ABSTRACT
This article presents a cylindrical counter-current flow diffusion denuder with high efficiency penetration of nanoparticles, for non-specific removal of trace gases from an air flow. The denuder was designed to exchange gases in the sample flow by diffusion to the purge flow across a cylindrical microporous glass tube. Laboratory test results indicated that removal efficiencies of gases increased with a lower sample flow rate and a higher sample to purge flow rate ratio. Additionally, the pore size of the microporous glass did not affect gas removal efficiency and particle penetration following optimization of sample and purge flow rate conditions. Significantly high particle penetration was obtained for the counter flow denuder technique (94% penetration for 20 nm of polystyrene latex particle [PSL]) that agreed with theoretical estimation attributed to diffusion loss.
Copyright © 2017 American Association for Aerosol Research
EDITOR:
1. Introduction
Many applications for aerosol particle measurement and calibration particle generation require removal of gaseous components. Diffusional separation techniques are widely used for this purpose (Kitto and Colbeck Citation1999; Hogrefe et al. 2011). Several denuder designs have been developed to increase gas removal efficiency including cylindrical, annular, parallel-plate, and honeycomb configurations (Kitto and Colbeck Citation1999). Additionally, many studies have used different chemical coatings to trap specific gases and selective membranes for denuder walls to improve the collection efficiency of the gases. For example, basic coatings such as sodium carbonate have been used for acidic gases such as nitrous acid. Similarly, adsorbents such as activated carbon coatings have been used to trap organic compounds (Kitto and Colbeck Citation1999; Lane Citation1999). However, the use of these denuder techniques is limited when less reactive and/or inert gases (i.e., CO2, H2O, CO, NO) need to be removed continuously. Consequently, a technique for measurement and/or generation of aerosol particles, which reduces gaseous interference due to absorption and adsorption on to the chemical substrate and removes non-specific gases, was needed. A counter-current parallel-plate membrane diffusion denuder was designed and developed to remove non-specific gases (Ruiz et al. 2006; Ashok and Gupta Citation2012). Gaseous pollutants were removed by diffusion from an inner sample channel to a purge channel where clean air was passed in a counter-flow fashion. However, particle loss in the denuder, especially of nanoparticles, was much higher than theoretically expected. Therefore, further investigation of different types of counter flow denuders that use conductive microporous membranes and experience less particle loss is required.
Recently, aerosol mass spectrometry employing the Aerodyne aerosol mass spectrometer (AMS; Canagaratna et al. Citation2007) has been widely used for real-time characterization of the size, chemical composition, and in situ measurement of aerosol nanoparticles without filter sampling technique, which has been used during field observation of oxygenated organic aerosol nanoparticles from secondary sources (Ahlm et al. Citation2012). Laser-vaporization AMS has been used for the measurement of metal nanoparticles (Nilsson et al. Citation2015). The AMS has an inlet system that comprises an aerodynamic lens for pre-concentration of aerosol particles during removal of gases. However, several studies indicate that the signals generated by gas compounds with concentrations over a few dozen ppm can influence the interpretation of the signal of the particle-phase compounds (Collier and Zhang Citation2013). Thus, such interferences with the aerosol mass spectra further complicate accurate determination of H2O+, CO2+, and CO+ signals from oxygenated organic species sampled in air (Collier and Zhang Citation2013; Canagaratna et al. Citation2015). Separation of signals originating from organic aerosol particles and other background trace gases in the air becomes difficult. Therefore, gas removal techniques may be necessary even when the aerodynamic lens technique is used.
In this study, a cylindrical counter flow denuder was developed using a microporous glass tube instead of Teflon membranes to obtain a denuder system that had high efficiency nanoparticle penetration and removal of non-specific trace gases. The denuder performance for particle loss and trace gas removal was evaluated using experimental parameters in a laboratory test and compared with the result of Teflon membranes.
