ABSTRACT
Upper-room ultraviolet germicidal irradiation (UR-UVGI) is recommended for the defense against airborne pathogens in poorly ventilated rooms in public buildings or high risk environments, such as hospitals. Currently, there are few studies on the performance of UR-UVGI with the effective bacteria susceptibility constant (eZ-value) obtained from UR-UVGI experiment. In this study, the Eulerian model for the inactivation of UVGI was improved to consider the difference between exposure time and computational time, and was validated by our previous experiments. The method was applied to study numerically the performance of the UR-UVGI for 3, 6, and 10 air changes per hour (ACH) with the application of the eZ-value of airborne bacteria. Moreover, the eZ-values of commonly found bacteria, including Serratia marcescens, Staphylococcus epidermidis, Pseudomonas alcaligenes, and Micrococcus luteus, were obtained from a series of experiments in a full-scale environmental chamber equipped with a UR-UVGI fixture. The eZ-values were 0.0983, 0.0586, 0.0476, and 0.0115 m2/J, respectively. Compared with the data in the literature, these eZ-values of the tested bacteria are different from the Z-values obtained from single-pass UVGI. The simulation results show that UR-UVGI exhibited the highest inactivation efficiency on S. marcescens among the tested bacteria in this study. The percentage of bacteria inactivated by UR-UVGI decreased as the ventilation rate increased. The bacteria concentration in the breathing zone under low ventilation rate could be decreased using UR-UVGI. The findings demonstrate that high indoor air quality can be achieved with the application of UR-UVGI without the need to maintain high ventilation rate.
Copyright © 2017 American Association for Aerosol Research
EDITOR:
1. Introduction
Ultraviolet germicidal irradiation (UVGI) air disinfection utilizing the UV C-band (UVC; 200–280 nm) has been shown to sterilize air and inactivate airborne pathogens (Lidwell Citation1994; Beggs et al. Citation2000; Miller et al. Citation2002; CDC Citation2005; Kowalski Citation2009; NIOSH Citation2009; Reed Citation2010), such as Mycobacterium tuberculosis and influenza virus. The application of UVGI air disinfection is generally classified into in-duct UVGI (Ko et al. Citation2000; Peccia et al. Citation2001; Kowalski et al. Citation2003; Lai et al. Citation2004; Noakes et al. Citation2004b; McDevitt et al. Citation2007; Walker and Ko Citation2007; Rudnick et al. Citation2009; Ryan et al. Citation2010; Hwang et al. Citation2010) and upper-room UVGI (UR-UVGI; Riley and Permutt Citation1971; Beggs and Sleigh Citation2002; Ko et al. Citation2002; Xu et al. Citation2005). In-duct UVGI systems employ a bare UVC lamp to disinfect directly a large volume of air with high irradiance intensity. Because bacteria pass through the irradiated zone without recirculation, the in-duct UVGI system is often referred to as a single-pass UVGI system. UR-UVGI systems utilize a UVGI fixture at the upper part of the room and only irradiate the upper-room air. Generally, the UR-UVGI fixture is equipped with a multi-louvers damper in front of the UV lamp to generate collimated and parallel rays. Hence, UV rays do not irradiate the lower part of the room, minimizing human exposure to UVC rays. Studies based on the real and simulated clinical conditions have found that UR-UVGI air disinfection with air mixing efficiently reduced the transmission of Tuberculosis and Poxviruses (McDevitt et al. Citation2008; Escombe et al. Citation2009; Mphaphlele et al. Citation2015). Numerous public building rooms in China, such as classrooms and canteens for schools and kindergartens, have no central heating, ventilation, and air-conditioning systems. The application of UR-UVGI systems is one of the best control measures to prevent potential outbreaks of epidemic diseases in these buildings.
The efficiency of UR-UVGI air disinfection is not only affected by numerous environmental factors (e.g., airflow pattern, ceiling fan, relative humidity) but is also significantly dependent on the biological characteristics of the bacteria (e.g., airborne bacteria size, Z-value) (Noakes et al. Citation2006; Zhu et al. Citation2014; Kanaan et al. Citation2015; Yang et al. Citation2016). The Z-value, which is generally defined as the ratio of the inactivation rate normalized to the average irradiance, characterizes the susceptibility of airborne bacteria inactivated by UVGI. The Z-value obtained from the UR-UVGI is known as the effective Z-values (eZ-values; Beggs et al. Citation2006).
