ABSTRACT
Laser-induced incandescence (LII) measurements were conducted to explore the ability of LII to detect small soot particles of less than 10 nm in two sooting flat premixed flames of n-butane: a so-called nucleation flame obtained at a threshold equivalence ratio Φ = 1.75, in which the incipient soot particles undergo only minor soot surface growth along the flame, and a more sooting flame at Φ = 1.95. Size measurements were obtained by modeling the time-resolved LII signals detected using 1064 nm laser excitation. Spectrally-resolved LII signals collected in the nucleation flame display a similar blackbody-like behavior as mature soot. Soot particle temperature was determined from spectrally-resolved detection. LII modeling was conducted using parameters either relevant to those of mature soot or derived from fitting the modeled results to the experimental LII data. Particle size measurements were also carried out using (1) ex situ analysis by helium-ion microscopy (HIM) of particles sampled thermophoretically and (2) online size distribution analysis of microprobe-sampled particles using a 1 nm-SMPS. The size distributions of the incipient soot particles, found in the nucleation flame and in the early soot region of the Φ = 1.95 flame, derived from time-resolved LII signals are in good agreement with HIM and 1 nm-SMPS measurements and are in the range of 2–4 nm. The thermal and optical properties of incipient soot were found to be not radically different from those of mature soot commonly used in LII modeling. This explains the ability of incipient soot particles to produce continuous thermal emissions in the visible spectrum. This study demonstrates that LII is a promising in situ optical particle sizing technique that is capable of detecting incipient soot as small as about 2.5 nm and potentially 2 nm and resolving small changes in soot sizes below 10 nm.
© 2017 American Association for Aerosol Research
EDITOR:
1. Introduction
Emission of soot formed from incomplete combustion of fossil fuels, biofuels, and biomass is a serious concern due to its harmful impact on human health, environment, and its positive radiative forcing on climate. Gaining fundamental understanding of soot formation, particularly the nucleation step leading to the formation of the first soot nuclei (incipient soot particles), is critical to develop reliable predictive soot models to help the design of more efficient and cleaner combustion devices. Unfortunately, the mechanisms of gas-phase species to particle transformation and the subsequent particle mass and size growth in hydrocarbon flames are still poorly understood (Wang Citation2011). To adequately understand the soot nucleation and growth in flames, it is essential to have the experimental capabilities to follow the kinetics of newly formed incipient soot particles, which means at least to resolve both the evolution of the particle size and particle number density distributions. In addition to size and number density, it is also highly desirable to probe the particle chemical composition and micro-structures, which are even more challenging (Wang Citation2011; Desgroux et al. Citation2013; Skeen et al. Citation2013; Michelsen Citation2017).
A detailed understanding of soot inception has been hindered by the difficulties of measuring the size evolution of incipient soot particles accurately, which are typically only a few nanometers in diameter (Dobbins and Subramaniasivam Citation1994; D'Alessio et al. Citation2000; Thierley et al. Citation2007; Zhao et al. Citation2007). Accurate measurements of particles smaller than 10 nm remain a challenge (Zhao et al. Citation2007; Zhao et al. Citation2003; Abid et al. Citation2008; D'Anna Citation2009; Schenk et al. Citation2013). Available experimental techniques for measuring soot particle size and concentration have been discussed by Wang (Citation2011), Desgroux et al. (Citation2013), Michelsen (Citation2016), and D'Anna (Citation2009). A series of experimental investigations of the particle size distribution function (PSDF) of soot particles formed in rich premixed ethylene flames have recently been conducted by Wang and co-workers (Zhao et al. Citation2007; Zhao et al. Citation2003; Abid et al. Citation2008; Camacho et al. Citation2015; Gu et al. Citation2016), Maricq et al. (Citation2003), and Stirn et al. (Citation2009). These studies first rely on the online sampling of the particles by a tubular probe placed transversally to the flame embedded or not in the stabilization plate or by a microprobe inserted vertically along the flame axis. Then the particles are size-selected according to their electrical mobility using a scanning mobility particle sizer (SMPS) to follow the evolution of PSDF of incipient soot particles formed in flat premixed flames, which can be considered as pseudo one-dimensional along the flame centerline. These systematic studies have consistently demonstrated that PSDFs at different heights are in general bimodal, except at fairly low equivalence ratios or at very low heights close to the burner surface where the PSDF is typically single-peaked.
The technique of thermophoretic sampling followed by transmission electron microscopy (TEM) image analysis has been widely used to study the size and morphology of flame-generated soot with success after the pioneering work of Dobbins and Megaridis (Citation1987). However, this technique is not suitable to investigate the size of newly formed soot particles for the following reasons. First, these particles may be liquid-like at the very early stage of inception and could tend to splash upon deposition on the TEM grids (Zhao et al. Citation2007; Abid et al. Citation2008). Second, the TEM images of these nascent particles have poor contrast and the particle structure is prone to be damaged by the electron beam (Zhao et al. Citation2007; Schenk et al. Citation2013). Due to these limitations, the smallest soot particles measured by the TEM approach are typically about 10 nm (Zhao et al. Citation2007; Bladh et al. Citation2011). Only a few studies using TEM have reported particles as small as 5 nm (Simonsson et al. Citation2017; Zhang et al. Citation2017).
Recently, incipient particles with structures as small as 2 nm could be detected by helium-ion microscopy (HIM), a technique which involves much lower beam-sample interaction than TEM (Schenk et al. Citation2013). Therefore, HIM seems a reliable alternative to TEM to image the size and morphology of incipient soot particles (Schenk et al. Citation2013). Direct comparisons of PSDFs of incipient soot obtained by HIM and SMPS in ethylene-oxygen-argon premixed flames showed that there is generally good agreement in the results of the two techniques at higher distances from the burner exit, where soot particles become more mature and larger (Schenk et al. Citation2013, Citation2015). However, large discrepancies are typically observed for newly formed incipient soot particles at low distances from the burner exit. The potential causes for the discrepancies were attributed to the sample spread on the HIM substrate and lower sampling efficiency for very small particles of few nanometers (Schenk et al. Citation2013, Citation2015). Very recently, Tang et al. (Citation2016) have demonstrated that incipient soot particles as small as about 1.5 nm can be measured with a diethylene glycol (DEG) SMPS. It is noticed that Sgro et al. (Citation2007, Citation2009) and D'Anna et al. (Citation2005) also measured particles with sizes below 2 nm in rich premixed and diffusion flames; however, they considered these particles as a new category of flame-generated nanostructure, namely nanoparticles of organic carbon (NOC), rather than newly formed incipient soot particles.
It is important to point out that both SMPS and HIM techniques are ex situ in nature because they rely on the insertion of a physical probe into the flame to extract the particle samples. The effects of probe sampling on soot distribution measured in burner-stabilized stagnation flames by SMPS have been recently discussed and numerically investigated (Saggese et al. Citation2016). It was found that the effects of probe sampling may slightly alter the bimodality of the soot size distribution and may lead to a small shift of about 1–2 mm from the stagnation surface. Although the influences of thermophoretic sampling on the distribution of very small particles, such as those detected by HIM, in premixed flames are unknown, they are expected to be similar to those found in TEM experiments.
The uncertainties in PSDF of incipient soot caused by the intrusiveness of probe sampling in both the HIM and SMPS techniques motivate further investigation of incipient soot particles using laser diagnostics. Laser-based techniques to diagnose incipient soot particles seem attractive, since these methods are non-intrusive, albeit they suffer from the uncertainties in the required optical properties and the potential interference to interpret the detected signals. Laser diagnostics of incipient soot particles also offer the potential to reconcile the discrepancies in PSDF between the results of HIM and SMPS (Schenk et al. Citation2013, Citation2015). Although laser scattering has often been used to probe the morphology of mature soot (Hu et al. Citation2003; Sorensen Citation2001), its application to incipient soot particles is challenging. Nevertheless, signals could be detected in the nucleation region of a laminar coflow diffusion flame by D'Anna et al. (Citation2005), from which mean particle diameters of about 3 to 4 nm were determined. The particles detected by D'Alessio and co-workers were referred as NOC (Sgro et al. Citation2007, Citation2009; D'Anna et al. Citation2005). Besides the needs to correct the scattering signals due to large gas-phase compounds (D'Anna Citation2009), the scattering signals tend to be dominated by the presence of a few large particles, e.g., aggregates (Zhao et al. Citation2003). Other non-intrusive techniques for particle size measurements have been discussed in the recent review by Michelsen (Citation2016), including wide-angle X-ray scattering (WAXS) and laser-induced incandescence (LII), among others.
A remaining question concerns the nature of the particles that are optically detected in flames, i.e., if the newly formed incipient nanoparticles are capable of emitting and absorbing thermal radiation in the visible and near-infrared spectrum. In the present work we define a soot particle as a carbon-containing entity that is able to radiate continuous blackbody-type radiation and can be detected in flames in the visible spectral range without or with external radiation heating. Despite the general belief that incipient soot particles of few nanometers in diameter do not produce LII signals under the excitation of a laser pulse (Michelsen Citation2017), three recent experimental studies of soot formation in low-sooting burner-stabilized premixed flames (Mouton et al. Citation2013; Bladh et al. Citation2015; Faccinetto et al. Citation2017) have demonstrated that LII signals can be produced by incipient soot particles and detected using LII in the so-called “nucleation flames.” The equivalence ratio of those particular flames is such that the mixture is just rich enough to produce incipient soot particles and these particles undergo very minor surface growth when they are transported downstream. A nucleation flame is therefore barely able to display a pale red sooting zone due to thermal emissions from the incipient soot particles. Below or above this critical narrow range of equivalence ratio, the flame is either blue or markedly sooting, respectively. The decay rate of time-resolved LII signals of incipient soot particles in these nucleation flames seems still to bear size information of such newly formed soot particles (Bladh et al. Citation2015), as expected from the principle of particle sizing using LII: a smaller particle cools faster than a larger one (Will et al. Citation1995; Schultz et al. Citation2006). The first attempt was made by Bladh et al. (Citation2015) to use a LII model to fit the predicted time-resolved LII signal to the experimentally measured one with the aim to derive the diameter of soot particles produced in the nucleation flames. They showed that these nucleation particles are about 1 to 2 nm and thus very close to the size of soot particles detected in the nucleation region of premixed flames using HIM and SMPS (Schenk et al. Citation2013, Citation2015; Tang et al. Citation2017). The consistency of these results is considered encouraging given the uncertainties in the thermal and optical properties of newly formed incipient particles.
In this work, we have now combined a comprehensive arsenal of techniques in a further experimental study to probe incipient soot formed in n-butane flat premixed flames at and slightly above the critical equivalence ratio at atmospheric pressure. LII modeling was carried out to derive the incipient soot particle size distribution based on the experimentally detected time-resolved LII signals at two wavelengths. Model parameters were either chosen based on best available knowledge in the literature or determined by fitting the model results to the LII data obtained at a particular set of conditions. To validate and compare the particle size derived using LII, complementary experiments were also performed using 1nm-SMPS and HIM to provide independent particle size distributions. To confirm that the detected signals are indeed attributable to LII, i.e., the continuous emissions from the laser-heated soot particles in the nucleation flame, spectrally resolved detection in the visible spectrum was also performed. The objectives of this study are (1) to explore the potential of using LII for measuring PSDF of incipient soot, (2) to validate the soot particle size derived from LII using the results of HIM and 1nm-SMPS, (3) to gain improved understanding of the thermal and radiative properties of incipient soot, and (4) to unambiguously establish that the time-resolved signals detected at 532 and 650 nm and the spectrally resolved signals detected in the visible spectrum are due to thermal emissions from the laser heated incipient soot particles.