2. Materials and methods
2.1. Design of the counter flow denuder
A schematic diagram of the counter flow denuder is illustrated in . The cylindrical counter flow denuder had two concentric tubes; an inner porous tube through which aerosol particles were passed as sample flow; and an outer tube made of seamless stainless steel (SUS 316L) with counter-current purge gas supply as purge flow, in opposite direction to the sample flow. The inner tube for sample gas flow was designed with maximum dimensions and had a center channel of 250 mm length and 5 mm outer diameter (o.d.) through which the aerosol and gas were passed. The inlet and outlet ports (tube connections) of the sample flow had an o.d. of 6.35 mm. Several evaluation tests for particle loss were performed with sample flow tubes made of different types of porous inner tubes. The outer tube as purge gas flow channel had a center channel with dimensions similar to that of the sample flow, but with o.d. of 9.53 mm and two T-shaped pipe joints and tube connections with o.d. of 6.35 mm at each end to supply and remove the purge air.
Three types of porous inner tubes (5 mm o.d.; 250 mm length) were used to separate the sample and the purge channels: (1) porous glass (Shirasu Porous Glass [SPG], SPG Techno Co. Ltd., Japan), (Yamazaki et al. Citation2002), with a pore size of 0.1 μm (as measured by Hg intrusion) and thickness of 0.4 mm; (2) porous glass with pore size of 0.05 μm and thickness of 0.4 mm (SPG, SPG Techno Co. Ltd., Japan); (3) Poreflon® microporous tube (Sumitomo Electric Industries Ltd., Japan) made of poly-tetra-fluoroethene (PTFE), with pore size of 1 μm (as measured by bubble point test) and thickness of 1 mm. This PTFE counter flow denuder was used only to measure particle loss. For the Poreflon® microporous tube, the inner tube was sandwiched by a stainless tube with o.d. of 6 mm, and female connector of the T-shaped pipe joint. An O-ring gasket was set in the groove of the T-shaped pipe joint along the edges of the inner tube to minimize leaks. Leak tests were conducted for all the denuders by passing a 0.15 L/min flow through the sample channel and measuring flow recovery downstream using a soap bubble gas flow meter (SF-1U-VP-3, HORIBA STEC Co. Ltd., Japan), while the remaining ports were open. The leak-free denuder was approved if at least 2% of the flow (0.01 L/min), which is within full scale of a panel mount mass flow controller (MPC9500, Azbil, Japan) was recovered.
2.2. Gas removal efficiency in laboratory evaluation
The denuder performance was evaluated in the laboratory using artificial gases and that included both less reactive and reactive gases. Initial experiments were carried out using CO2 as the test gas with the sample flow rate (Qs) varying from 0.1 L/min to 20 L/min and purge flow rate (Qp) varying from 0.05 L/min to 20 L/min. The Qp and Qs ratio (Qp/Qs) was set at 1, 2, 10, and 20. The purge gas flow used pure air from the cylinder and CO2 was monitored using a non-dispersive infrared detector (NDIR; VIA-510, HORIBA Ltd., Japan).
The ability of the denuder to remove gases using the principles presented in session 3.2 was evaluated with experiments that included both inert and more reactive gases. The evaluation of gas removal efficiency was carried out for CO, NOX, and volatile organic compounds (VOCs) supplied from standard gas cylinders, artificial O3, and ambient humidity. O3 gas was generated using a mercury lamp (Hamamatsu Photonics Corp., Japan) and pure air from the cylinder. The Qs for standard gas sampling and Qp for pure gas were set at 0.15 L/min and 3 L/min, respectively. Concentrations of CO, NOX, O3, and VOCs were measured continuously using the following instruments; CO using non-dispersive infrared gas filter correlation detector (model 48i, Thermo Electron Corp., USA), NOX (NO and NO2) using chemi-luminescence detector (model 42c, Thermo Electron Corp., USA). VOCs premixed standard gas containing benzene, toluene, trimethylbenzene, naphthalene balanced with N2 (NOMG, Sumitomo Seika Chemicals Co. Ltd., Japan), and odor standard gas containing acetaldehyde, butylaldehyde, valeraldehyde, and acetone balanced with N2 (ODOR-J14, Sumitomo Seika Chemicals Co. Ltd., Japan) were used as inputs. The VOCs were measured using proton transfer reaction mass spectrometer (de Gouw and Warneke Citation2007) (PTR-TOF 8000, IONICON Analytik GmbH, Austria) for assigning analyte ions based on the reaction between hydronium ions and analytes in the gas phase such as m/z 79 (benzene), m/z 93 (toluene), m/z 121 (trimethylbenzenes), m/z 129 (naphthalene), m/z 45 (acetaldehyde), m/z 73 (butylaldehyde), m/z 87 (valeraldehyde), and m/z 59 (acetone). The cylinder with pure air was attached to the instrument inlet to adjust the sample flow rate of CO (1 L/min), NOX (0.2 L/min), and O3 (1.2 L/min) for a flow rate of 0.15 L/min for the denuder. To evaluate the humidity removal of the counter flow denuder, sample channels of the inlet and outlet were measured for humidity for 2 weeks using individual hygrometers (HMT333, Vaisala Inc., Finland). The measurements were conducted in outdoor air during summer season (wet season) in Japan. Additionally, water vapor generated by the aerosol atomizer (model 3076, TSI Inc., Shoreview, MN, USA) was tested using Milli-Q water in the laboratory (18 MΩ, Milli-Q Integral, Q-POD Element 10A, Merck Millipore Co., Ltd., Germany).