In general, the Z-values of airborne bacteria are evaluated with a single-pass UVGI experiment (Sharp Citation1940; Riley et al. Citation1976; Nakamura Citation1987; Ko et al. Citation2000; Peccia et al. Citation2001; VanOsdell and Foarde Citation2002; Lai et al. Citation2004; Noakes et al. Citation2004b; McDevitt et al. Citation2007; Walker and Ko Citation2007; Rudnick et al. Citation2015). In a single-pass UVGI experiment, a UVC lamp is located high above a vertically thin chamber through which contaminated air flows horizontally. Only the vertical rays of the UV radiation pass through the top surface of the chamber, which is composed of quartz. The floor of the chamber is flat and black minimizing UV reflections. Contaminated airflow behaves as a plug flow when bacteria are directly exposed to high irradiance within a narrow space and the UV dose (the product of the exposure time and irradiance) received by the bacteria can be precisely evaluated. Because the experimental conditions were simplified for artificial controlling, the Z-values of the bacteria obtained under these conditions are referred to as single-pass Z-values (sZ-values) herein.
For UR-UVGI air disinfection, bacteria are assumed to be well-mixed in the room and travel frequently through the upper irradiated zone, which accounts for a small fraction of the total room. Not all bacteria are exposed simultaneously, which may allow them sufficient time to repair their DNA, since bacteria have been known to be more resistant to UVC under UR-UVGI air disinfection (Peccia and Hernandez Citation2001; Beggs and Sleigh Citation2002; Beggs et al. Citation2006). Accordingly, the eZ-values, differing from the sZ-values (Beggs et al. Citation2006; Rudnick et al. Citation2015), are supplementary to the Z-value in the application of UR-UVGI. For example, the Z-value of M. parafortuitum has been reported to be 0.074 m2/J for sZ-value in a single-pass experiment (Rudnick et al. Citation2015), and 0.16 and 0.12 m2/J for sZ-value and eZ-value, respectively (Xu et al. Citation2003).
Recently, researchers have become increasingly interested in applying computational fluid dynamics (CFD) to model UR-UVGI air disinfection (Noakes et al. Citation2004a; Sung and Kato Citation2010, Citation2011; Yang et al. Citation2012; Gilkeson and Noakes Citation2013; Xu et al. Citation2013; Zhu et al. Citation2013; Kanaan et al. Citation2014; Pichurov et al. Citation2015). When we numerically investigate the performance of UR-UVGI, it is essential to consider using various eZ-values. The simulation based on the eZ-value is sufficient for characterizing the performance of UR-UVGI air disinfection (Yang et al. Citation2012). Xu et al. (Citation2003) conducted a full-scale UR-UVGI experiment to study the eZ-value of M. parafortuitum by adopting the obtained eZ-value to numerically investigate the effectiveness of UR-UVGI in hospital settings (Xu et al. Citation2013). However, the majority of the existing simulations for UR-UVGI are based on sZ-values (Noakes et al. Citation2006; Sung and Kato Citation2011; Kanaan et al. Citation2014, Citation2015; Pichurov et al. Citation2015). Therefore, more studies should be conducted to investigate the performance of UR-UVGI with eZ-values. It is necessary to further discuss the difference between both types of Z-values on the numerical simulation of bacteria inactivation (Gilkeson and Noakes Citation2013).
In the simulation, the UV inactivation rate is treated as the sink term of the bacteria transport equation for Eulerian approach. The sink term is the product of the eZ-value, the spatial irradiance, and the concentration of bacteria, representing the inactivation rate of UVGI on the airborne bacteria per unit time. Noakes et al. (Citation2004a) employed this simulation method to study the disinfection of airborne bacteria using two UR-UVGI fixtures in a displacement ventilated room. The numerical results were compared with the analytical solution of the two- and three-zone models. The group has shown that the simulation aptly describes the interaction of the room airflow with the UR-UVGI inactivation. Furthermore, Pichurov et al. (Citation2015) used this Eulerian method to evaluate the disinfection using two UR-UVGI fixtures with the inclusion of the mixing effect of a ceiling-mounted rotating fan. These researchers observed that the numerical results compared well with the experimental data.
The drift flux model is one of the most favorable applications of the Eulerian method for the simulations of bacteria/particles (Chen et al. Citation2006; Lai and Cheng Citation2007; Mui et al. et al. Citation2009; Zhao et al. Citation2009). The model assesses the bacteria/particle deposition onto walls by using the semi-empirical particle deposition model (Lai and Nazaroff Citation2000). Yang et al. (Citation2012) improved the drift flux model to include the inactivation rate of UVGI. The spatial irradiance of the UR-UVGI fixture was predicted with a view factor mathematical model that considered the shading effect of the louvers. These researchers applied this method to study the disinfection of E. coli and S. marcescens using one UR-UVGI fixture in a well-mixed ventilation scheme. Experiments were also conducted to validate the simulation model. Later, Yang et al. (Citation2016) expanded the view factor mathematical model to predict the fluence rate of multiple UR-UVGI fixtures and investigated the equivalent air exchange rate of multiple UR-UVGI fixtures on airborne bacteria for 3 and 6 ACH under well-mixed ventilation schemes.
