Laboratory NREL/SR-510-31461. Available at http://www.nrel.gov/docs/fy03osti/31461.pdf
- Gysel, N. R., Russell, R. L., Welch, W. A., Cocker, D. R., and Ghosh, S. (2014). Impact of Sugarcane Renewable Fuel on In-Use Gaseous and Particulate Matter Emissions from a Marine Vessel. Energy Fuels, 28:4177–4182.
- Hansen, K. F., and Jensen, M. G. (1997). Chemical and Biological Characteristics of Exhaust Emissions from a DI Diesel Engine Fuelled with Rapeseed Oil Methyl Ester (RME). SAE Paper 971689.
- Heikkila, J., Happonen, M., Murtonen, T., Lehto, K., Sarjovaara, T., Larmi, M., Keskinen, J., and Virtanen, A. (2012). Study of Miller Timing on Exhaust Emissions of a Hydrotreated Vegetable Oil (HVO)-Fueled Diesel Engine. J. Air Waste Manag. Assoc., 62(11):1305–1312.
- Hoekman, S. K., Broch, A., Robbins, C., Ceniceros, E., and Natarajan, M. (2012). Review of Biodiesel Composition, Properties, and Specifications. Renew. Sustain. Energy Rev., 16:143–169.
- IMO (2014). Third IMO Greenhouse Gas Study, 2014. Available at http://www.imo.org/en/OurWork/Environment/PollutionPrevention/AirPollution/Documents/Third%20Greenhouse%20Gas%20Study/GHG3%20Executive%20Summary%20and%20Report.pdf
- IMO (2016). Sulphur oxides (SOx) – Regulation 14. Available at http://www.imo.org/en/OurWork/Environment/PollutionPrevention/AirPollution/Pages/Sulphur-oxides-%28SOx%29-%E2%80%93-Regulation-14.aspx
- Jayaram, V., Agrawal, H., Welch, W. A., Miller, J. W., and Cocker, D. R. (2011). Real-Time Gaseous, PM and Ultrafine Particle Emissions from a Modern Marine Engine Operating on Biodiesel. Environ. Sci. Technol., 45:2286–2292.
- Khan, M. Y., Giordano, M., Gutierrez, J., Welch, W. A., Asa-Awuku, A., Miller, J. W., and Cocker, D. R. (2012a). Benefits of Two Mitigation Strategies for Container Vessels: Cleaner Engines and Cleaner Fuels, Environ. Sci. Technol., 46:5049–5056.
- Khan, M. Y., Russell, R. L., Welch, W. A., Cocker, D. R., and Ghosh, S. (2012b). Impact of Algae Biofuel on In-Use Gaseous and Particulate Emissions from a Marine Vessel. Energy Fuels, 26:6137–6143.
- Kim, D., Kim, S., Oh., S., and No, S.-Y. (2014). Engine Performance and Emission Characteristics of Hydrotreated Vegetable Oil in Light Duty Diesel Engines. Fuel, 125:36–43.
- Kuang, X. M., Scott, J. A., da Rocha, G. O., Betha, R., Price, D. J., Russell, L. M., Cocker, D. R., and Paulson, S. E. (2017). Hydroxyl Radical Formation and Trace Metal Content in Particulate Matter from Renewable Diesel and Ultra Low Sulfur Diesel in At-Sea Operations of a Research Vessel. Aerosol. Sci. Technol. 51(2):147-158
- Lackey, L., and Paulson, S. E. (2012). The Influence of Feedstock: Air Pollution and Climate Related Emissions from a Diesel Generator Operating on Soybean, Canola, and Yellow Grease Biodiesel. Energy Fuels, 1:686–700.
- Lapuerta, M., Armas, O., and Rodriguez-Fernandez, J. (2007). Effect of Biodiesel Fuels on Diesel Engine Emissions. Progress Energy Combus. Sci., 34:198–223.
- Murphy, S. M., Agrawal, H., Sorooshian, A., Padro, L. T., Gates, H., Hersey, S., Welch, W. A., Jung, H., Miller, J. W., Cocker, D. R., Nenes, A., Jonsson, H. H., Flagan, R. C., and Seinfeld, J. H. (2009). Comprehensive Simultaneous Shipboard and Airborne Characterization of Exhaust from a Modern Container Ship at Sea. Environ. Sci. Technol., 43(13):4626–4640.
- Petzold, A., Lauer, P., Fritsche, U., Hasselbach, J., Lichtenstern, M., Schlager, H., and Fleischer, F. (2011). Operation of Marine Diesel Engines on Biogenic Fuels: Modification of Emissions and Resulting Climate Effects. Environ. Sci. Technol., 45:10394–10400.
- Price, D. J., Chen, C.-L., Russell, L. M., Lamjiri, M. A., Betha, R., Sanchez, K., Liu, J., Lee, A. K. Y., and Cocker, D. R. (2017). More Unsaturated, Cooking-Type Hydrocarbon-Like Organic Aerosol Particle Emissions from Renewable Diesel Compared to Ultra Low Sulfur Diesel in At-Sea Operations of a Research Vessel. Aerosol. Sci. Technol. 51(2):135-146
- Prokopwicz, A., Zaciera, M., Sobczak, A., Bielaczyz, P., and Woodburn, J. (2015). The Effects of Neat Biodiesel and Biodiesel and HVO Blends in Diesel Fuel on Exhaust Emissions from a Light Duty Vehicle with a Diesel Engine. Environ. Sci. Technol., 49:7473–7482.
- Russell, L. M., Betha, R., Sanchez, K. J., Liu, J., Price, D. J., Lamjiri, M. A., Chen, C.-L., Miller, J. W., and Cocker, D. R. (2016). Stack Gas and Plume Aerosol Measurements from Renewable Diesel and Ultra Low Sulfur Diesel in At-Sea Operation of Research Vessel Robert Gordon Sproul. UC San Diego Library Digital Collections. Available at http://doi.org/10.6075/J0V985ZZ.
- Song, S. (2014). Ship Emissions Inventory, Social Cost and Eco-Efficiency in Shanghai Yangshan Port. Atmos. Environ., 82(0):288–297.
- Vutukuru, S., and Dabdub, D. (2008). Modeling the Effects of Ship Emissions on Coastal Air Quality: A Case Study of Southern California. Atmos. Environ., 42(16):3751–3764.
- Westphal, G. A., Krahl, J., Munack, A., Rosenkranz, N., Schroder, O., Schaak, J., Pabst, C., Bruning, T., and Bunger, J. (2013). Combustion of Hydrotreated Vegetable Oil and Jatropha Methyl Ester in a Heavy Duty Engine: Emissions and Bacterial Mutagenicity. Environ. Sci. Technol., 47:6038–6046.
- Winnes, H., Moldanova, J., Anderson, M., and Fridell, E. (2014). On-Board Measurements of Particle Emissions from Marine Engines Using Fuels with Different Sulphur Content. Proc. IMechE., Part M: J. Eng. Maritim. Environ., 230:45–54.