2.3. Denuder particle loss measurement
shows the system for measuring particle loss in the denuders. Monodispersed polystyrene latex (PSL) particles generated by atomization-drying were classified using a differential mobility analyzer (DMA; model 3081, TSI Inc.) to obtain monodispersed particles of the desired size.
Figure 2. Experimental setup for evaluation of particle loss in counter flow denuders with different pore sizes.
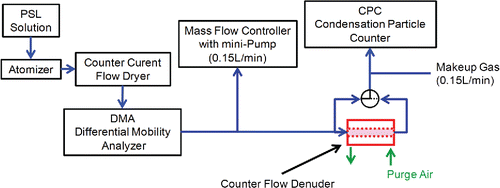
As small residue particles of an unknown impurity following evaporation of the liquid droplet without polystyrene latex spheres (PSL) particles were found, a DMA was placed between the atomizer and the denuder to eliminate the source of polydispersity. The singly charged monodispersed aerosols generated were of sizes 0.021 μm (Thermo Fisher Scientific, USA), 0.048 μm, 0.107 μm, 0.202 μm, and 0.309 μm (JSR, Inc., Japan) in diameter. To account for the diffusion loss of sub-0.02 μm particles, 0.01 μm diameter particles were generated as residuals of the 0.021 μm PSL particles classified by DMA as a reference. A previous study reported that particle loss from a glass wall denuder occurred while using monodispersed aerosols with different electrical charges and particle loss at a given diameter slightly increased with increasing average charge of the aerosol (neutral < Boltzmann < singly charged; Ye et al. Citation1991). During a preliminary check for this study, a slight difference in the particle penetration (2–5%) between neutral and singly charged particles was observed. Therefore, a neutralizer and an electrical condenser were not interposed downstream DMA. The DMA output was used directly for singly charged particles. Particle loss in the denuder system under test was determined by measuring particle number concentration at upstream and downstream locations of the test system and comparing the particle loss in the absence of the test system. Upstream and downstream concentrations were measured using one condensation particle counters (CPC; model 3025A, TSI Inc.) with a three-way valve.
3. Results and discussion
3.1. Initial test for purge gas flow rate
The purpose of the laboratory evaluations was to determine the gas removal efficiency of the denuder as a function of different experimental parameters. The parameters investigated were: (1) sample flow rate (Qs), (2) purge to sample flow rate ratio (Qp/Qs), and (3) pore size of the microporous tubes.