2. Experimental work
2.1. Burner and flames conditions
Two n-butane flames stabilized on a commercial McKenna burner (Holthuis) at atmospheric pressure have been investigated: a standard sooting flame at ф = 1.95 (Flame1.95) and the so-called nucleation flame at ф = 1.75 (Flame1.75).
The burner has a central bronze porous plug (60 mm diameter). It is surrounded by a co-annular porous to protect the flame from external perturbation and to prevent the formation of a diffusion flame between the unburnt fuel and the surrounding air. A stainless steel disk (60 mm diameter, 30 mm thick) was placed at 16 mm above the burner surface. The disk is perforated at its center for introducing axially a sampling probe used for SMPS measurements. The probe can be vertically translated between the burner surface and the disk. A water cooling circuit allows keeping the burner temperature at 70°C during experiments. The burner can be translated vertically with a spatial resolution better than 50 µm.
The gas flow rate of oxygen/nitrogen/n-butane mixture was 6.71 SLPM (standard liter per minute) and the nitrogen flow rate was 55% of the total flow rate. The shielding co-flow of nitrogen was 20 SLPM. Both flames studied display a yellow/orange luminous color appearance, characteristics of blackbody emission at flame temperature as shown in , though the luminosity of the nucleation flame due to particle emissions above the blue zone is quite faint, . It must be noticed that the faint red-color appearance could still be observed with naked eyes down to an equivalence ratio of 1.70; however, this flame, i.e., ф = 1.70, was not selected for measurements because of the very low signal-to-noise ratio observed in test LII experiments.
2.2. Centerline gas temperature distribution by NO-LIF thermometry
Among the species used for thermometry, NO is a good candidate because this molecule can be seeded as a tracer in the reactive mixture, allowing the measurement of the complete temperature profile from the burner surface to the burnt gases (Hartlieb et al. Citation2000). In addition, the capability of multi-line laser-induced fluorescence thermometry on NO species (NO-LIF thermometry) has also been demonstrated to measure the temperature of sooting flames (Bejaoui et al. Citation2015; Bessler and Schulz Citation2004; Denisov et al. Citation2014).
In this work, temperature profiles along the flame centerline have been obtained by NO-LIF thermometry. Since this method was described in detail in a previous paper (Bejaoui et al. Citation2015), the details of measurements are provided in the online supplementary information (SI).
2.3. LII setup: Time-resolved LII and spectrally-resolved LII measurements
2.3.1 Optical set-up
A schematic of the LII experimental setup is provided in . LII experiments have been carried out by using a 1064 nm laser excitation wavelength generated by a Nd:YAG laser (Quantel Brilliant) at 10 Hz with a FWHM pulse duration of 9 ns. The laser temporal profile is provided in the SI. This wavelength is the most appropriate to avoid spectral interferences with PAHs absorption transitions. The excitation system was improved to maximize the signal-to-noise ratio by setting a large collection volume in which the soot distribution can be considered as constant. We took advantage of the flatness characteristics of the premixed flame at a given height above the burner (HAB) to expand the collection volume in a plane parallel to the burner surface (x,y). First, the laser beam was expanded in a horizontal plane using cylindrical lenses (f1 = −40 mm, f2 = 200 mm) and then passed through a rectangular slit (0.48 mm z axis and 7.23 mm x axis) relay imaged on the burner axis by means of a lens (f3 = 300 mm) (). The homogeneity of the top-hat beam was monitored with a beam profiler (Gentec Beamage). The fairly top-hat fluence distribution ensures to heat all the particles with nearly the same laser fluence, which was varied between 0 and 4 mJ/mm2.
The collection volume was selected by imaging the collection slit (0.3 mm z axis and 5.6 mm y axis; ) into the top-hat laser beam with two achromatic lenses (f4 = 250 mm, f5 = 200 mm).
2.3.2. Time-resolved measurements
The time-resolved LII signals at two wavelengths (532 and 650 nm) were measured at different HABs along the centerline of the two flames to infer the soot size evolution from LII modeling. LII issued from the collection volume was split with a dichroic mirror (Semrock FF596-DI01) and detected simultaneously by two photomultipliers tubes (PMT) (Hamamatsu R2257), which have a rise time of 2.6 ns and were equipped with interference filters centered respectively at 532 nm with FWHM 8.6 nm and at 650 nm with FWHM 10 nm. A chopper (Oriel Electronic Shutter 76994) placed in front of the collection system with an opening time of 20 ms and a repetition rate of 10 Hz was used to reduce the continuous light emission from the flame on the PMTs. This partial rejection of the flame emission improved significantly the signal-to-noise ratio. A delay generator (Stanford DG535) allowed the synchronization of the chopper aperture with LII signals. The LII signals were recorded by an oscilloscope (LECROY 6050A, 8 bit, 500 MHz bandwidth, 5 GS/s sampling rate), which was triggered by a photodiode (Hamamatsu S1722-02) measuring the transmitted laser radiation and placed after the burner. The time-resolved LII signals were averaged over at least 2000 laser shots to improve signal-to-noise ratio. The calibration of the detection system and the bandpass filters was performed using an integrating sphere (SphereOptics CSTM-LR-6-M) which emits calibrated blackbody radiations for different temperatures.
2.3.3. Spectrally-resolved LII measurements
Two achromatic lenses (f7 = 400 mm, f8 = 200 mm) were used to image the LII signals onto the spectrograph slit (0.1 mm z axis and 5 mm y axis). LII spectra were recorded with an imaging spectrograph (Acton SP 2300) with a 50-grooves/mm 600-nm blazed grating and a gated ICCD camera (Princeton PiMAX Gen III). The camera was triggered by the Q-switch output of the laser. The exposure time was fixed to 10 ns starting with the peak of the laser. The spectrograph wavelength calibration was achieved using the spectral lines of mercury (Oriel 6035) and the integrating sphere. All the spectra presented in this article were corrected for background and spectrally calibrated.
2.4. HIM
For the HIM detection in Bielefeld, samples were collected thermophoretically (Li and Wang Citation2004) by insertion of a silicon wafer into the flame. The silicon wafer was connected to a stepper motor allowing a repetitive swing movement across a horizontal plane parallel to the burner surface. The spatial resolution along the flame height direction is estimated to ± 0.5 mm. The mean insertion velocity was 0.1 m/s. The total time that the wafer was inserted inside the flame was optimized for each flame and HAB by changing the number of sweeps. The samples were collected at 3.5, 6 and 10 mm in Flame1.95. In Flame1.75, the soot concentration was very low and the sampling was taken at 12 mm only. The collected samples were analyzed by a helium-ion microscope (Carl Zeiss Orion Plus) (Hlawacek and Gölzhäuser Citation2016). This microscope focuses helium ions onto the specimen and scans the beam over the sample. Similar to a scanning electron microscope (SEM), secondary electrons are recorded to generate an image that offers higher resolution, contrast and surface sensitivity compared to an SEM (Ward et al. Citation2006). The images were recorded at a beam energy of 35 keV and a beam current of 0.4 pA.
The HIM images were processed using the free software ImageJ (https://imagej.nih.gov/ij/docs/guide/146-30.html#fig:Display-options-Particle-Analyzer, 2016). A similar procedure, as reported by Schenk et al. (Citation2015), was used to process the images. Examples of the image processing are available in the SI. In particular, a manual and an automatic method were used for particle size determination. Examples of the image processing, as well as information on the above-mentioned methods for particle size determination, are available in the SI. In all cases, the size of individual primary particles was measured using the tools available in the software. Even though small aggregates could be identified at high HAB, we did not include them in the present study because of the insufficient contrast to extract the primary particles from them. Thus only the recognizable primary particles were measured. This is consistent with the current LII analysis which is limited to primary soot particles that have not undergone aggregation.
2.5. Electrical mobility sizing of soot nanoparticles
2.5.1. Probe system for soot size distribution
In order to extract soot and combustion gases from the nucleation flame we developed a probe sampling system featuring high dilution ratio to quench post-sampling chemical reactions and to avoid nanoparticle coagulation and aggregation. This flame extraction system used in Lille consists of a sampling microprobe coupled to an automatic pressure regulation system to achieve a high dilution ratio while minimizing flame perturbations. The microprobe is made of two co-annular quartz tubes. The outer tube ends with a long thin tip with a 100 µm orifice. A nitrogen dilution flow (2–20 L min−1) enters the microprobe through the side port and flows between the two quartz tubes up to the probe tip. A sample flow from the flame enters the microprobe tip orifice drawn in by the room-probe pressure difference. The sample flow is immediately diluted by the cold nitrogen dilution flow, and both of them are removed into the pumping flow through the inner tube. A buffer volume located downstream the microprobe increases the overall particle concentration stability. The automatic pressure regulation system is located further downstream the buffer volume. The automatic regulation valve (Pfeiffer EVR 116) uses a proportional–integral–derivative (PID) feedback to maintain the pressure in the sampling line within 0.5 mbar from the setpoint. Finally, the buffer volume is connected through a third independent outlet to the SMPS system, so that only part of the pumping flow (0.3 or 1.5 L min−1) is sampled during online analyses. Conductive silicone tubing (around 40 cm) is used to this purpose. The pressure differential Δp between the sampling line (thus the microprobe) and the room (thus the flame) is typically set in the range 5–50 mbar depending on the current experiment. The larger the Δp the more efficient the extraction from the flame (soot and gas concentration in the sampling line increase), but also the larger the particle aggregation and vapor condensation rates in parallel. The dilution ratio as a function of the Δp and of the dilution flow was calibrated with a reference aerosol of ammonium sulfate. The PSDFs measured using 1 nm-SMPS in this work have been obtained with a dilution ratio estimated as 3000 ± 20%, and allowing satisfying signal-to-noise ratio.
2.5.2. SMPS methodology
Scanning Mobility Particle Sizer (SMPS) spectrometers employ a differential mobility analyzer (DMA) for size classification in the sub-micrometer range and combine it with a detector with high efficiency for small particles. The technique uses the electrical mobility concept (Wang and Flagan Citation1990; Baron and Willeke Citation2005) to separate particles with a known charge suspended in a gas. SMPS spectrometer sizing is a discreet technique in which number concentrations are measured directly without assuming the shape of the particle size distribution. The method has a high degree of absolute sizing accuracy and measurement repeatability. Tröstl et al. (Citation2015) provided details on the Nano-SMPS and its performance. Condensation Particle Counter (CPC) has been widely used as detector for the measurement of aerosol particle size distributions.
In this work we have used a new 1nm-SMPS system (Model 3938E77, TSI Inc., Shoreview, MN, USA) capable of measuring size distributions from 1 to 50 nm. It provides a high-resolution method to do fast measurements of particle size distributions starting from 1 nm (D50 at 1.1 nm geometric diameter or 1.4 nm electrical mobility diameter). This lower cut-off diameter is achieved by using the Nano Enhancer (Model 3777, TSI Inc.) to activate particles using Diethylene glycol (DEG) before entering the butanol based CPC (Model 3772, TSI Inc.). DEG has been used as a working fluid to pre-grow sub-2.5 nm particles to a size detectable with a standard CPC (Iida et al. Citation2009; Jiang et al. Citation2011). The Electrostatic Classifier of SMPS is equipped with a soft-x-ray neutralizer and the new 1nm-DMA (Model 3086, TSI Inc.) which has a similar design to the Nano-DMA but was further optimized for size-selecting particles down to 1 nm with lower diffusion losses. The aerosol inlet flow of the 1nm-SMPS from the probe system was 2.5 L min−1 and the component setup of the 1 nm-SMPS was optimized for minimal distance to reduce diffusion losses.