In these models, the UV inactivation rate of bacteria was calculated per time step without considering the difference between exposure time and computational time. The inactivation rate is not only determined by the irradiance but also the exposure time. Bacteria spend more time in the irradiated zone and receive high UV doses. Consequently, the inactivation rate of UVGI on airborne bacteria should be evaluated with the exposure time. Some numerical studies (Noakes et al. Citation2006; Sung and Kato Citation2010, Citation2011; Gilkeson and Noakes Citation2013) treated the UV dose as a passive scalar and the irradiance was considered as the source term in the scalar transport equation. Then, the transport equation was solved to obtain the UV dose contour field. This method can display vividly the effect of airflow patterns on the distribution of UV dose, but few of the existing Eulerian approaches have incorporated the effects of exposure time on the UVGI inactivation sink term.
This study was conducted to determine experimentally the eZ-values of Serratia marcescens, Staphylococcus epidermidis, Pseudomonas alcaligenes, and Micrococcus luteus under exposure to UR-UVGI in a full-scale chamber. The drift flux model was modified and applied to investigate the performance of UR-UVGI under various ventilation rates with the obtained eZ-values. In the modification of the drift flux model, the UVGI inactivation rate set as the sink term was coupled with the bacteria residence time in the irradiated zone.
2. Materials and methods
In the decay method, airborne microorganisms were continuously injected into the room before switching on the UR-UVGI fixture. When the initial concentration of airborne bacteria stabilized, the room air was sampled with the UR-UVGI fixture in the off and on states, respectively. Subsequently, the decay rate of the concentration of airborne bacteria was used to calculate the eZ-value with the irradiance of the UR-UVGI fixture.
2.1. Experiments
In this study, all experiments were conducted in a mechanically ventilated, environmentally controlled chamber with a dimension of 2.25 × 2.3 × 2.3 m (x × y × w) (). The chamber was composed of stainless steel and had one door and no windows. An air supply diffuser and a return outlet were installed on the ceiling. The door was closed during the measurements.
2.1.1. Measurement of UV irradiance
A UR UVC germicidal fixture (TB-12-W, American Ultraviolet, Inc., Lebanon, IN, USA) was mounted on the north wall with the bottom surface of the fixture at a height of 1.96 m (). The fixture consisted of 37 × 16 × 11 cm3 (L × W × H) louvers with a 16 W UVC lamp.
The irradiance was measured using a radiometer (ILT1400, International Light Technologies, Inc., Technology Drive Peabody, MA, USA) equipped with a detector with a 254 nm peak (SEL/NS254/W). The UVC detector was fixed to a stand and clamped at a height of 2.05 m. For the measurements, the stand and clamp was positioned at 36 points (9 columns × 4 rows) in the chamber (). The UVC germicidal fixture was warmed up for 30 min before the measurements were performed. All chamber internal walls were covered with black paper and the lighting was turned off during the UVC measurements. The detector was factory-calibrated before the experiment and covered to calibrate the radiometer to a zero-reading before each measurement. Data were recorded when the radiometer showed a stable reading. Each measurement at each point was repeated at least three times.
2.1.2. Airborne bacteria generation and collection
S. marcescens (ATCC 6911), S. epidermidis (ATCC 12228), P. alcaligenes (ATCC 14909), and M. luteus (ATCC 4698) were supplied by the American Type Culture Collection (ATCC). The selected bacteria can be classified as gram-negative and rod-shaped (S. marcescens and P. alcaligenes) or gram-positive and coccus, spherical-shaped (M. luteus and S. epidermidis). The sizes of S. marcescens, P. alcaligenes, M. luteus, and S. epidermidis are 0.4–0.6 × 1.0 μm, 0.3–0.4 × 2.0 μm, 0.7 μm, and 0.8–0.9 μm, respectively. S. marcescens and S. epidermidis are commonly selected for UV inactivation experiments, because they are vulnerable to inactivation by UVC (Nakamura Citation1987; Ko et al. Citation2000; Peccia et al. Citation2001; VanOsdell and Foarde Citation2002). Both categories are known causes of hospital-acquired infections. For example, S. marcescens can cause respiratory tract infection, urinary tract infection, and infective endocarditis (Hejazi and Falkiner Citation1997). S. epidermidis can cause nosocomial infections, associated bloodstream infections, and cardiovascular infections (Vuong and Otto Citation2002). M. luteus was selected for this study because it is resistant to UVC and is considered a surrogate of pathogenic skin colonizers, whereas P. alcaligenes was selected as a surrogate of P. aeruginosa (Lai et al. Citation2016).