The initial laboratory evaluation of the counter flow denuder was carried out using CO2 as the cylinder gas with Qs from 0.1 L/min to 0.5 L/min and Qp from 0.1 L/min to 20 L/min. Gas concentrations were measured at the inlet (Cs,in,i) and outlet (Cs,out,i) of the sample flow. Ei, the gas removal efficiency of gas species i, was calculated using Equation (Equation1[1] ):
[1]
The gas removal efficiency ECO2 of the counter flow denuder was evaluated using CO2 concentrations at the outlet of the sample flow channel using NDIR.
shows the gas removal efficiency ECO2 with variation in Qp/Qs ratio and Qs. As expected, a lower flow rate increased residence time in the denuder and increased gas removal. During the AMS operation, Qs was 0.15 L/min but depended on the design of the aerodynamic lens. When Qs of 0.15 L/min was used, higher removal efficiencies of ECO2 was found at higher Qp/Qs (i.e., 98.3% for pore size 0.05 μm and 99.9% for pore size 0.1 μm at 3 L/min, Qp/Qs = 20) than at lower flow rates (i.e., 73.6% for pore size 0.05 μm and 83.8% for pore size 0.1 μm at 0.15 L/min, Qp/Qs = 1). This was expected as a higher Qp/Qs ratio increased the concentration gradient (difference in the partial pressures) between the purge and the sample channels, thus increasing the diffusion rate between channels. When Qp increased and Qp/Qs increased from 10 to 20, ECO2 was not very different and seemed to have achieved equilibrium or saturation of the gas exchange capacity of diffusion between the sample and purge channels. In a previous experiment using glass porous tube as the air to argon gas exchange device for aerosol inlet system of inductivity coupled plasma mass spectrometer (ICP-MS), remaining concentration of N2 was measured and considered complete when N2 concentration after the exchange was less than 0.01% (100 ppmv; Nishiguchi et al. Citation2008; Tabersky et al. Citation2013). Using this criterion, the recommended Qp should be at least 10 times higher than the Qs to maintain gas removal efficiency. The air to argon gas exchange device used a different gas (Ar), design (i.e., 0.1 μm pore size; 10 mm inner diameter, length 450 mm), and gas flow rate (0.35 L/min to 0.8 L/min). However, the same explanation applies to this case as a higher sample flow rate decreased residence time in the denuder, therefore decreasing gas removal efficiency. When the sample flow rate was increased to more than 0.2 L/min, ECO2 decreased for all sample flow rates examined, with ECO2 values ranging from 99% to 84% at Qp/Qs of 20 for 0.1 μm pore size. The flow rate of the counter flow denuder was set at 0.15 L/min, as it was used as an inlet of the AMS. To maintain high efficiency trace gas removal, all experiments used a sample flow of 0.15 L/min and purge gas flow rate of 3 L/min.
3.2. Gas removal efficiency in laboratory performance evaluation
The behavior of trace amounts of gases in a denuder system is well explained by a theoretical equation based on Fick's laws of diffusion that was derived by Gormley and Kennedy (Citation1949). Removal efficiency (Ei) for a cylindrical denuder can be described using Equations (Equation2[2] ) and (Equation3
[3] ):
[2]
Δ was calculated as[3]
where Di is the diffusion coefficient of the gas species i in air (cm2/s), L is the length of sample flow channel (25 cm), γ is the viscosity coefficient of air (0.152 cm2/s, 20°C, 1 atm), Re is the Reynolds number, and d is the inner diameter of the sample flow channel (0.42 cm). lists Ei, for gas species calculated using the Gormley and Kennedy equation at Qs of 0.15 L/min. Most gas species tested in this study showed 100% Ei, except naphthalene, which showed 99.7%. An Ei of 100% is explained by diffusion theory that would lead to null concentrations at the walls.
Other trace gases were efficiently removed (Ei more than 93%) during this study (). For instance, ECO was 99.8% and 99.9% for pore sizes 0.05 μm and 0.1 μm, respectively. However, at different Qp (), the ECO2 showed different values depending on the sample and purge gas flow rates and ratios, which cannot be explained by diffusion theory only. A previous study using a medium sized parallel-plate counter flow denuder also showed lower ECO of 90% compared to the predicted ECO of 99.97% as per the diffusion theory of Gormley and Kennedy for rectangular/parallel plate shape (Ruiz et al. 2006).
Possible explanations for this unexpected difference in ECO can be (1) Qp/Qs ratio, (2) residence time, and (3) permeability at the denuder wall (which is assumed to allow only diffusion process).
The first possible explanation suggests that Qp/Qs was higher (value of 20) than for the medium sized parallel-plate counter flow denuder (Qp/Qs of 2). Efficient ECO2 (87% and 96% in ) were observed in this study (Qp/Qs = 2 with 0.15 L/min of sample flow). Therefore, efficient ECO cannot be explained only by Qp/Qs ratio.
