ABSTRACT
An experimental study was carried out to investigate the formation process of airborne nanoparticles from tire tread. The formation of nanoparticles by volatilization of the tire tread was simulated in a reaction chamber. The number concentration of nanoparticles in the reaction chamber suddenly increased when the tire tread surface temperature reached above 160°C. The generated nanoparticles have a unimodal distribution and the number of nanoparticles increased as the heating rate increased. The geometric mean diameter and size distribution of the generated particles can be controlled by adjusting the cooling rate since the cooling rate is directly related to the growth of particles. From the morphological and elemental analyses, the main components of the tire nanoparticles were C, O, S, and Si and the particles had an irregular shape. Based on these observations, we concluded that the formation mechanism of nanoparticles from the tire tread was volatilization and condensation of the organic materials in the tire tread.
Copyright © 2017 American Association for Aerosol Research
EDITOR:
Introduction
Recently, numerous studies based on traffic-related particulate matter (PM) emissions have been conducted for air quality management and control of urban atmospheres. Most previous studies have focused on the emissions of particulate matter from engine exhaust (Abdul-Khalek et al. Citation1998; Kittelson Citation1998; Harris and Maricq Citation2001; Zhang and Wexler Citation2004; Wang et al. Citation2006). However, in the past few decades, there have been comprehensive technical improvements such as new combustion strategies, fuel enhancement, and after-treatments for the reduction of PM from engine exhaust emissions in order to meet stringent regulation requirements (Takahashi et al. Citation1996; Konstandopoulos et al. Citation2000; Chen et al. Citation2007; Splitter et al. Citation2010; Kokjohn et al. Citation2011; Jones et al. Citation2012). As a result, the relative contribution from non-exhaust sources such as tire particles emitted from the interaction between the tire and the road surface, brake particles, and roadway particles has increased. Kumar et al. (Citation2013) classified 11 non-vehicle exhaust sources (NES) as major sources of nanoparticle emissions in the atmosphere. They demonstrated that the nanoparticles from each non-exhaust source had different characteristics resulting from different formation mechanisms. Among the various non-exhaust sources, several studies have focused on PM formation from tire. Most previous studies concerning PM formation from tire demonstrated that coarse and fine particles (PM10 and PM2.5) were released due to mechanical abrasion between tire and pavement (Kupiainen et al. Citation2005; Thorpe and Harrison Citation2008; Lee et al. Citation2013). However, Dahl et al. and Gustafsson et al. reported the generation of ultrafine particles from tire by utilizing laboratory measurements (Dahl et al. Citation2006; Gustafsson et al. Citation2008). Dahl et al. (Citation2006) determined the number size distributions of ultrafine particles of studded and non-studded tires considering different types of pavement using a road simulator. They reported that the mean particle diameters emitted from the tires ranged between 15 and 50 nm with the highest number concentrations in studded tires; these particles presumably originated from the tire and not the pavement. However, in a study carried out by Gustafsson et al. (Citation2008), they found that ultrafine particles with sizes of 30–50 nm only occurred during tests with studded tires, and the major contributor of ultrafine particles is pavement wear rather than tire wear. Kwak et al. (Citation2013, Citation2014) also observed the generation of not only coarse and fine particles, but also ultrafine particles of the tire under various driving conditions, including constant speed, cornering, and harsh braking. They reported that the frictional heat due to the interaction between the tire and road pavement under harsh braking conditions could evaporate the organic compounds of tire, and consequently, ultrafine particles could be formed via gas to particles conversion, i.e., a nucleation process. In a study conducted by Mathissenet et al. (Citation2011), on-road measurements of ultrafine particles were obtained from tire-road interfaces and near the brake pad under different driving conditions using summer tires. The results revealed that the nanoparticle numbers significantly increased near the tire-road interface and brake pad during braking events. The morphological characteristics of the non-exhaust ultrafine particles have been reported in a various studies. Dahl et al. (Citation2006) and Kwak et al. (Citation2014) sampled roadway particles under various driving conditions and classified the particle types with respect to morphological and compositional features.