3. LII modeling
3.1. LII model
To simulate the thermal response of incipient particles under the excitation of a laser pulse of nano-second duration, the temporal evolution of soot temperature and primary particle diameter is calculated using an LII model consisting of the unsteady energy and mass conservation equations of a primary particle. Particle aggregation is neglected under the present conditions. The LII model used in this study takes into accounts only the commonly accepted heat and mass transfer processes, i.e., laser energy absorption, soot particle internal energy change, heat conduction, and thermal sublimation. Thermal radiation from soot particles contributes negligibly to particle heat loss at atmospheric pressure and is not included in the energy equation. The model formulation has been described in several previous studies (Smallwood et al. Citation2001; Liu et al. Citation2006; Michelsen et al. Citation2007); therefore, only a brief summary is given here. The unsteady energy and mass conservation equations written for a primary particle can be expressed as[1]
[2] where dp is the primary particle diameter, ρs and cs are the density and specific heat of soot, t is time, T is soot temperature, Cabs is the absorption cross-section of the primary particle and is evaluated using the Rayleigh regime expression, F is the laser fluence, q(t) is the normalized laser power temporal profile, qc is the heat loss rate due to conduction. The last term in Equation (Equation1
[1] ) represents the sublimation heat loss rate with M being the mass of primary particle, Mv the mean molecular weight of the sublimated species, and ΔHv the heat of sublimation. Quantity pv in Equation (Equation2
[2] ) is the sublimation pressure (Smallwood et al. Citation2001), Ru is the universal gas constant (8.3145 J/mol K), and β is the effective sublimation coefficient, which is also called the mass accommodation coefficient (Michelsen et al. Citation2007). Under the flame conditions of the present study, heat conduction between a soot particle and the surrounding gas occurs in the free-molecular regime. Therefore, the soot conduction heat loss rate, qc, can be calculated using the free-molecular expression (Filippov and Rosner Citation2000)
[3] where α is the thermal accommodation coefficient, pg is the ambient pressure (1 atm in this study), kB is the Boltzmann constant, mg is the ambient gas molecule mass, Tg and γ* are the local gas temperature and the averaged specific heat ratio, respectively (Filippov and Rosner Citation2000).
The incipient and young soot particles are typically only few nanometers in diameter (Schenk et al. Citation2013, Citation2015) and the corresponding size parameters (πdp/λ) in the visible spectrum are small enough to use the Rayleigh regime approximation to evaluate the absorption cross-section of primary particle expressed as[4]
The value of absorption function E(m) for mature soot in the visible and near-infrared is about 0.3 to 0.4 (Snelling et al. Citation2004; Bladh et al. Citation2011; Bejaoui et al. Citation2015). For incipient soot, however, the value of E(m) is likely somewhat lower based on recent studies (Bladh et al. Citation2011; Bejaoui et al. Citation2015) and likely dependent on the degree of soot maturity (López-Yglesias et al. Citation2014; Simonsson et al. Citation2015), which cannot be easily quantified. Nevertheless, there are considerable uncertainties in the thermal (density, specific heat, vapor pressure, enthalpy of vaporization/sublimation) and optical (refractive index, or the absorption function in the context of LII) properties of incipient soot particles.
Particle aggregation was neglected in this study, since the model was used only in the region of the flames where the aggregation is negligible based on the HIM images described later. The primary particle size distribution was taken into account by assuming that it follows the log-normal distribution given as[5] where dg and σg are the geometric mean primary particle diameter and the geometric standard deviation of dp, respectively.
After correcting for the detection system specific characteristics, such as the filter bandwidth, PMT gain, and PMT efficiency, the total LII signal at detection wavelength λ, contributed from all the soot particles in the laser beam cross-section viewed by the detection optics, can then be expressed as[6] where c is the speed of light in vacuum, h is the Planck constant, kB is the Stefan-Boltzmann constant, and Θ is a detection system constant, which accounts for the detection-wavelength-independent parameters, such as the laser beam width and signal collection solid angle, and cross-sectional area of the detection optics. To simulate the soot temperatures derived from the spectrally-resolved LII signals collected at the moment of the laser peak for 10 ns described in Section 2.3.3, it is necessary to define an effective soot temperature Teff as follows
[7] where τ1, which coincides with the peak of the laser pulse, and τ2 are the beginning and end of the ICCD exposure (τ2 = τ1 + 10 ns), λ1 and λ2 are chosen to be the same as those in the LII experiments, i.e., 532 and 650 nm, respectively. Substitution of Equation (Equation6
[6] ) into Equation (Equation7
[7] ) leads to
[8] It is noticed that the soot temperature in the integrals of Equation (Equation8
[8] ) is dependent on time t and the primary particle diameter dp at a given laser fluence F. Iteration is required to obtain Teff from Equation (Equation8
[8] ). However, an explicit solution can be found under the Wien approximation, i.e.,
. In this case, Teff can be expressed as
[9]
Using the Teff determined from Equation (Equation9[9] ) as the first guess, the accurate Teff satisfying Equation (Equation8
[8] ) can be obtained in typically only about 3 to 4 iterations using the iterative approach of Levendis et al. (Citation1992).
The measured laser pulse temporal profile (given in the SI), which has a duration of about 9 ns (FWHM), was used in the LII modeling. Further details of the LII model calculations can also be found in the SI.
3.2. Thermal and optical properties of incipient soot
The thermal and optical properties of incipient or in some studies also called ‘young’ soot are scarce or even unavailable in the literature. Even for mature soot, its properties are subject to large uncertainties (Michelsen et al. Citation2007). Some attention has been paid to the mass density of young soot in several recent studies (Zhao et al. Citation2007; Camacho et al. Citation2015; López-Yglesias et al. Citation2014; Totton et al. Citation2010). While Totton et al. (Citation2010) recommended a very low value of 1.12 g/cm3 for incipient soot density, a higher value of 1.5 g/cm3 was preferred by Zhao et al. (Citation2007) and Camacho et al. (Citation2015) for the density of newly formed incipient soot. It is noticed that the value of 1.5 g/cm3 seems more reasonable based on the consideration that the mass densities of soot precursors are around 1.3 g/cm3 (1.27 g/cm3 for pyrene and 1.35 g/cm3 for benzopyrene). To account for the effect of soot particle maturity on soot density López-Yglesias et al. (Citation2014) proposed a model in which the soot density varies linearly with soot maturity (characterized the degree of annealing) between 2.28 g/cm3 (for graphite) and 1.63 g/cm3 for fully un-annealed soot.
As shown in Equation (Equation1[1] ), the product of soot density and soot specific heat, ρscs, is required in the energy equation, rather than the soot density ρs itself. Unfortunately, there have been no studies to investigate the specific heat of incipient soot to our best knowledge, though it seems reasonable to assume that young soot has a somewhat lower specific heat than that of mature soot. As such, it is assumed in this study that the newly formed young soot has a constant mass density of 1.3 g/cm3 and resumes the same temperature-dependent specific heat of mature soot as in the Liu model given in Michelsen et al. (Citation2007).
The soot absorption function E(m) at the laser wavelength is one of the most important parameters in modeling LII, since it determines the laser energy absorption rate. Several efforts have been made to investigate the absorption function E(m) of aging soot in the visible and near infrared as a function of HAB in flat premixed flames (Bladh et al. Citation2011; Bejaoui et al. Citation2015; Maffi et al. Citation2011) and its wavelength dependence (López-Yglesias et al. Citation2014; Simonsson et al. Citation2015; Cléon et al. Citation2011; Migliorini et al. Citation2011). These studies have shown that the soot absorption increases with increasing HAB from about 0.2 to 0.4 as soot particles evolve from young to more mature state. In addition, E(m) of young soot low in the flames displays a strong wavelength dependence and it increases with decreasing wavelength (López-Yglesias et al. Citation2014; Simonsson et al. Citation2015; Migliorini et al. Citation2011). The spectral dependence of E(m) has a strong implication for the soot temperature inferred from 2C-LII: assuming a wavelength-independent E(m) in the visible leads to a higher soot temperature than assuming an increasing E(m) with decreasing wavelength. In an attempt to investigate the size dependence of E(m) at 1064 nm for growing carbon particles during acetylene pyrolysis, Eremin et al. obtained E(m) values as small as about 0.05 (Eremin et al. Citation2011). Caution should be taken regarding the E(m) values derived from these 2C-LII studies using the methodology of Snelling et al. (Citation2004), since the product ρscs of mature soot was used for young soot. Nevertheless, given the uncertainty in how the absorption function of young soot varies with wavelength in the visible spectrum, which is the subject of ongoing research, the E(m) of young soot is assumed as spectrally independent in the visible spectrum in this study.
The thermal accommodation coefficient (TAC) of soot is another important parameter in LII modeling, which affects the particle heat conduction cooling rate and has been the subject of several recent studies. Some consensus has been reached that the TAC of mature soot falls in the range of about 0.2 to 0.4 (Snelling et al. Citation2004; Maffi et al. Citation2011; Kuhlmann et al. Citation2006; Michelsen Citation2009). However, there have been no studies in the literature to investigate the TAC of incipient soot. The most relevant studies of incipient soot TAC are perhaps those in Bladh et al. (Citation2011), Bejaoui et al. (Citation2015), and Maffi et al. (Citation2011). In particular, Maffi et al. (Citation2011) obtained a TAC value of 0.22 at HAB = 10 mm in a flat premixed flame where the soot particles are 10 nm in diameter.
The thermal properties required to model the sublimation/vaporization of young soot, such as the vapor pressure, enthalpy of sublimation, the mean molecular weight of the sublimed species, and the effective sublimation coefficient, are essentially unavailable. The values of these properties commonly used for mature soot were summarized in the study of Michelsen et al. (Citation2007). In particular, the effective sublimation coefficient β is subject to a large uncertainty ranging from 0.1 for C3, which is the dominant sublimation species (Smallwood et al. Citation2001) in the Michelsen model (Michelsen et al. Citation2007) to as high as 0.9 in other models (Michelsen et al. Citation2007). Only a few recent studies have been conducted to gain some understanding of the sublimation processes of carbon nanoparticles (Olofsson et al. Citation2015; Eremin et al. Citation2013). Olofsson et al. (Citation2015) investigated the sublimation threshold temperature of soot at different heights of flat premixed flames using a combination technique of 2C-LII and elastic light scattering and modeled soot sublimation using properties of mature soot at all the heights considered. The potential size dependence of the sublimation process of carbon nanoparticles produced in the pyrolysis of 1% C6H6 in argon in a shock tube was explored by Eremin et al. (Citation2013) using a combination of 2C-LII and laser light extinction. Although these studies are useful to elucidate the weakness of the current sublimation sub-model along with the properties of mature soot, they offer little help to determine the properties of incipient soot in modeling the thermal sublimation process.