Second possible explanation is that the residence time τs in the sample flow was different. A lower flow rate of this compact cylindrical counter flow denuder increases residence time in the denuder, hence increasing gas removal (). However, this compact cylindrical counter flow denuder has shorter residence time (τs = ca. 0.4 s at 0.15 L/min) compared to the medium sized parallel-plate counter flow denuder (τs = ca. 17 s at 5 L/min). Therefore, a comparison between compact cylindrical denuder and parallel-plate counter flow denuder indicated that efficient ECO cannot be explained by residence time of the sample gas flow only.
The third possible explanation considers the permeability at the denuder wall; the molecules of trace gas that reach the denuder wall are trapped and/or moved outside the wall, leading to reduced to nil concentrations at the walls. The permeability at the denuder wall is dependent on different pore sizes and thickness of the denuder wall (membrane). The compact cylindrical counter flow denuder had smaller (0.05 μm or 0.1 μm) and thicker (0.4 mm) pore size compared to the medium sized parallel-plate counter flow denuder (pore size of 0.2 μm or 1.5 μm and thickness of 0.2 mm or 0.09 mm). The difference in permeability may be related to other factors, for instance, uniformity of the microporous structure of the membrane.
To test the effects of varying porous structure of the denuder wall, different pore sizes of 0.05 μm and 0.1 μm were compared using gas species with different chemical structures and diffusion coefficients. Ei for some gases (i.e., NO, NO2, Benzene, Toluene, and Xylene) did not show any significant difference between pore sizes of 0.05 μm and 0.1 μm (Ei difference of 1.7–3.9%, ). This result indicated that the pore size did not contribute to the difference in gas removal efficiency for this study. Using different pore sizes of SPG membrane (i.e., smaller than 0.05 μm or larger than 0.1 μm) may have explained the difference in gas removal efficiency due to the porosity of denuder wall. However, other pore sizes could not be procured from the manufacturer at the time of the experiment, and further experimentation will be beyond the scope of this article.
illustrates the relative humidity (RH %) at the inlet and outlet of the sample gas flow for ambient atmosphere. The RH ranges were 36–93% (average 75%) at the inlet of the denuder (ambient air) and 6–10% (average 7%) at the outlet of denuder, respectively. It is known that organic and inorganic aerosol particles in humid air often show hysteresis depending on their RH history and crystallization of aerosol particles may occur rather than RH of 35%. This result indicated that the counter flow denuder needs to be equipped with a dry air feed method capable of maintaining low humidity air in a predetermined range. Additionally, water vapor generated by the aerosol atomizer was tested using pure water in the laboratory. Figure S1 shows the RH of the atomizer gas. The result indicated that the counter flow denuder is also useful as an aerosol particle dryer that can efficiently remove water vapor while minimizing particle loss. As aerosol particles are subsequently passed through a typical diffusion denuder and possible contamination of silica gel dust from the inner wire mesh screen may occur, the microporous inner tube will assist in prevention of contamination.
3.3. Mass balance in laboratory performance evaluation
To demonstrate that gases were removed by diffusion only and not by loss from the denuder wall, mass balance (MBi) of gas species i was calculated as the ratio of gas being removed from the sample channel to the gas mass leaving the purge channels, as shown in Equation (Equation4[4] ):
[4]
where Cp,i and Cs,i are gas concentrations of gas species i of the purge and sample channels, respectively. A mass balance of less than 100% would indicate wall depositions or similar processes.
To confirm the validity of this experiment, MBi of less reactive gases like CO and NO were tested. Additionally, reactive gases and other organic gases that have the different chemical characteristics or molecular weight, including reactive gases (O3 and NO2), aromatics as hydrophobic less reactive VOCs (benzene, toluene, xylene, trimethylbenzene, and naphthalene), and aldehydes/ketones as hydrophilic VOCs (acetaldehyde, butylaldehyde, valeraldehyde, and acetone) were tested.