In a preliminary test, we confirmed the formation of nanoparticles from tire under harsh braking conditions using a mobile sampling vehicle, where the experimental apparatus and test methods are presented in our previous study (Kwak et al. Citation2013). shows the particle number concentration with respect to the driving speed. Under harsh braking conditions, the number concentration of particles significantly increased and the size distribution of the particles had peak values of 10–20 nm. Based on previous studies, including our experiments, we expected that the volatilization of tire was initiated due to the frictional heat between the tire and pavement under the braking conditions. Consequently, nanoparticles could be formed during the formation process, as shown in .
Figure 1. (a) On-road nanoparticle formation from tire under braking conditions and (b) a schematic of the formation procedure of nanoparticles from tire.
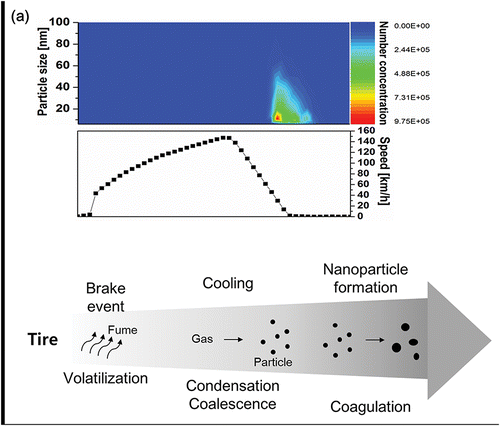
In this study, we conducted laboratory measurements in order to confirm our hypothesis of the formation mechanism of nanoparticles, which was mainly due to the volatilization of organic compounds in the tire tread. The number size distribution and number concentration of particles from the tire tread were measured and morphological and compositional analyses were conducted under various reaction temperatures, heating, and cooling conditions.
Material and methods
The aim of this study was to characterize the formation of nanoparticles from tires by volatilization of tire materials. We investigated the generation of tire nanoparticles under various temperatures and flow rates of air.
Experimental apparatus
As shown in , our experimental setup consists of three components: air supply, reaction, and measurement sections. Air was fed into the reaction chamber and a mass flow controller (MFC, F-206AI; Bronkhorst High-Tech B. V., The Netherlands) was used to adjust the flow rate of air. In order to supply clean air, a high efficiency particulate air (HEPA) filter was installed at the inlet of the reaction chamber. In the reaction chamber, the temperature of the tire specimen was controlled using a hot plate (PC-400D; Corning Inc., USA). The reaction temperature of the tire specimen was measured using a thermocouple at the specimen dish. In the measurement section, a fast mobility particle size spectrometer (FMPS, FMPS 3091; TSI Inc., Shoreview, MN, USA) that could measure the time-resolved size and number distributions of nanoparticles in the range of 5–560 nm was used.
The airborne nanoparticles from the tire tread were collected onto TEM substrate grids (657-200-Cu; Ted Pella, Inc., Redding, CA, USA) to investigate the morphology of the nanoparticles. A transmission electron microscope (TEM, Tecnai G2 F30 ST; FEI Company, Hillsboro, OR, USA) and energy dispersive spectrometer (EDS, Tecnai G2 F30 ST; FEI Company) were utilized for the morphology and elemental analyses, respectively. All the experiments were conducted using commercially available tires (KUMHO KH25 225/45/R18, UTQG (Uniform Tire Quality Grade Standards) tread wear 480).
Experimental procedures
First, a thermo-gravimetric analysis (TGA, TG 209 F3 Tarsus; NETZSCH-Gerätebau GmbH., Selb, Germany) was conducted to determine the thermal characteristics of the tire tread. A tire sample was prepared in the form of powder. The tire powder was produced by mechanical abrasion using sand paper with a medium coarse abrasiveness (80-grit, average particle size of 201 μm). The as-prepared tire powder was heated from 25 to 800°C at a heating rate of 10°C/min. TG analysis was carried out in air and nitrogen atmospheres to compare the volatilization and oxidation characteristics of the tire tread. The carbonaceous contents in the tire tread were analyzed via OC/EC analysis (OCEC Lab Instrument model 5; Sunset Laboratory Inc., Tigard, OR, USA).