Given this status of our knowledge of the thermal and optical properties of incipient soot, most of the properties of mature soot used in the Liu model described in Michelsen et al. (Citation2007), such as the specific heat, mean molecular weight of the sublimated species, sublimation pressure, and sublimation enthalpy, except few properties discussed below, are used in this study due to a lack of better information. As it was pointed out earlier, the density of incipient soot was assumed constant at ρs = 1.3 g/cm3. The thermal accommodation α was estimated by matching the modeled effective soot temperature decay rate to that obtained from the experimentally determined time-resolved soot temperature based on the ratio of LII signals detected at 532 and 650 nm according to the principle of two-color pyrometry at HAB = 7 mm in Flame1.75. In doing so, the fitted lognormal distribution based on the measured soot particle size distribution by 1 nm-SMPS (dg = 3 nm and σg = 1.13) presented later and the measured gas temperature at HAB = 7 mm in Flame1.75 were used as input to the LII model. The estimated TAC is 0.35, which falls in the uncertainty range of the literature values (∼0.2 to 0.4) for mature soot, and is used throughout this study, i.e., at other heights in both Flame1.75 and Flame1.95. The absorption function E(m) at 1064 nm and the effective sublimation coefficient β of incipient soot are allowed to vary with HAB or with flame condition. They were determined by matching the experimentally derived average soot particle temperatures over 10 ns starting at the laser peak over a fairly wide range of laser fluences. This methodology is based on the fact that in the low laser fluence regime, where the peak soot temperature is below about 3400 to 3600 K (Liu et al. Citation2006; Olofsson et al. Citation2015), the average soot temperature is solely determined by E(m) for known ρscs, laser fluence, the local flame temperature, and an estimated primary particle diameter. At high laser fluences, where soot sublimation is significant, the average soot temperature over the 10 ns starting at the laser peak is sensitive to β under the known E(m) derived from the average soot temperature at low laser fluences.
4. Results and discussion: LII measurements and modeling
4.1. Spectrally-resolved LII in Flame1.95 and Flame1.75
The averaged soot particle temperature over 10 ns from the peak of the laser is determined by fitting the Planck function to the experimental LII spectra on a chosen wavelength range. In this study, we have fitted our experimental spectra between 450 nm and 800 nm. This determination is illustrated in case of young soot particles detected in Flame1.95 and in Flame1.75 for two laser fluences in . The natural emissions of the flames, i.e., blackbody radiation from both flames without the laser heating, are also plotted in the figure for comparison. The signal-to-noise ratio is found higher in Flame1.95 due to the higher number density of incipient soot. The C2 emission can be identified at high laser fluences (Goulay et al. Citation2010). When C2 emission is clearly visible, the spectral ranges considered for the fitting were reduced to two narrower spectral ranges, i.e., [450–500 nm] and [525–800 nm] to exclude the interference of C2 emission. Goulay et al. (Citation2013) noted that contributions to the spectra from second-order reflections from the grating lead to a temperature that is higher by 60 K at Ts = 4300 K and by a smaller difference at lower temperatures. This effect has not been considered in our analysis.
Figure 3. LII emission spectra collected over 10 ns starting at the peak of the laser: (a) Flame1.95 at HAB = 5 mm and (b) Flame1.75 at HAB = 10 mm. Symbols stand for experimental data at different laser fluences: black Δ, F = 3.5 mJ/mm2; grey x, F = 2.4 mJ/mm2; blue ◊ (right vertical axis), F = 0, i.e., natural flame emission without laser heating. Lines (red) represent the Planck function fit used to obtain Ts.
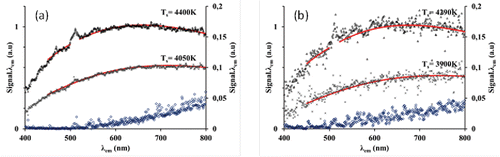
compares the spectrally-resolved emission spectra detected at HAB = 5 mm in Flame1.95 and at HAB = 10 mm in Flame1.75 for two different laser fluences. The spectrally-resolved emission signals shown in and are direct evidence that the incipient particles formed in rich premixed flames investigated in this study, with and without laser heating, are capable of producing continuous thermal emissions in the visible spectrum, i.e., LII signals. In addition, these results imply that the radiative properties of incipient soot in Flame1.95 and particles in Flame1.75 are similar.
Figure 4. Emission spectra collected for (a) F = 3.6 mJ/mm2 and (b) F = 1.3 mJ/mm2 at HAB = 5 mm in Flame1.95 (line [red]) and at HAB = 10 mm in Flame1.75 (symbols). The temperatures obtained by Planck function fit are (a) 4290 K in Flame1.75 and 4400 K in Flame1.95 and (b) 2950 K in both flames. The dashed line in (b) represents the 5-point moving average.
![Figure 4. Emission spectra collected for (a) F = 3.6 mJ/mm2 and (b) F = 1.3 mJ/mm2 at HAB = 5 mm in Flame1.95 (line [red]) and at HAB = 10 mm in Flame1.75 (symbols). The temperatures obtained by Planck function fit are (a) 4290 K in Flame1.75 and 4400 K in Flame1.95 and (b) 2950 K in both flames. The dashed line in (b) represents the 5-point moving average.](/cms/asset/f18bc37f-8319-4496-ba26-99842843685a/uast_a_1325440_f0004_oc.gif)
4.2. Soot particle temperatures
The LII model, along with the model parameters discussed above, was first used to estimate the geometric mean primary particle diameter dg while keeping the geometric standard deviation σg constant at 1.15 at HAB = 7 and 10 mm in Flame1.75 based on the experimentally measured time-resolved LII signal at 532 nm at a high laser fluence of 3.5 mJ/mm2. Although a technique has been proposed previously to obtain both dg and σg using time-resolved soot temperature (Liu et al. Citation2006), it cannot be applied in the present context due to the very rapid cooling of incipient soot particles of few nanometers. The consequence of assuming a constant σg will be discussed later. It was found that dg is approximately 2.5 to 3 nm at these heights. These primary particle size distributions were then used to determine E(m) and β following the approach discussed above. The measured local flame temperature was used as an input parameter to the LII model.
It is important to point out that the determination of E(m) and β based on the measured average soot temperatures requires the soot particle size distribution for incipient soot particles of few nanometers, which in turn is inferred from the time-resolved normalized LII signal. Therefore, there is a strong coupling between estimate of E(m) and β and the LII inferred particle size distribution. The results presented below were obtained through iteration to ensure that the inferred E(m), β, and the particle size distribution provide the overall good fit to both the average soot temperatures over 10 ns after the laser peak and over a wide range of laser fluence and the time-resolved normalized LII signals. The purpose of LII modeling in the present context is not to independently predict the LII behavior of incipient soot particles, but to retrieve the particle size distribution of incipient soot through multivariable optimization. The intimate coupling between E(m) and β on one hand and the particle size distribution on the other highlights the fact that it is necessary to measure both time-resolved LII signals and particle temperatures over a range of laser fluences in order to obtain the particle size distribution.
compares the modeled average soot temperature over 10 ns starting from the laser peak with those derived from LII experiments over a wide range of laser fluence in Flame1.75 at HAB = 7 and 10 mm. Fairly good agreement is observed between the modeled soot temperatures and those inferred from the Planck function fitting procedure over the spectral range of 450 to 800 nm over the whole range of laser fluence. This agreement is somewhat expected since the modeling results are based on the E(m) and β values derived from matching the measured soot temperatures at the low and high laser fluences, respectively. Nevertheless, the modeled soot temperatures at the intermediate laser fluences also agree well with the experimentally derived soot temperatures at both heights.
Figure 5. Comparison of the modeled average soot temperatures with experimentally derived ones over 10 ns after the laser peak at HAB = 7 and 10 mm in Flame1.75. The inferred soot absorption function and the effective sublimation coefficient are E(m) = 0.23, β = 0.04 at HAB = 7 mm and E(m) = 0.26, β = 0.02 at HAB = 10 mm. The geometric mean particle diameters are 2.5 and 3 nm at HAB = 7 and 10 mm, respectively.
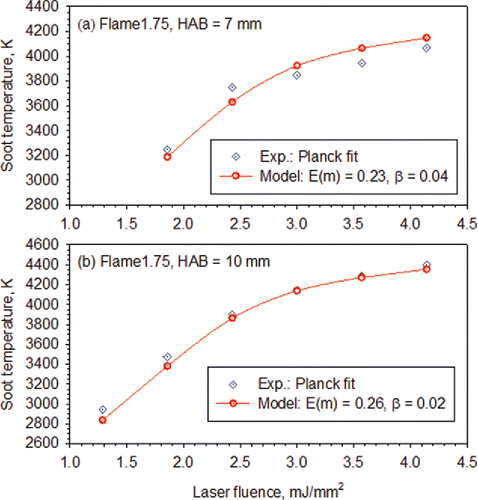
The derived values of E(m) and β, which are given in the figure legend, increase and decrease, respectively, somewhat with HAB. The HAB dependence of E(m) and β is likely an indication that the properties of soot still evolve even in this nucleation flame. The degree of increase in E(m) with HAB (from 0.23 at HAB = 7 mm to 0.26 HAB = 10 mm) is smaller than earlier studies (Bladh et al. Citation2011; Bejaoui et al. Citation2015), which can be attributed to the fact that there is weak soot surface growth and therefore only limited transition from incipient to mature soot in Flame1.75, as confirmed by SMPS measurements shown below. The decreased effective sublimation coefficient β with increasing HAB from 0.04 at HAB = 7 mm to 0.02 at HAB = 10 mm implies that the thermal sublimation rate of incipient is higher, or equivalently the threshold soot sublimation temperature is slightly lower, at a lower HAB. The lower threshold sublimation temperature at a lower HAB, which is manifested by a larger effective sublimation coefficient while keeping other sublimation parameters fixed, however, is in disagreement with the finding of Olofsson et al. (Citation2015), who arrived at a higher threshold sublimation temperature at a lower HAB when assuming wavelength-independent E(m) in the evaluation of soot temperatures from their two-color LII measurements. The cause of such disagreement remains unclear and deserves further investigations.
The average soot temperature over 10 ns after the laser peak has been measured using Planck function fitting over the spectral range of 450 to 800 nm at HAB = 5, 7, and 10 mm in Flame1.95 for a wide range of laser fluences. Modeling of the average soot temperature was performed only at HAB = 5 mm, since particle aggregation becomes significant at higher heights and the present model did not take into account this effect. The modeled average soot temperatures at HAB = 5 mm in Flame1.95 are compared with the measured values in . It can be seen that the agreement between the modeled and the measured average soot temperatures is fairly good, considering the uncertainty affecting the temperature measurements estimated to be about ±4%, though the model underpredicts soot temperatures at low fluences. The derived values of E(m) and β are given in the figure legend.
Figure 6. Comparison of the modeled and measured average soot temperatures over 10 ns after the laser peak at HAB = 5 mm in Flame1.95. The inferred soot absorption function and the effective sublimation coefficient are E(m) = 0.25, β = 0.025 using the particle size distribution of dg = 8 nm and σg = 1.3.