Mass balance of 102% for MBCO and 99% for MBNO were observed. Similar results were also observed for NO2 and O3 with MBNO2 of 99% and 96%, and MBO3 of 100% and 95%, for 0.05 and 0.1 μm pore size, respectively. These results indicated that less reactive gases like CO and NO, as well as reactive gases like NO2 and O3 were removed efficiently by the diffusion process. Additionally, there was no significant difference due to pore size in this study. These results were surprising as different results were observed in the PTFE counter flow denuder test as a large fraction of O3 was lost to the denuder walls (Ruiz et al. 2006). One possible explanation for this unexpected result is the difference in surface area between the SPG and PTFE counter flow denuders. However, since there were no descriptions of the surface area, further details are not known. Another possible explanation for this unexpected result is that the counter flow denuder in this study was more compact with less residence time τp in the purge gas flow τp = ca. 0.2 s for the compact cylindrical counter flow denuder and τp = ca. 10 s for the PTFE counter flow denuder system.
The results of mass balance for other VOCs indicated that the tendency depended on different chemical characteristics or molecular weights of the gases (). For hydrophobic VOCs with low molecular weight (benzene, toluene, and xylene), MBi ranged from 96 to 116%, indicating that the VOCs were removed by diffusion. However, for hydrophobic VOCs with middle molecular weight (trimethylbenzene and naphthalene), MBi ranged from 17 to 79% indicating that about 21–83% of the VOCs were removed by mechanisms other than diffusion, probably due to deposition on the wall surfaces of the membrane or purge gas channel. For hydrophilic VOCs (acetaldehyde, butylaldehyde, valeraldehyde, and acetone), MBi ranged from 36 to 74%, indicating that about 26–64% of the hydrophilic VOCs in this study were removed by mechanisms similar to hydrophobic VOCs. The SPG membrane was inherently hydrophilic due to the presence of silanol groups on the surface (as reported by the manufacturer and Vladisavljevića et al. Citation2005). A possible explanation for the difference in the MEi is that gas adsorption on the membrane wall was influenced by properties of molecular species that affect mass transfer in the microporous layer (i.e., diffusion coefficient, chemical affinity or reaction with the material, tortuosity). A theoretical equation to describe the behavior of cylindrical counter flow denuder has not yet been developed for innumerable gaseous species, and is beyond the scope of this article.
In conclusion, the gas removal process is based on diffusion through a microporous glass tube into a counter-directed purge gas flow. Pore size does not impact removal of gases under optimized flow rate conditions. However, the gas adsorption on the membrane wall depends on molecular species, pore size, and membrane materials.
3.4. Denuder particle loss in laboratory performance evaluation
The performance of the three denuders was tested using artificially generated PSL particles to determine particle losses.
Traditional theory of particle penetration Pi (Hinds Citation1999), in a single cylindrical tube with a particle diameter i (μm), was calculated using Equation (Equation5[5] ):
[5]
where ni,out and ni,in are number concentration of particle with diameter i (μm), #/cm3, at outlet and inlet of the denuder; μ was calculated using Equation (Equation6[6] ):
[6]
where D (cm2/s) is the diffusion coefficient of the particle with diameter i (μm), length of the inner tube L (cm), and volume flow rate through the inner tube Q (L/min).
shows the experimental measurements (shown as solid circles) and theoretical estimation by diffusion losses calculated using Equation (Equation2[2] ) (shown as dashed lines) for particle penetration. High particle penetration (>94% penetration for 20 nm) was observed for PSL particles larger than 20 nm and pore size (0.1 μm and 0.05 μm) did not impact penetration of the particles significantly. Since the particle penetration was similar to the theoretical loss curve shown in , I infer that the particle loss in the counter flow denuder could be explained by diffusion loss.
Figure 5. Particle penetration through different counter flow denuders. Averaged particle number concentrations at denuder inlet: 379 #/cm3 for 0.309 μm, 1111 #/cm3 for 0.202 μm, 3641 #/cm3 for 0.107 μm, 5489 #/cm3 for 0.07 μm, 3413 #/cm3 for 0.048 μm, 1378 #/cm3 for 0.02 μm. Experimental conditions: Qs = 0.15 L/min, Qp = 3 L/min.