In the reaction chamber, the tire powder was heated to 400°C and the real-time number size distributions of the generated particles were measured using an FMPS. We adjusted the reaction temperature, heat flux, and flow rate of air to investigate the relationship between the temperature and particle generation. Morphological and compositional analyses were carried out using TEM and EDS analyses. Nanoparticles were directly collected at a flow rate of 0.3 LPM on TEM grids using a mini particle sampler (MPS, ECOMESURE, Janvry, France). The particle sampling on the TEM grid and number size distribution measurements were performed under the same test conditions.
Results and discussion
Thermal properties of the tire
The TGA curves of the tire tread obtained under different atmospheres are shown in . The TGA profiles of the tire tread weight loss were obtained for the temperature range of 100 to 800°C. For both of the atmosphere conditions, the onset of thermal degradation was observed at about 200°C. Mass losses of tire tread can be presented as a derivative thermo gravimetric (DTG) curve, which was expressed as the rate of mass change with respect to the temperature. In the case of the air atmosphere, the degradation process of the tire tread by oxidation has a multi-modal distribution and the reaction was completed at 650°C, and a more than 80% weight loss was observed. The main reason for the weight loss could be simply due to the oxidation of carbon in the tire tread. On the contrary, in the case of the nitrogen atmosphere, the TGA curve shows a unimodal profile of weight loss. The reaction was completed at about 535°C, and almost a 60% weight loss of the tire tread occurred during this reaction. Notably, contrary to the air atmosphere, more than 60% of the tire tread was transformed to a gaseous phase by volatilization of the organic compounds in the tire tread. A similar result was obtained from EC/OC analysis, as shown in . EC/OC analysis is the reference method to quantitatively measure organic carbon (OC) and elemental carbon (EC). The EC/OC result confirms that 72.39% of the tire consists of carbonaceous materials. Among these materials, 75% of the carbon could be classified as organic carbon (54% of the total tire) and the remaining 25% of carbon is elementary carbon (18% of the total tire). Generally, it is known that OC could be the primary and secondary origins of particulate matter (Pandis et al. Citation1992; Castro et al. Citation1999). The plausible mechanism of the PM formation is a combined process of volatilization and condensation of organic compounds. The tire could be a potential source of PM emissions due to the large amount of OC in the tire tread. From the TGA and EC/OC results, the organic compounds in the tire tread could be transformed to a gaseous phase when the tire tread reaches a certain temperature.
Nanoparticle generation from the tire tread
Initiation of particle generation
To initiate the volatilization process of the tire tread, a certain amount of heat was provided to the tire specimen using a hot plate. Based on the TGA results, the onset of the reaction temperature was about 200°C for all of the atmospheric conditions.
The tire specimen was heated up to 200°C and the flow rate of air (carrier gas) was fixed at 150 lpm. shows the number size distributions of the generated particles. Note that when the temperature reached above 160°C, the formation of particles could be initiated. The results showed that the onset temperature in the reaction chamber was in good agreement with the TGA results. The number concentration of the tire particle increased with increasing temperature. For all of the temperature conditions (after the onset of reaction, T > 160°C), unimodal size distribution were observed. The median diameter ranged from 50 to 60 nm. The geometric standard deviation of the lower temperature conditions (, 130–200°C) was similar regardless of the temperature and ranged from 1.61 to 1.67. The number size distributions of the particles were quasi-monodispersed for the lower temperature conditions. For higher temperature conditions (, 200–350°C), tire particles were produced at high concentrations (2.0106−1.5
107 #/cm3). The number size distributions of the particles were broader than those obtained from the lower temperature conditions, that the particles had a poly-dispersed distribution. The results from and indicate that the number concentration of tire particles increased with increasing temperature. However, the peak number concentrations in and for the same temperature of 200°C do not correspond with each other. Although the temperature was the same, there is a huge difference in the peak number concentrations. To investigate this discrepancy, the effect of the heating rate on the formation of particles was examined under various heating rates.