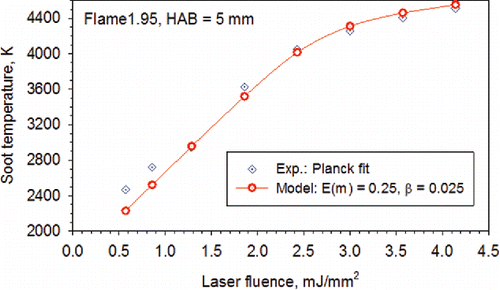
It is interesting to point out that the soot particles at HAB = 5 mm in Flame1.95 and at HAB = 10 mm in Flame1.75 have very similar E(m) (0.25 vs. 0.26) and β (0.025 vs. 0.02), consistent with the results shown in .
4.3. Time-resolved LII and primary particle size distributions
The experimentally detected time-resolved normalized LII signals at 532 nm at HAB = 7, 10, and 12 mm in Flame1.75 at a high laser fluence of 3.5 mJ/mm2 were used to derive the primary particle size distributions at these three heights, i.e., to determine the geometric mean primary diameter dg of the assumed lognormal distribution with σg = 1.15. presents a comparison between the measured and modeled time-resolved normalized 532 nm LII signals for the best fitted dg at these three heights in Flame1.75 using the HAB dependent E(m) and β derived above. At HAB = 12 mm, E(m) = 0.27 and β = 0.01 were used. The derived geometric mean particle diameters dg at these three heights are shown in the figure legend. Several observations can be made from . First, the LII signals decay very rapidly and at 100 ns from the onset of the laser pulse the LII signals drop to essentially noise levels. Such an LII signal duration is much shorter than that typically observed in experiments conducted in sooting diffusion flames of about several-hundred nano-seconds (Schultz et al. Citation2006; Snelling et al. Citation2004). Second, the initial LII signal decay rates at the three heights are almost the same in both the measured and modeled LII signals, which is expected at high laser fluences. Third, the measured normalized LII signals at the three heights start to deviate from about 15 ns onward and display a trend of slower decay with increasing HAB. However, the modeled LII signals at the three heights continue to be close to each other till about 30 ns. At longer times, the modeled LII signals begin to display the size effect, i.e., the LII signal at a higher HAB, which corresponds to larger particles, decays slower. From about 45 ns onward, the measured and modeled normalized LII signals agree well to each other at all the three heights. It is important to point out that the decay of normalized time-resolved LII signal under conditions where heat conduction cooling is dominant (approximately beyond 25 ns in ) is sufficiently sensitive to resolve a small variation of about 0.5 nm in the incipient soot particle size from about 3 to 4 nm in mean diameter.
Figure 7. Comparison of the measured and modeled time-resolved normalized LII signals at 532 nm at HAB = 7, 10, and 12 mm in Flame1.75 for a laser fluence of 3.5 mJ/mm2. The inferred geometric mean primary particle diameters dg for a fixed σg = 1.15 at these three heights are indicated in the figure legend.
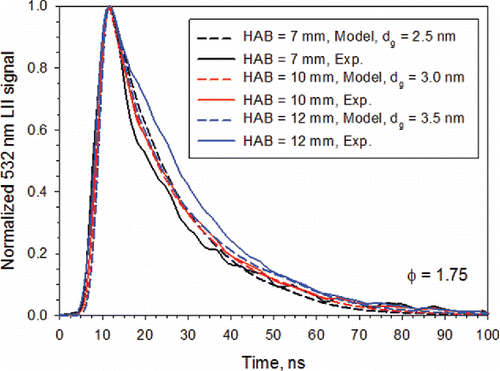
The inferred geometric mean primary particle diameters at HAB = 7, 10, and 12 mm in Flame1.75 are dg = 2.5, 3.0, and 3.5 nm, respectively. It is noticed that the fairly good agreement between the LII derived geometric mean particle diameter at HAB = 7 mm in Flame1.75 (dg = 2.5 nm) with that inferred from the experimental size distribution is expected, since the latter was used to infer TAC. Overall, the agreement between the modeled and measured time-resolved normalized 532 nm LII signals is considered fairly good at all three heights.
The experimental average soot temperatures over 10 ns starting from the laser peak at different laser fluences at HAB = 3.5 mm in Flame1.95 were not available to derive E(m), β, and particle size distribution. However, it was found that the measured time-resolved normalized 532 nm LII signal at HAB = 3.5 mm in Flame1.95 is nearly the same as that at HAB = 10 mm in Flame1.75. Therefore, it is reasonable to assume that the incipient soot particles at HAB = 3.5 mm in Flame1.95 and at HAB = 10 mm in Flame1.75 have the same particle size distribution and similar thermal and optical properties, i.e., dg = 3 nm, σg = 1.15, E(m) = 0.26, and β = 0.02.
At HAB = 5 mm in Flame1.95, the measured average soot temperatures over 10 ns after the laser peak and time-resolved normalized LII signal at 532 nm were used to derive E(m) and β shown in and particle size distribution shown in , which displays the measured and modeled time-resolved normalized 532 nm LII signals for F = 3.5 mJ/mm2 and at HAB = 3.5 and 5 mm in Flame1.95. The agreement between modeling and measurement is excellent at HAB = 3.5 mm in Flame1.95, suggesting that the soot particles at HAB = 3.5 mm in Flame1.95 possess the same properties and size distribution as those at HAB = 10 mm in Flame1.75. To verify this point, both the measured and modeled time-resolved normalized LII signals at HAB = 10 mm in Flame1.75 are also plotted in . It is evident that a very close agreement is found between the time-resolved normalized LII signals at HAB = 3.5 mm in Flame1.95 and at HAB = 10 mm in Flame1.75.
Figure 8. Comparison of the measured and modeled time-resolved normalized LII signals at 532 nm at HAB = 3.5 and 5 mm in Flame1.95. The inferred geometric mean primary particle diameters dg at these two heights are indicated in the figure legend. Also plotted in the figure are the measured and modeled time-resolved normalized LII signals are 532 nm at HAB = 10 mm in Flame1.75 (blue lines).
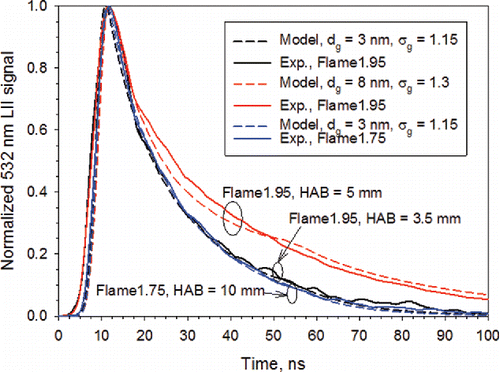
The agreement between the model and the measurement at HAB = 5 mm is also fairly good. The lognormal parameters of the derived particle size distribution at HAB = 5 mm are dg = 8 nm and σg = 1.3. This lognormal distribution was used to infer E(m) and β from the measured average soot temperatures shown in . It is also noticed that a wider geometric standard deviation σg = 1.3 was assumed at HAB = 5 mm in Flame1.95 to account for significant particle growth. The results shown in once again demonstrate that a change in incipient soot particle size is clearly reflected in the normalized time-resolved LII signal, i.e., LII is capable of resolving the size difference for small particles below 10 nm. Modeling the LII data at higher HAB in Flame1.95, where particle aggregation becomes significant, was not explored because the model used in this study did not take into account aggregation.
4.4. Fluence curves
The measured and modeled normalized fluence curves, i.e., the variation of the peak LII signal with the laser fluence, at HAB = 7 and 10 mm in Flame1.75 and at HAB = 5 mm in Flame1.95 at 532 nm are compared in the SI. The fluence curve is an important aspect to evaluate the performance and robustness of a LII model. The results shown in the SI confirm that the LII model, along with the model parameters, is able to reproduce fairly well the measured fluence curves, confirming the overall good performance of the model.
5. Results and discussion: Advanced knowledge of size distributions of incipient soot particles by combining LII, HIM, and SMPS
In the above sections, the size distribution of incipient soot particles has been determined by using LII. The interest for particle sizing by LII is strongly related to the high sensitivity of this in situ technique, its real-time potential, its high spatial and temporal resolution, allowing applications in turbulent flames and technical environments. In this work, LII has been used to detect incipient soot particles in nucleation flames by improving the set-up and experimental methodology to get the required sensitivity. Advanced LII modeling was carried out to determine the size of incipient soot particles from the time-resolved signals. However, the size determination by LII is still confronted by the lack of well-established knowledge of several critical parameters of incipient soot particles, as detailed in Section 3. Some of the model parameters were chosen here based on the literature. Two sensitive parameters, i.e., E(m) and β, were determined by fitting the model results to the LII data obtained at a particular set of conditions. These data consist of the experimental temperatures of soot particles heated at different fluences and the experimentally detected time-resolved LII signals. The reliability of the model was improved by incorporating the size distribution obtained by SMPS in the nucleation flame at HAB = 7 mm as input.
In this section, the results obtained independently by ex situ HIM of particles sampled thermophoretically and by online size distribution analysis of microprobe-sampled particles using a 1nm-SMPS are presented and compared with those by LII to assess the potential of using LII as an in situ particle sizing technique for incipient soot.
5.1. Particle size measurements – HIM
shows representative HIM images obtained for different samples. In Flame1.95, very small particles are observed at the nucleation zone, (HAB = 3.5 mm), larger particles and small aggregates are present in the early mass growth zone, (HAB = 6.0 mm), and larger aggregates are formed subsequently, (HAB = 10 mm). This behavior is consistent with soot growth and aggregation processes expected in a sooting premixed flame (Wang Citation2011). In the nucleation flame, Flame1.75, the particles responsible for the LII signals can still be well identified, . Their relevant HIM image is qualitatively close to the one obtained in the nucleation zone of Flame1.95, .
Figure 9. HIM images obtained for the samples in Flame1.95 at HAB = 10 mm (a), 6 mm (b), and 3.5 mm (c), and in Flame1.75 at HAB = 12 mm (d). Twenty sweeps were used for the sampling in Flame1.95 at all the three heights and 70 sweeps were used in Flame1.75. The brightness and contrast were adjusted as explained in the online supplementary information.
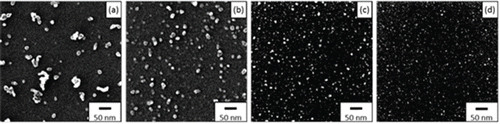
The size determination on the images is affected by uncertainties linked to the quality of the contrast and to the human estimation of the contour of the primary particles. By studying several samples obtained in the same flame conditions and the same images by different users, the peak of the size distribution is estimated to be affected by ± 0.6 nm in Flame 1.95 at 10 mm. These images were processed as detailed in Section 2.4 to determine the soot size distributions at different HABs in Flame1.95.