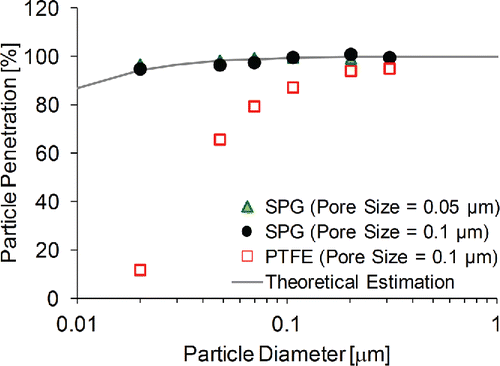
Previous study reported that particle penetration was much lower than theoretical estimation (<75% penetration for 20 nm) because the nonconductive membrane (PTFE) may have caused particle losses (Ruiz et al. 2006). This study found that when PTFE microporous tube was used instead of SPG in the counter flow denuder (Section 2.2), particle penetration efficiency was significantly low (<2% penetration for 20 nm of PSL, shown as Solid Square in ). These experimental results reaffirm the importance of microporous membrane material in counter flow denuder technique (Ruiz et al. 2006).
Future studies can use the high particle penetration counter flow denuder for aerosol preparations without co-existing compounds enabling simultaneous gas removal and different gas medium exchange (e.g., He, N2, and Ar). For example, the aerosol mass spectrometry without interference gases can be used the counter flow denuder for more accurate determination of H2O+, CO2+, and CO+ signals from oxygenated organic species sampled in air.
UAST_1271939_Supplemental_File.zip
Download Zip (59.1 KB)Acknowledgments
The author would like to thank the editor and reviewers for their careful perusal of this manuscript and their constructive comments.
Funding
This research was partially supported by the Environment Research and Technology Development Fund (5-1605) of the Ministry of the Environment, Japan.
References
- Ahlm, L., Liu, S., Day, D. A., Russell, L. M., Weber, R., Gentner, D. R., Goldstein, A. H., DiGangi, J. P., Henry, S. B., Keutsch, F. N., VandenBoer, T. C., Markovic, M. Z., Murphy, J. G., Ren, X., and Scheller, S. (2012). Formation and Growth of Ultrafine Particles From Secondary Sources in Bakersfield, California. J. Geophys. Res. D, 117:D00V08. doi:10.1029/2011JD017144
- Ashok, V., and Gupta, T. (2012). Evaluation of a Newly Developed Diffusion Denuder for Atmospheric Aerosol Separation from Co-Pollutant Gases. Sci. Total Environ., 439:150–157.
- Canagaratna, M. R., Jayne, J. T., Jimenez, J. L., Allan, J. D., Alfarra, M. R., Zhang, Q., Onasch, T. B., Drewnick, F., Coe, H., Middlebrook, A., Delia, A., Williams, L. R., Trimborn, A. M., Northway, M. J., DeCarlo, P. F., Kolb, C. E., Davidovits, P., and Worsnop, D. R. (2007). Chemical and Microphysical Characterization of Ambient Aerosols with the Aerodyne Aerosol Mass Spectrometer. Mass Spectrom. Rev., 26:185–222.
- Canagaratna, M. R., Jimenez, J. L., Kroll, J. H., Chen, Q., Kessler, S. H., Massoli, P., Hildebrandt L., Fortner, R. E., Williams, L. R., Wilson, K. R., Surratt, J. D., Donahue, N. M., Jayne, J. T., and Worsnop, D. R. (2015). Elemental Ratio Measurements of Organic Compounds Using Aerosol Mass Spectrometry: Characterization, Improved Calibration, and Implications. Atmos. Chem. Phys., 15:253–272.
- Collier, S., and Zhang, Q. (2013). Gas-Phase CO2 Subtraction for Improved Measurements of the Organic Aerosol Mass Concentration and Oxidation Degree by an Aerosol Mass Spectrometer. Environ. Sci. Technol., 47:14324–14331.
- de Gouw, J., and Warneke, C. (2007). Measurements of Volatile Organic Compounds in the Earth's Atmosphere Using Proton-Transfer Reaction Mass Spectrometry. Mass Spectrom. Rev., 26:223–257.