Particle generation under a uniform heating rate
shows the number size distributions of particles according to the heating rate with values of 0.2°C/s, 0.4°C/s, and 0.6°C/s for a fixed initial temperature (T = 270°C) and continuous initial temperatures of T = 260°C, 280°C, and 320°C, respectively (). The flow rate of air was fixed at 150 lpm for all of the test conditions. First, in the case of the constant initial temperature (), the particle number increased with increasing heating rate. During the heating conditions, the differences of the number concentrations at the median diameters were 0.85106, 3.9
106, and 5.5
106 #/cm3. This result implies that the particle concentration increased as the heating rate increased.
Figure 5. Number size distributions for airborne tire nanoparticles obtained under various heating rates and temperatures.
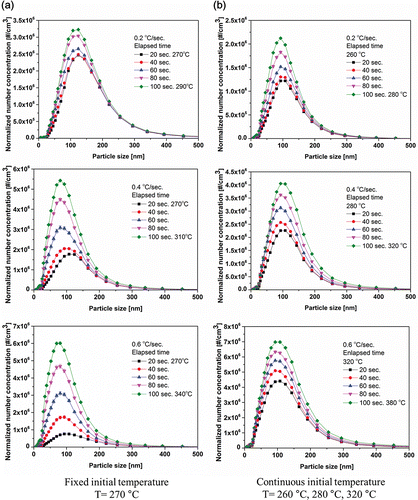
In the case of the continuous initial temperature (), the differences of the number concentrations at the median diameter were 1106, 2
106, and 3
106 #/cm3. The number concentration of particles was proportional to the heating rate. The results showed a more reasonable tendency to increase compared to the results of the fixed initial temperature conditions. From these results, the generation of nanoparticles from tire tread dominantly depends on the heating rate at the onset temperature. Above the onset temperature of the particle formation, the total number concentration of particle is predominantly related to the heating rate.
In the case of excessive thermal loads such as braking events, the harsh braking conditions may generate more nanoparticles compared to normal braking conditions since the tire undergoes more severe friction, resulting in more frictional heat.
Effect of the flow rate of air
The air flow played two important roles in this study. One is for the carrier gas to deliver the particles to the measurement section and the other is for the cooling gas of the tire fumes. Generally, the vapor concentration and cooling rate primarily determine the growth of particles during the gas to particle conversion process. To examine the effect of the cooling rate on the formation of particles, the change of the number size distribution of particles was measured according to the flow rate. For all of the temperature conditions, the geometric mean diameter of the particles decreased with increasing air flow, as shown in . This demonstrates that the growth of particles had a strong dependency on the cooling rate. These results can be explained by the theory of particle collisions. The collision frequency for particle collisions of volumes and
can be represented by Equation (Equation1
[1] ) (Friedlander Citation1977):
[1]
Figure 6. Comparison of the median diameter as a function of the flow change in flow rate of air at constant temperatures of (a) 270°C, (b) 310°C, (c) 350°C, and (d) 400°C (where, ,
).
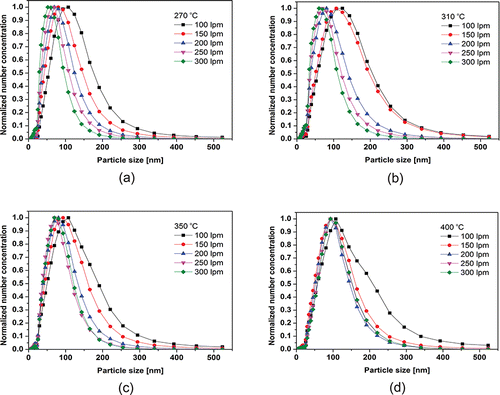
After the nucleation of particles, the particles will grow via coagulation and agglomeration. During this process, the particle growth is governed by random collisions of the particles and vapor molecules. At the initial stage of particle formation, the incident air cools down the gaseous tire fumes and the tire fumes undergo rapid cooling. Then the gaseous tire fumes transform into primary particles. When the cooling is rapid, a shorter nucleation process occurs and smaller particles are acquired.