The particle size distributions determined by HIM image analysis are displayed in . The particle sizes increase from the nucleation zone (HAB = 3.5 mm) to the surface growth and aggregation zones at higher HABs. The nucleation zone (HAB = 3.5 mm) highlights soot particles with the smallest arithmetic average diameter of 4.6 nm. This diameter increases to 8 nm in the early mass growth at HAB = 6 mm and to 10.2 nm in the coagulation region at HAB = 10 mm. These distributions may have been broadened by the sampling procedure that relied on a swing movement of the sample across the flame. Indeed, it has been shown that premixed flat sooting flames may have some distortions at the boundary of the flame, or radial inhomogeneity of the flame temperature and soot volume fraction (Migliorini et al. Citation2008; Xuan and Blanquart Citation2016). The corresponding time-resolved normalized LII measurements are shown in . The height of the laser-probed volume for LII measurements is 0.37 mm as determined by the image of the slit width into the flame. This size was found necessary to get a sufficient signal-to-noise ratio at low heights in the nucleation flame (Flame1.75). represents the time-resolved LII signals with a color scale corresponding to the HIM measurements affected by a spatial resolution estimated to be ±0.5 mm. In , the blue traces are LII signals collected for the HAB range between 3 and 4.5 mm, the red ones for 5–7 mm range and the black ones for 9–11 mm. HIM measurements were conducted over each of these three HAB ranges. The slower LII signal decays with increasing HAB suggest that the particle sizes become larger at a higher HAB (Bladh et al. Citation2015; Will et al. Citation1995; Schultz et al. Citation2006). Even though this comparison is qualitative, it illustrates the high potential of using LII to capture the spatial evolution of incipient soot particles, at least as a complementary approach to the thermophoretic sampling technique. Again, it can be observed from the measured normalized time-resolved LII signals shown in that LII is clearly sensitive enough to resolve particle size variation of incipient soot particles with HAB for mean particles from about 4.5 to 10 nm in diameter.
Figure 10. Particle size distributions and arithmetic average size (A.S) of soot particles sampled at (a) HAB = 3.5, 6.0, and 10.0 mm in Flame1.95 obtained by HIM image analysis (manual method) and (b) time-resolved normalized LII signals at 532 nm in Flame1.95 at different ranges of HAB as indicated by the colors (dark gray [blue]: 3, 3.5, 4 and 4.5 mm; light gray [red]: 5, 5.5, 6, and 7 mm; black: 9, 10, and 11 mm).
![Figure 10. Particle size distributions and arithmetic average size (A.S) of soot particles sampled at (a) HAB = 3.5, 6.0, and 10.0 mm in Flame1.95 obtained by HIM image analysis (manual method) and (b) time-resolved normalized LII signals at 532 nm in Flame1.95 at different ranges of HAB as indicated by the colors (dark gray [blue]: 3, 3.5, 4 and 4.5 mm; light gray [red]: 5, 5.5, 6, and 7 mm; black: 9, 10, and 11 mm).](/cms/asset/759ba131-4c7f-4e9e-a793-b09608f31e96/uast_a_1325440_f0010_oc.gif)
5.2. 1 nm-SMPS results
The online measurements of PSDF using the 1nm-SMPS were taken at HAB = 6, 8, and 10 mm in Flame1.75 and are shown in as a function of the mobility diameter, which is essentially the same as the particle diameter in the case of unaggregated primary particles. The absolute particle number concentration can have relatively high uncertainties in the sub-5 nm range. The size distributions have not been corrected and post-processed for diffusion losses. Particularly it is expected that diffusion losses can increase with decreasing mobility diameter Dp, which tends to reduce the particle count in the smallest size bins. A further correction would presumably lead to a small shift towards the lower sizes. Also a higher dilution ratio could be worth considering, since residual post-sampling coagulation might be responsible of the slightly asymmetric PSDFs shown in . However, the clear peaks around 3 nm suggest that there are not too many smaller particles to be expected. There are no significant numbers of particles smaller than 2 nm detected. Considering the current uncertainty for diffusion loss corrections applied to very small particles, and since the determination of the absolute concentration was not required in the current work, size distributions have not been corrected and post-processed for diffusion losses as was performed by Abid et al. (Citation2009).
Figure 11. The measured particle-size distribution functions (PSDF) by 1 nm-SMPS at HAB = 6, 8, and 10 mm in Flame1.75. The lines (red) are fits to data using a lognormal distribution function and the corresponding fitting parameters are shown in the figure.
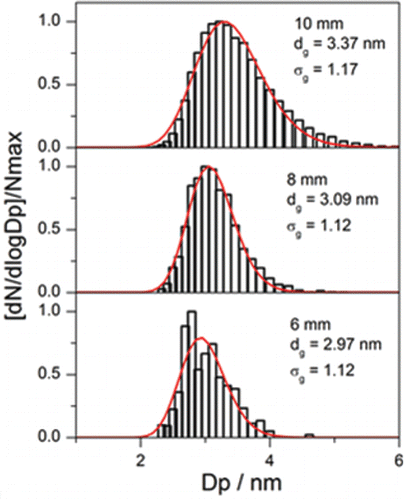
These distributions (normalized) have a geometric mean diameter around 3 nm. They are quite narrow (geometric standard deviation about 1.12) in comparison with HIM distributions (σg = 1.27, see below). Indeed, the axial sampling by the taper-shapeed probe leads to a better spatial resolution, which is estimated to around two sampling-orifice diameter, i.e., ∼0.2 mm. With increasing HAB the mean diameter increases about 10% and the distribution becomes slightly broader, consistent with the slight decrease in the decay rate of time-resolved normalized LII signal in Flame1.75 shown in . The 1nm-SMPS measurements confirm that the soot growth process is very weak in the nucleation flame (Flame1.75).
5.3. Comparison of size distributions of incipient soot by LII-HIM-SMPS
Few studies in the literature have made inter-comparisons of soot particle size measurement in flat premixed flames using different techniques for the purpose of either understanding the advantages and disadvantages of photoionization mass spectrometry (PIMS), SMPS, and LII and their applicability range (Stirn et al. Citation2009) or investigating the causes for the different soot particle sizes measured by SMPS and TEM (Zhao et al. Citation2007). However, there have been no studies to demonstrate the potential of using LII to measure the size of incipient soot in nucleation flames. Our study represents the first effort to evaluate the suitability and capability of LII as an in situ particle sizing technique for incipient soot measurement in the nucleation region of premixed flames using independent results obtained by HIM and 1nm-SMPS. As discussed above, each method provides a particle size distribution at each position sampled in the flame. The measured distribution is likely a result that consists of two parts: (1) the actual size distribution of the particles resulting from the chemical and physical processes they have undergone in the flame and (2) some artifacts, such as bias inherent to each technique and the inadequate spatial resolution of the technique. SMPS is able to provide a narrow PDSF. Only a relatively small number of particles sampled on the grid can be analyzed by HIM; therefore, HIM typically only provides a fairly coarse particle size distribution in histogram form. As explained in Section 3, the size distribution obtained by 1nm-SMPS at HAB = 7 mm in Flame1.75 was used as an input for LII modeling to estimate TAC of incipient soot. Then the particle size distribution in the form of log-normal expression can be recovered by a combined use of LII measurements and modeling through multivariable optimization.
A direct comparison of the particle size distributions measured by 1nm-SMPS, LII and HIM at two flame heights in Flame1.75 are displayed in . HIM distributions (in counts, automatic method) taken from Supplementary information were transformed in dN/dlogDp in and could be fitted by a log-normal distribution (at HAB = 12 mm, dp = 3.3 nm, σg = 1.27). It is important to recall that the LII measurements are non-intrusive. The insertion of any physical probe required for the SMPS or HIM sampling could significantly disturb the flame. At HAB = 10 mm, the mean primary particle size derived by LII is somewhat smaller than that obtained by 1nm-SMPS, . The shift can be at least to some extent explained by the difference between electrical mobility equivalent diameter from the SMPS to the physical diameter (also called mass or geometrical). The geometric mean particle diameter obtained by LII agrees fairly well with that by HIM analysis at HAB = 12 mm, . However, the HIM size distribution contains a small number of larger particles about 5 to 8 nm, which can be explained by the potential sampling artifacts and the splash effect of liquid-like particles on the sampling grid mentioned above. Nevertheless, considering the differences among the three techniques in working principle (in situ, ex situ, and online) and the different sampling procedures between HIM and 1nm-SMPS, the agreement between the distributions obtained by these techniques is quite good. Further investigations will be pursued to explore the origin of the shift in the particle size distributions between 1nm-SMPS and LII observed in .
Figure 12. Comparison of normalized particle size distributions measured by 1 nm-SMPS, HIM (automatic method), and LII in Flame1.75 at (a) HAB = 10 mm and (b) HAB = 12 mm. In (a) the 1 nm-SMPS results are shown as vertical bars and LII results as the solid blue line. In (b) the HIM results are given as bars and LII as the solid line (blue).
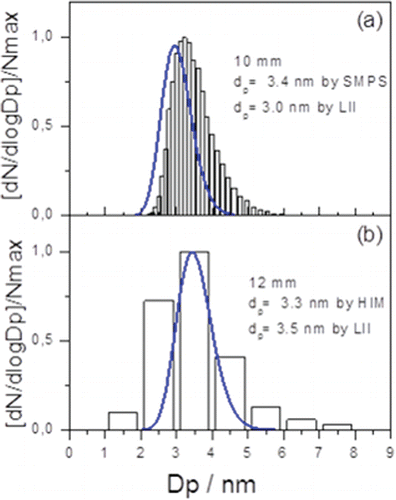
compares the geometric mean diameters of soot particles obtained with the three techniques as a function of HAB in the nucleation flame (Flame1.75). The figure highlights the very good agreement between the results obtained by LII, 1nm-SMPS, and HIM, particularly considering the different ways of soot sampling and different nature of the techniques, i.e., in situ, ex situ, and online.
Figure 13. Variation of the geometric mean diameter of the incipient soot particles with HAB in the nucleation flame measured by the three techniques.
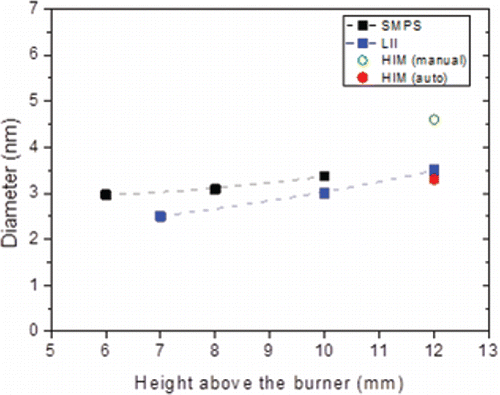
In Flame1.95 soot growth occurs once particles are formed as noticed by the slight decrease of the LII signal decay rates shown in . In addition, the particle size distribution determined by HIM at 3.5 mm in this flame is likely broadened by the sampling procedure as mentioned in Section 5.1 and the spatial resolution estimated to ±0.5 mm, which tend to collect primary soot particles of larger mean diameters and a wider distribution (arithmetic average diameter = 4.6 nm, ). Despite this bias, a good consistency between LII and HIM is also found in Flame1.95 in the early soot formation zone. These results again confirm that the particles responsible for the detected LII signals, which are identified as soot entities as explained in Section 1, are indeed those identified by HIM and measured by 1nm-SMPS. These incipient soot particles or “nuclei” have diameters around 3 nm. Although HIM provides broader size distributions in comparison with LII and 1nm-SMPS, it is a vital tool for the measurements of incipient soot particles below 10 nm. Indeed, HIM provides direct evidence for the gradual evolution from incipient particles to small aggregates highlighted in Flame1.95. The close resemblance between HIM images obtained at HAB = 3.5 mm in Flame1.95 and at HAB = 12 mm in Flame1.75 is a clear indication that the incipient soot particles identified in the nucleation flame have very similar characteristics as those found in the nucleation zone of the sooting flame. It is consistent with the similarity of LII behavior for particles at HAB = 3.5 mm in Flame1.95 and at HAB = 10 to 12 mm in Flame1.75 for time-resolved and spectrally-resolved signals as well as the fluence curve, as mentioned in the previous sections. This finding is important to refine soot formation modeling. Finally, confirms that there is only a very slight increase in the particle diameter with HAB in the nucleation flame (Flame1.75), supporting the conclusions of our previous studies (Mouton et al. Citation2013; Bladh et al. Citation2015; Faccinetto et al. Citation2017) based only on LII measurements.