- Drewnick, F., Schwab, J. J., Jayne, J. T., Canagaratna, M., Worsnop, D. R., and Demerjian, K. L. (2004). Measurement of Ambient Aerosol Composition During the PMTACS-NY 2001 Using an Aerosol Mass Spectrometer. Part I: Mass Concentrations. Aerosol Sci. Technol., 38:92–103.
- Gormley, P. G., and Kennedy, M. (1949). Diffusion from a Stream Flowing Through a Cylindrical Tube. Proc. R. Irish Acad., 52:163–196.
- Hinds, W. C. (1999). Aerosol Technology. 2nd ed. John Wiley and Sons, Inc., New York.
- Hogrefe, O., Drewnick, F., Lala, G. G., Schwab, J. J., and Demerjian, K. L. (2004). Development, Operation and Applications of an Aerosol Generation, Calibration and Research Facility. Aerosol Sci. Technol., 38:196–214.
- Kitto, A.-M. N., and Colbeck, I. (1999). Filtration and Denuder Sampling Techniques, in Analytical Chemistry of Aerosol, K. R. Spurmy, and K. Willeke, eds., CRC Press, Boca Raton, FL, pp. 103–126.
- Lane, D. A. (1999). Gas and Particle Phase Measurements of Atmospheric Organic Compounds. CRC Press, Boca Raton, FL.
- Nilsson, P. K., Eriksson, A. C., Ludvigsson, L., Messing, M. E., Nordin, E. Z., Gudmundsson, A., Meuller, B. O., Deppert, K., Fortner, B. C., Onasch, T. B., and Pagels, J. H. (2015). In-Situ Characterization of metal Nanoparticles and Their Organic Coatings using Laser-Vaporization Aerosol Mass Spectrometry. Nano Res., 8:3780–3795.
- Nishiguchi, K., Utania, K., and Fujimori, E. (2008). Real-Time Multi-Element Monitoring of Airborne Particulate Matter using ICP-MS Instrument Equipped with Gas Converter Apparatus. J. Anal. Atom. Spectrom., 23:1125–1129.
- Ruiz, P. A., Lawrence, J. E., Ferguson, S. T., Wolfson, J. M., and Koutrakis, P. (2006). A Counter-Current Parallel-Plate Membrane Denuder for the Non-Specific Removal of Trace Gases. Environ. Sci. Technol., 40:5058–5063.
- Tabersky, D., Nishiguchi, K., Utani, K., Ohata, M., Dietiker, R., Fricker, M. B., Maddalena, I. M., Kocha, J., and Gunther, D. (2013). Aerosol Entrainment and a Large-Capacity Gas Exchange Device (Q-GED) for Laser Ablation Inductively Coupled Plasma Mass Spectrometry in Atmospheric Pressure Air. J. Anal. Atom. Spectrom., 28:831–842.
- Takegawa, N., Miyazaki, Y., Kondo, Y., Komazaki, Y., Miyakawa, T., Jimenez, J. L., Jayne, J. T., Worsnop, D. R., Allan, J. D., and Weber, R. J. (2005). Characterization of an Aerodyne Aerosol Mass Spectrometer (AMS): Intercomparison with Other Aerosol Instruments. Aerosol Sci. Technol., 39:760–770.
- Vladisavljevića, G. T., Shimizu, M., and Nakashima, T. (2005). Permeability of Hydrophilic and Hydrophobic Shirasu-Porous-Glass (SPG) Membranes to Pure Liquids and Its Microstructure. J. Membr. Sci., 250:69–77.
- Yamazaki, N., Yuyama, H., Nagai, M., Ma, G.-H., and Omi, S. (2002). A Comparison of Membrane Emulsification Obtained Using SPG (Shirasu Porous Glass) and PTFE [Poly(Tetrafluoroethylene)] Membranes. J. Dispersion Sci. Technol., 23:279–292.
- Ye, Y., Tsai, C.-J., Pui, D. Y. H., and Lewis, C. W. (1991). Particle Transmission Characteristics of an Annular Denuder Ambient Sampling System. Aerosol Sci. Technol., 14:102–111.