After the formation of particles, the particles can agglomerate with each other, and larger particles can be formed by the agglomeration of primary particles. If the air flow rate is high, the atmospheric temperature decreases. In this circumstance, the tire fume with high concentration may rapidly condense and the fresh nucleated particles could be formed. On the other hands, the high flow rates of air impede the agglomeration of particles since the collision frequency of particles is proportional to the square root of temperature, and the dilution effect by abundant air is not negligible under high flow rate conditions. Therefore, the number size distribution of particles became narrow and the geometric standard deviation of the size distribution decreased as the flow rate of air increased. This relationship was maintained as the flow rate of air was increased further.
shows the size and number distribution depending on the reaction temperature under a high flow rate of air. For a fixed flow rate of air, the median diameter decreased slightly as the temperature increased. The size distribution became broader as the temperature increased since the number concentration of particles and the collision frequency also increased as the temperature increased. Under these conditions, the high concentration of particles led to the higher probability of agglomeration of particles and the primary particles could grow by agglomeration.
Morphological and compositional characteristics of tire nanoparticles
The TEM/EDS analysis result of the tire nanoparticles are shown in . The tire nanoparticles seem to have a black dot shape. In the case of a lower temperature (170°C, ), most of the particles had diameters less than 100 nm with a circular or faint stain shape. Notably, a relatively big bubble was occasionally observed in . These bubble particles rapidly evaporated under high vacuum and strong electron beam intensity in the TEM, indicating that they may consist of volatile materials (e.g., volatile organic carbon, nitrate, or sulfate). This finding is consistent with previous studies conducted by Kwak et al. (Citation2014) and Smith et al. (Citation2012), who reported that sulfur-containing particles were electron beam sensitive and damaged easily, resulting in a rapid change of morphology and the appearance of moving pores within a particle. In the EDS analysis, the particle mainly consisted of C, O, S, Si, and Cu. This particle is expected to be generated from the tire tread, since sulfur is an essential substance for the vulcanization process in tire manufacturing.
Figure 8. TEM/EDS analyses of tire nanoparticles at reaction temperatures of (a) 150°C and (b) 300°C.
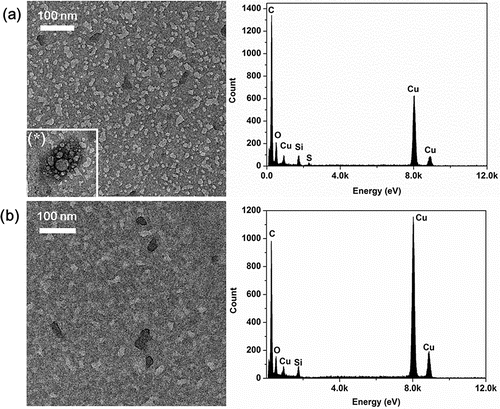
In the case of higher temperature condition (300°C, ), larger particles with a deep black color were observed. The average diameter ranged between 50 and 100 nm and the shape of the particles was irregular. The elemental composition of these particles included C, O, and Si, which are the main substances of tire rubber. Compared to a previous study by Dahl et al. (Dahl et al. Citation2006; Kwak et al. Citation2014), some particles (Types 1 and 4) have a similar morphology and elemental composition to our particles.
We believe that our TEM/EDS results provide morphological and compositional information of tire nanoparticles and help to distinguish tire-related nanoparticles among the particles obtained from various non-exhaust sources.
Conclusion
The formation process of nanoparticles from tire tread is complex and active research area. Although a few plausible mechanisms for the formation of nanoparticles have been reported, there has not been strong evidence to identify the formation process of tire nanoparticles. To establish the formation mechanism of tire nanoparticles, laboratory measurements in a reaction chamber were conducted under various heating and cooling conditions.