The good agreement between the particle size distributions measured by the three techniques indicates that the LII model along with the model parameters (some were selected from the literature and some were determined based on the present LII measurements) gives satisfying description of the LII behavior of incipient soot particles. Finally, it is worth pointing out that the goal of this study is to explore the potential of using LII as an in situ sizing technique for incipient soot particles in the nucleation region of flames where the particle size distribution is unimodal. It has been well-established that the size distribution function of particle mobility diameter evolves into a bimodal distribution above the nucleation zone in sooting flames due to the presence of both particle nucleation and aggregation (Zhao et al. Citation2003, Citation2007; Abid et al. Citation2008; Stirn et al. Citation2009). Under these conditions, it is not feasible to use LII to retrieve the bimodality of the particle size distribution since small particles contribute negligibly to the LII signals in the presence of large ones.
6. Conclusions
Laser-induced incandescence measurements were conducted using a 1064 nm laser pulse in two laminar flat premixed n-butane flames at equivalence ratios of 1.75 and 1.95. The equivalence ratio of 1.75 corresponds to the nucleation flame condition where the flame only displays faint red color above the blue base and incipient soot particles undergo nearly negligible surface growth, while the richer flame of equivalence ratio 1.95 is much more luminous and soot particles undergo significant surface growth and aggregation. Time-resolved LII signals were detected at 532 and 650 nm and spectrally resolved signal detection in the visible spectrum between 450 and 800 nm was also performed over 10 ns at the peak of the laser. A multivariable optimization procedure was proposed to simultaneously retrieve the absorption function, the effective sublimation coefficient, and particle size distribution of incipient soot by matching the modeled time-resolved normalized LII signals and the average soot temperatures for a range of laser fluences to the measurements. To validate the LII inferred soot particle size distributions in flame conditions where particle aggregation is negligible and the particle size distribution is unimodal, additional particle size measurements were carried out using helium-ion microscope (HIM) and a 1-nm scanning mobility particle sizer (1nm-SMPS). This study represents the first effort to explore the capability of LII as an in situ sizing technique for incipient soot produced in either the nucleation flame or early soot formation region of sooting flames. The following conclusions can be drawn based on the present experimental and numerical studies:
1. | Incipient soot particles in the 2–4 nm range can be detected independently using LII, HIM, and 1nm-SMPS and the particle size distributions measured by these three techniques display good agreement. | ||||
2. | The good agreement in the measured particle size distributions in the nucleation flame and the nucleation region of the sooting flame and the continuous signals of the spectrally-resolved detection in the visible spectrum, without or with laser heating, are clear indications that incipient soot particles as small as 2 to 4 nm are capable of absorbing laser energy to emit LII signals. | ||||
3. | Incipient soot particles formed in the nucleation flame of equivalence ratio 1.75 undergo only minor growth along the flame with a mean diameter of about 3 nm. This feature confirms the kinetic interest of such flames for a better understanding of the soot nucleation process. | ||||
4. | The incipient soot particles at HAB = 3.5 mm in Flame1.95 have very similar optical and thermal properties as those at HAB = 10 mm in Flame1.75 as far as LII measurements and modeling are concerned. This indicates that the nucleation process in different flames may involve similar incipient soot particles. This finding deserves further studies. | ||||
5. | The absorption function of incipient soot at 1064 nm is slightly above 0.2 and increases modestly with increasing HAB to reach about 0.27 in the nucleation flame. This range of soot absorption functions is similar to those derived in several previous studies. The thermal accommodation coefficient of incipient soot was also found similar to that of mature soot. However, the effective sublimation coefficient of incipient soot was found significantly lower than that typically used in LII modeling of mature soot. |
It is interesting to notice that the thermal and optical properties of incipient soot are not drastically different from those of mature soot commonly used in LII modeling. The spectrally resolved signals and the agreement between the LII model results using the somewhat similar thermal and optical properties to those of mature soot and the measured time-resolved signals and particle temperatures can be considered as clear indications that the nucleation soot formed in the rich premixed flat flames is capable of producing continuous thermal emissions in the visible spectrum. Further research is required to conduct LII measurements and modeling of incipient soot in other flame conditions to evaluate the robustness of the model parameters and the multivariable optimization approach proposed in this study.
UAST_1325440_Supplementary_File.zip
Download Zip (1 MB)Funding
The authors thank the Région “Hauts de France,” the Ministère de l'Enseignement Supérieur et de la Recherche (CPER Climibio), the European Fund for Regional Economic Development and ANR-13-TDMO-0002 ASMAPE for their financial support. The CaPPA project (Chemical and Physical Properties of the Atmosphere) is funded by the French National Research Agency (ANR) through the Programme d'Investissement d'Avenir under contract ANR-11-LABX-0005. Maurin Salamanca thanks the Alexander von Humboldt Foundation for the financial support. HIM experiments in Bielefeld have been financially supported by COST Action CM1404 Chemistry of Smart Energy Carriers and Technologies.
References
- Abid, A.D., Heinz, N., Tolmachoff, E.D., Phares, D.J., Campbell, C.S., and Wang, H. (2008). On Evolution of Particle Size Distribution Functions of Incipient Soot in Premixed Ethylene-Oxygen-Argon Flames. Combust. Flame, 154:775–788.
- Abid, A.D., Tolmachoff, E.D., Phares, D.J., Wang, H., Liu, Y., and Laskin, A. (2009). Size Distribution and Morphology of Nascent Soot in Premixed Ethylene Flames with and without Benzene Doping. Proc. Combust. Inst., 32:681–688.
- Baron, P.A., and Willeke, K. (2005). Aerosol Measurement – Principles, Techniques, and Applications. Second ed., J. Wiley & Sons.
- Bejaoui, S., Batut, S., Therssen, E., Lamoureux, N., Desgroux, P., and Liu, F. (2015). Measurements and Modeling of Laser-Induced Incandescence of Soot at Different Heights in a Flat Premixed Flame. Appl. Phys. B, 118(3):449–469.
- Bessler, W.G., and Schulz, C. (2004). Quantitative Multi-Line NO-LIF Temperature Imaging. Appl. Phys. B, 78(5):519–533.
- Bladh, H., Johnsson, J., Olofsson, N.-E., Bohlin, A., and Bengtsson, P.-E. (2011). Optical Soot Characterization using Two-Color Laser-Induced Incandescence (2C-LII) in the Soot Growth Region of a Premised Flat Flame. Proc. Combust. Inst., 33:641–648.
- Bladh, H., Olofsson, N.-E., Mouton, T., Simonsson, J., Mercier, X., Faccinetto, A., Bengtsson, P.-E., and Desgroux, P. (2015). Probing the Smallest Soot Particles in Low-Sooting Premixed Flames using Laser-Induced Incandescence. Proc. Combust. Inst., 35:1843–1850.
- Camacho, J., Liu, C., Gu, C., Lin, H., Huang, Z., Tang, Q., You, X., Saggese, C., Li, Y., Jung, H., Deng, L., Wlokas, I., and Wang, H. (2015). Mobility Size and Mass of Nascent Soot Particles in a Benchmark Premixed Ethylene Flame. Combust. Flame, 162:3810–3822.
- Cléon, G., Amodeo, T., Faccinetto, A., and Desgroux, P. (2011). Laser Induced Incandescence Determination of the Ratio of the Soot Absorption Functions at 532 nm and 1–64 nm in the Nucleation Zone of a Low Pressure Premixed Sooting Flame. Appl. Phys. B, 104:297–305.
- D'Alessio, A., D'Anna, A., Minutolo, P., Sgro, L.A., and Violi, A. (2000). On the Relevance of Surface Growth in Soot Formation in Premixed Flames. Proc. Combust. Inst., 28:2547–2554.
- D'Anna, A., Rolando, A., Allouis, C., Minutolo, P., and D'Alessio, A. (2005). Nano-Organic Carbon and Soot Particle Measurements in a Laminar Ethylene Diffusion Flame. Proc. Combust. Inst., 30:1449–1456.
- D'Anna, A. (2009). Combustion-Formed Nanoparticles. Proc. Combust. Inst., 32:593–613.
- Denisov, A., Colmegna, G., and Jansohn, P. (2014). Temperature Measurements in Sooting Counterflow Diffusion Flames Using Laser-Induced Fluorescence of Flame-Produced Nitric Oxide. Appl. Phys. B, 116(2):339–346.
- Desgroux, P., Mercier, X., and Thomson, K.A. (2013). Study of the Formation of Soot and its Precursors in Flames Using Optical Diagnostics. Proc. Combust. Inst., 34:1713–1738.
- Dobbins, R.A., and Megaridis, C.M. (1987). Morphology of Flame-Generated Soot as Determined by Thermophoretic Sampling. Langmuir, 3:254–259.
- Dobbins, R.A., and Subramaniasivam, H. (1994). Soot Precursor Particles in Flames, in Mechanisms and Models of Soot Formation – Lecture Notes in Physics. H. Bockhorn ( Ed.) Springer-Verlag, New York, Chap. 17.
- Eremin, A., Gurentsov, E., Popova, E., and Priemchenko, K. (2011). Size Dependence of Complex Refractive Index Function of Growing Nanoparticles. Appl. Phys. B, 104:285–295.
- Eremin, A., Gurentsov, E., Mikheyeva, E., and Priemchenko, K. (2013). Experimental Study of Carbon and Iron Nanoparticle Vaporization under Pulse Laser Heating. Appl. Phys. B, 112:421–432.
- Faccinetto, A., Mercier, X., Mouton, T., El Bakali, A., Aubagnac Karkar, D., Desgroux, P. (2017). Comparative Study of the Soot Formation Process in a “Nucleation” and a “Sooting” Low Pressure Premixed Methane Flame. Combust. Flame, under revision.
- Filippov, A.V., and Rosner, D.E. (2000). Energy Transfer Between an Aerosol Particle and Gas at High Temperature Ratios in the Knudsen Transition Regime. Int. J. Heat Mass Transfer, 43:127–138.
- Goulay, F., Nemes, L., Schrader, P.E., and Michelsen, H.A. (2010). Spontaneous Emission from C2 (d 3Πg) and C3 (A 1Πu) during Laser Irradiation of Soot Particles. Mol. Phys., 108(7–9):1013–1025.
- Goulay, F., Schrader, P.E., López-Yglesias, X., and Michelsen, H.A. (2013). A Data Set for Validation of Models of Laser-Induced Incandescence from Soot: Temporal Profiles of LII Signal and Particle Temperature. Appl. Phys. B, 112(3):287–306.
- Gu, C., Lin, H., Camacho, J., Lin, B., Shao, C., Li, R., Gu, H., Guan, B., Huang, Z., and Wang, H. (2016). Particle Size Distribution of Nascent Soot in Lightly and Heavily Sooting Premixed Ethylene Flames. Combust. Flame, 165:177–187.
- Hartlieb, A.T., Atakan, B., and Kohse-Höinghaus, K. (2000). Temperature Measurement in Fuel-Rich Non-Sooting Low-Pressure Hydrocarbon Flames. Appl. Phys. B, 70(3):435–445.