We focused on the effects of the reaction temperature and heating rate of tire tread on the formation of nanoparticles. The results showed that the formation of tire nanoparticles was initiated above the onset temperature of the tire, and the total number concentration depended more on the heating rate than the reaction temperature.
The mean diameter of the generated particles varied depending on the cooling conditions. For all of the test conditions, the number size distributions of the particles from the tire tread have a unimodal and log-normal distribution. The geometric mean diameter of the particles was in the range of 60–100 nm. The TEM/EDS analyses of the nanoparticles from the tire tread revealed that the particles consist of ultrafine particles with a nearly spherical shape and the elemental compositions mainly consisted of C, O, Si, and S from rubber in the tire tread.
Based on our experimental results, we propose the formation mechanism of tire nanoparticles in which the organic compounds in the tire tread volatilize due to frictional heat under braking condition. Above a reaction temperature of 160°C, the nucleation of tire fumes is initiated and a number of nanoparticles can be formed. We believe that our study provides comprehensive information such as the number size distribution, morphological, and compositional features of tire nanoparticles, and helps to distinguish the tire-related nanoparticles among the particles from various non-exhaust sources.
Uncertainties still remain in the identification of the possible emission source of nanoparticles under on-road driving conditions because nanoparticles from tire tread, brake pads, and bitumen in the pavement are simultaneously emitted. As a result, they may be mixed and sampled together in the inlet. Therefore, further research concerned with the investigation of the physical and chemical characteristics of ultrafine particles from brake pads and asphalt pavement is required.
Funding
The present study was supported by the Center for Environmentally Friendly Vehicles (CEFV) under the project “Development of the eco-friendly tire for reduction of carbon dioxide and tire wear particles” through the Ministry of Environment (ME, Republic of Korea).
References
- Abdul-Khalek, I. S., Kittelson, D., Graskow, B. R., Wei, Q., and Bear, F. (1998). Diesel Exhaust Particle Size: Measurement Issues and Trends. SAE Technical Paper, SAE 980525.
- Castro, L., Pio, C., Harrison, R. M., and Smith, D. (1999). Carbonaceous Aerosol in Urban and Rural European Atmospheres: Estimation of Secondary Organic Carbon Concentrations. Atmos. Environ., 33:2771–2781.
- Chen, H., Shi-Jin, S., and Jian-Xin, W. (2007). Study on Combustion Characteristics and PM Emission of Diesel Engines Using Ester–Ethanol–Diesel Blended Fuels. Proc. Combust. Inst., 31:2981–2989.
- Dahl, A., Gharibi, A., Swietlicki, E., Gudmundsson, A., Bohgard, M., Ljungman, A., Blomqvist, G., and Gustafsson, M. (2006). Traffic-Generated Emissions of Ultrafine Particles from Pavement–Tire Interface. Atmos. Environ., 40:1314–1323.
- Friedlander, S. K. (1977). Smoke, Dust and Haze: Fundamentals of Aerosol Behavior. Wiley-Interscience, New York, 1977. p. 333.
- Gustafsson, M., Blomqvist, G., Gudmundsson, A., Dahl, A., Swietlicki, E., Bohgard, M., Lindbom, J., and Ljungman, A. (2008). Properties and Toxicological Effects of Particles from the Interaction Between Tyres, Road Pavement and Winter Traction Material. Sci. Total Environ., 393:226–240.
- Harris, S. J., and Maricq, M. M. (2001). Signature Size Distributions for Diesel and Gasoline Engine Exhaust Particulate Matter. J. Aerosol Sci., 32:749–764.
- Jones, A. M., Harrison, R. M., Barratt, B., and Fuller, G. (2012). A Large Reduction in Airborne Particle Number Concentrations at the Time of the Introduction of “Sulphur Free” Diesel and the London Low Emission Zone. Atmos. Environ., 50:129–138.
- Kittelson, D. B. (1998). Engines and Nanoparticles: A Review. J. Aerosol Sci., 29:575–588.