- Hlawacek, G., and Gölzhäuser, A. ( eds.) (2016). Helium Ion Microscopy, Springer International Publishing, Cham.
- Hu, B., Yang, B., and Koylu, U.O. (2003). Soot Measurements at the Axis of an Ethylene/air Non-Premixed Turbulent Jet Flame. Combust. Flame, 134:93–106.
- Available from https://imagej.nih.gov/ij/docs/guide/146-30.html#fig:Display-options-Particle-Analyzer (2016-17-11)
- Jiang, J., Chen, M., Kuang, C., Attoui, M., and McMurry, P. H. (2011). Electrical Mobility Spectrometer using a Diethylene Glycol Condensation Particle Counter for Measurement of Aerosol Size Distributions Down to 1 nm. Aerosol Sci. Technol., 45(4):510–521.
- Kuhlmann, S., Reimann, J., and Will, S. (2006). On Heat Conduction Between Laser-Heated Nanoparticles and a Surrounding Gas. J Aerosol Sci., 37:1696–1716.
- Levendis, Y. A., Estrada, K. R., and Hottel, H. C. (1992). Development of Multicolor Pyrometers to Monitor the Transient Response of Burning Carbonaceous Particles. Rev. Sci. Instrum., 63:3608–3622.
- Li, Z., and Wang, H. (2004). Thermophoretic Force and Velocity of Nanoparticles in the Free Molecule Regime. Phys. Rev. E, 70:021205.
- Iida, K., Stolzenburg, M. R., and McMurry, P. H. (2009). Effect of Working Fluid on sub2 nm Particle Detection with a Laminar Flow Ultrafine Condensation Particle Counter. Aerosol Sci. Technol., 43(1):81–96.
- Liu, F., Stagg, B.J., Snelling, D.R., and Smallwood, G.J. (2006). Effects of Primary Soot Particle size Distribution on the Temperature of Soot Particles Heated by a Nanosecond Pulsed Laser in an Atmospheric Laminar Diffusion Flame. Int. J. Heat Mass Transfer, 49:777–788.
- López-Yglesias, X., Schrader, P.E., and Michelsen, H.A. (2014). Soot Maturity and Absorption Cross Sections. J. Aero. Sci., 75:43–64.
- Maffi, S., De Iuliis, S., Cignoli, F., and Zizak, G. (2011). Investigation on Thermal Accommodation Coefficient and Soot Absorption Function with Two-Color Tire-LII Technique in Rich Premised Flames. Appl. Phys. B, 104:357–366.
- Maricq, M.M., Harris, S.J., and Szente, J.J. (2003). Soot Size Distributions in Rich Premixed Ethylene Flames. Combust. Flame, 132:328–342.
- Michelsen, H.A., Liu, F., Kock, B.F., Bladh, H., Boiarciuc, A., Charwath, M., Dreier, T., Hadef, R., Hofmann, M., Reimann, J., Will, S., Bengtsson, P.-E., Bockhorn, H., Foucher, F., Geigle, K.-P., Mounaïm-Rousselle, C., Schultz, C., Stirn, R., Tribalet, B., and Suntz, R. (2007). Modeling Laser-Induced Incandescence of Soot: a Summary and Comparison of LII Models. Appl. Phys. B, 87:503–521.
- Michelsen, H.A. (2009). Derivation of a Temperature-Dependent Accommodation Coefficient for Use in Modeling Laser-Induced Incandescence of Soot. Appl. Phys. B, 94:103–117.
- Michelsen, H.A. (2017). Probing Soot Formation, Chemical and Physical Evolution, and Oxidation: A Review of in situ Diagnostic Techniques and Needs. Proc. Combust. Inst. 36(1):717–735.
- Migliorini, F., De Iuliis, S., Cignoli, F., and Zizak, G. (2008). How “Flat” is the Rich Premixed Flame Produced by your McKenna Burner?, Combust. Flame, 153(3):384–393.
- Migliorini, F., Thomson, K.A., and Smallwood, G.J. (2011). Investigation of Optical Properties of Aging Soot. App. Phys. B, 104:273–283.
- Mouton, T., Mercier, X., Wartel, M., Lamoureux, N., and Desgroux, P. (2013). Laser-Induced Incandescence Technique to Identify Soot Nucleation and Very Small Particles in Low-Pressure Methane Flames. Applied Phys. B, 112:369–379.
- Olofsson, N.-E., Simonsson, J., Török, S., Bladh, H., and Bengtsson, P.-E. (2015). Evolution of Properties for Aging Soot in Premixed Flat Flames Studied by Laser-Induced Incandescence and Elastic Light Scattering. Appl. Phys. B, 119:669–683.
- Saggese, C., Cuoci, A., Frassoldati, A., Ferrario, S., Camacho, J., Wang, H., and Faravelli, T. (2016). Probe Effects in Soot Sampling from a Burner-Stabilized Stagnation Flame. Combust. Flame, 167:184–197.
- Schenk, M., Lieb, S., Vieker, H., Beyer, A., Gölzhäuser, A., Wang, H., and Kohse-Höinghaus, K. (2013). Imaging Nanocarbon Materials: Soot Particles in Flames are not Structurally Homogeneous. Chen Phys Chem, 14:3248–3254.
- Schenk, M., Lieb, S., Vieker, H., Beyer, A., Gölzhäuser, A., Wang, H., Kohse-Höinghaus, K. (2015). Morphology of Nascent Soot in Ethylene Flames. Proc. Combust. Inst., 35:1879–1886.
- Schultz, C., Kock, B.F., Hofmann, M., Michelsen, H., Will, S., Bougie, B., Suntz, R., and Smallwood, G. (2006). Laser-Induced Incandescence: Recent Trends and Current Questions. Appl. Phys. B, 83:333–354.
- Sgro, L.A., Minutolo, P., Basile, G., and D'Alessio, A. (2001). UV-Visible Spectroscopy of Organic Carbon Particulate Sampled from Ethylene/air Flames. Chemosphere, 42:671–680.
- Sgro, L.A., De Filippo, A., Lanzuolo, G., and D'Alessio, A. (2007). Characterization of Nanoparticles of Organic Carbon (NOC) Produced in Rich Premixed Flames by Differential Mobility Analysis. Proc. Combust. Inst., 31:631–638.
- Sgro, L.A., Barone, A.C., Commodo, M., D'Alessio, A., De Filipo, A., Lanzuolo, G., and Minutolo, P. (2009). Measurement of Nanoparticles of Organic Carbon in Non-Sooting Flame Conditions. Proc. Combust. Inst., 32:689–696.
- Simonsson, J., Olofsson, N.-E., Török, S., Bengtsson, P.-E., and Bladh, H. (2015). Wavelength Dependence of Extinction in Sooting Flat Premixed Flames in the Visible and Near Infrared Regimes. Appl. Phys. B, 119:657–667.
- Simonsson, J., Olofsson, N.-E., Bladh, H., Sanati, M., and Bengtsson, P.-E. (2017). Influence of Potassium and Iron Chloride on the Early Stages of Soot Formation Studied using Imaging LII/ELS and TEM Techniques. Proc. Combust. Inst., 36(1):853–860.
- Skeen, S.A., Michelsen, H.A., Wilson, K.R., Popolan, D.M., Violi, A., and Hansen, N. (2013). Near-Threshold Photoionization Mass Spectra of Combustion-Generated High-Molecular-Weight Soot Precursors. J. Aerosol Sci., 58:86–102.
- Smallwood, G.J., Snelling, D.R., Liu, F., and Gülder, Ö.L. (2001). Clouds Over Soot Evaporation: Errors in Modeling Laser-Induced Incandescence of Soot. ASME J. Heat Transfer, 123:814–818.
- Snelling, D.R., Liu, F., Smallwood, G.J., and Gülder, Ö.L. (2004). Determination of the Soot Absorption Function and Thermal Accommodation Coefficient Using Low-Fluence LII in a Laminar Coflow Ethylene Diffusion Flame. Combust. Flame, 136:180–190.
- Sorensen, C.M. (2001). Light Scattering by Fractal Aggregates: A Review. Aerosol Sci. Technol., 35:648–687.
- Stirn, R., Gonzalez Baquet, T., Kanjarkar, S., Meier, W., Geigle, K.P., Grotheer, H.H., Wahl, C., and Aigner, M. (2009). Comparison of Particle Size Measurements with Laser-Induced Incandescence, Mass Spectroscopy, and Scanning Mobility Particle Sizing in a Laminar Premixed Ethylene/Air Flame. Combust. Sci. Technol., 181:329–349.
- Tang, Q., Cai, R., You, X., and Jiang, J. (2017). Nascent Soot Particle Size Distributions Down to 1 nm from a Laminar Premixed Burner-Stabilized Stagnation Ethylene Flame. Proc. Combust. Inst., 36(1):993–1000.
- Thierley, M., Grotheer, H.-H., Aigner, M., Yang, Z., Abid, A., Zhao, B., and Wang, H. (2007). On Existence of Nanoparticles Below the Sooting Threshold. Proc. Combust. Inst., 31:639–647.
- Totton, T.S., Chakrabarti, D., Misquitta, A.J., Sander, M., and Wales, D.J. (2010). Modelling the Internal Structure of Nascent Soot Particles. Combust. Flame, 157:909–914.
- Tröstl, J., Tritscher, T., Bischof, O.F., Horn, H.-G., Krinke, T.J., Baltensperger, U., and Gysel, M. (2015). Fast and Precise Measurement in the sub-20 nm Size Range using a Scanning Mobility Particle Sizer. J. Aerosol Sci., 87:75–87.
- Wang, S.C., and Flagan, R.C. (1990). Scanning Electrical Mobility Spectrometer. Aerosol Sci. Technol., 13:230–240.
- Wang, H. (2011). Formation of Nascent Soot and Other Condensed-Phase Materials in Flames. Proc. Combust. Inst., 33:41–67.
- Ward, B.W., Notte, J.A., and Economou, N.P. (2006). Helium Ion Microscope: A New Tool for Nanoscale Microscopy and Metrology. J. Vac. Sci. Technol. B, 24:2871–2874.
- Will, S., Schraml, S., and Leipertz, A. (1995). Two-Dimensional Soot-Particle Sizing by Time-Resolved Laser-Induced Incandescence. Opt. Lett., 20(22):2342–2344.
- Zhang, R., Zhang, Y., and Kook, S. (2017). Morphological Variations of in-Flame and Exhaust Soot Particles Associated with Jet-to-Jet Variations and Jet–Jet Interactions in a Light-Duty Diesel Engine. Combust. Flame, 176:377–390.
- Zhao, B., Yang, Z., Johnston, M.V., Wang, H., Wexler, A.S., Balthasar, M., and Kraft, M. (2003). Measurement and Numerical Simulation of Soot Particle Size Distribution Functions in a Laminar Premixed Ethylene-Oxygen-Argon Flame. Combust. Flame, 133:173–188.
- Zhao, B., Uchikawa, K., and Wang, H. (2007). A Comparative Study of Nanoparticles in Premixed Flames by Scanning Mobility Particle Sizer, Small Angle Neutron Scattering, and Transmission Electron Microscopy. Proc. Combust. Inst., 31:851–860.
- Xuan, Y., and Blanquart, G. (2016). Two-Dimensional Flow Effects on Soot Formation in Laminar Premixed Flames. Combust. Flame, 166:113–124.