- Kokjohn, S., Hanson, R., Splitter, D., and Reitz, R. (2011). Fuel Reactivity Controlled Compression Ignition (RCCI): A Pathway to Controlled High-Efficiency Clean Combustion. Int. J. Engine Res., 12:209–226.
- Konstandopoulos, A. G., Kostoglou, M., Skaperdas, E., Papaioannou, E., Zarvalis, D., and Kladopoulou, E. (2000). Fundamental Studies of Diesel Particulate Filters: Transient Loading, Regeneration and Aging. SAE Paper, SAE 2000-01-1016, p. 1016.
- Kumar, P., Pirjola, L., Ketzel, M., and Harrison, R. M. (2013). Nanoparticle Emissions From 11 Non-Vehicle Exhaust Sources–A Review. Atmos. Environ., 67:252–277.
- Kupiainen, K. J., Tervahattu, H., Räisänen, M., Mäkelä, T., Aurela, M., and Hillamo, R. (2005). Size and Composition of Airborne Particles from Pavement Wear, Tires, and Traction Sanding. Environ. Sci. Technol., 39:699–706.
- Kwak, J.-H., Kim, H., Lee, J., and Lee, S. (2013). Characterization of Non-Exhaust Coarse and Fine Particles from On-road Driving and Laboratory Measurements. Sci. Total Environ., 458:273–282.
- Kwak, J., Lee, S., and Lee, S. (2014). On-Road and Laboratory Investigations on Non-exhaust Ultrafine Particles from the Interaction Between the Tire and Road Pavement Under Braking Conditions. Atmos. Environ., 97:195–205.
- Lee, S., Kwak, J., Kim, H., and Lee, J. (2013). Properties of Roadway Particles from Interaction Between the Tire and Road Pavement. Int. J. Automot. Technol., 14:163–173.
- Mathissen, M., Scheer, V., Vogt, R., and Benter, T. (2011). Investigation on the Potential Generation of Ultrafine Particles from the Tire–Road Interface. Atmos. Environ., 45:6172–6179.
- Pandis, S. N., Harley, R. A., Cass, G. R., and Seinfeld, J. H. (1992). Secondary Organic Aerosol Formation and Transport. Atmos. Environ., Part A. Gen. Topics, 26:2269–2282.
- Smith, S., Ward, M., Lin, R., Brydson, R., Dall'Osto, M., and Harrison, R. M. (2012). Comparative Study of Single Particle Characterisation by Transmission Electron Microscopy and Time-Off-Flight Aerosol Mass Spectrometry in the London Atmosphere. Atmos. Environ., 62:400–407.
- Splitter, D., Reitz, R., and Hanson, R. (2010). High Efficiency, Low Emissions RCCI Combustion by Use of a Fuel Additive. SAE Int. J. Fuels Lubricants, 3:742–756.
- Takahashi, N., Shinjoh, H., Iijima, T., Suzuki, T., Yamazaki, K., Yokota, K., Suzuki, H., Miyoshi, N., Matsumoto, S.-i., and Tanizawa, T. (1996). The New Concept 3-Way Catalyst for Automotive Lean-Burn Engine: NO x Storage and Reduction Catalyst. Catal. Today, 27:63–69.
- Thorpe, A., and Harrison, R. M. (2008). Sources and Properties of Non-Exhaust Particulate Matter from Road Traffic: A Review. Sci. Total Environ., 400:270–282.
- Wang, J., Storey, J., Domingo, N., Huff, S., Thomas, J., and West, B. (2006). Studies of Diesel Engine Particle Emissions During Transient Operations Using an Engine Exhaust Particle Sizer. Aerosol Sci. Technol., 40:1002–1015.
- Zhang, K. M., and Wexler, A. S. (2004). Evolution of Particle Number Distribution Near Roadways—Part I: Analysis of Aerosol Dynamics and Its Implications for Engine Emission Measurement. Atmos. Environ., 38:6643–6653.