The incubation and harvesting methods have been described by Lai et al. (Citation2016). In brief, bacteria stock solutions preserved in a −80°C freezer were inoculated onto a nutrient agar (NA, Oxoid) or trypticase soy agar (TSA, Oxoid) plate and incubated at 37°C or 30°C for 24–48 h. The harvested bacteria were then transferred to 50 mL of sterilized distilled water in a 24-jet collison nebulizer (Collison Nebulizer, Bardays Global Investors, Inc., Waltham, MA, USA) for aerosolization. The inlet pressure of the nebulizer was 275.8 kPa and its outlet was connected to a 12 mm diameter copper tube. During the experiment, the nebulizer and pump were placed in a ventilated chamber adjacent to the test chamber. Aerosolized airborne bacteria were delivered at breathing level (1.68 m above the floor) into the center of the test chamber through the copper pipe.
A single-stage Andersen impactor (N6, Thermo Scientific, Inc., Franklin, MA, USA) located in the adjacent chamber was employed to sample the airborne bacteria concentration. An agar plate was placed inside the impactor, which was connected to a pump with a sampling flow rate of 28.3 L/min. Because it is unsafe to remain inside the chamber during the sampling of the bacteria, we controlled the sampling from outside the chamber. A 12 mm diameter copper pipe connected to the impactor was inserted through the testing chamber at the height of 2.05 m.
2.1.3. Procedure
For each type of airborne bacteria tested, the experimental procedure consisted of two stages: UR-UVGI fixture-off and UR-UVGI fixture-on. The ventilation system was turned off during all tests. All facilities and equipment were sterilized with 70% ethanol before each experiment. The nebulizer was sterilized by injecting compressed air to nebulize ethanol for 10 min. Next, we nebulized sterilized distilled water for 10 min to clean the nebulizer.
The UR-UVGI fixture-off experiment was conducted first. Prior to the injection of the airborne bacteria, air was sampled for 5 min to obtain the background airborne bacteria concentration. Next, the pump was switched off, and the agar plate was removed and replaced with a new one. A 50 mL bacteria solution was transferred into the nebulizer bottle. Bacteria were aerosolized into the test chamber for 15 min. During aerosolization, a portable household mixing fan (55 W) was used to achieve well-mixed initial conditions. When aerosolization was completed, air samples were collected 11 times (1 min/sampling). The time interval between sampling was 2 min (1 min for the first sample). If the bacteria were found to be contaminated, the sample was discarded. Measurements of bacteria were repeated a minimum of three times. When one set of measurements was completed, the ventilation system was turned on to exhaust the residual bacteria from the chamber before commencing the subsequent experiment.
The corresponding UR-UVGI fixture-on experiments were conducted immediately after the UR-UVGI fixture-off experiments. The UR-UVGI fixture was warmed up for at least 30 min before the experiment and was shielded with a cardboard cover to avoid exposing the UV dose to the bacteria during warm-up. The cover was attached to a rope that extended to the outside of the chamber. When the aerosolization process was completed, the cover was removed from outside the chamber by pulling on the rope. The aerosolization and sampling procedure was identical to that of the UR-UVGI fixture-off case.
Assuming the concentration of airborne bacteria was uniform in the control volume under well-mixed condition, the formula for calculating the surviving fraction of airborne bacteria exposed to UV irradiance is expressed as follows (Xu et al. Citation2003; Yang et al. Citation2016):[1] where C0 is the initial concentration of airborne bacteria, (CFU); Ci is the concentration of bacteria i at time t, (CFU); kn is the natural removal rate including natural die-off and deposition onto wall surfaces, (s−1); and kuv is the equivalent air-exchange rate of bacteria inactivated by the UR-UVGI system (s−1).
The value of kn was obtained when the UR-UVGI fixture was off, whereas that of (kn + kuv) was obtained when the UR-UVGI fixture was switched on. Subtracting kn from (kn + kuv) gives kuv. The eZ-value (eZ) can then be obtained as follows:[2] where IrV is the average irradiance of the irradiated zone (W/m2), which was calculated using the following equation (Yang et al. Citation2016):
[3] where Ir is the spatial irradiance (W/m2), which was predicted by using a view factor mathematical model introduced by Wu et al. (Citation2011), and VI is the volume of the irradiated zone. In our experimental chamber, irradiance was mainly detected in the w = 2.0–2.1 m zone and the values below w = 2.0 m were low and thus neglected. Hence, VI only contains the w = 2.0–2.1 m zone.
2.2. Simulation methodologies
Simulations were performed to investigate the effect of ventilation rate on the disinfection performance of the UR-UVGI system. The drift flux model was modified to describe the inactivation process of the UR-UVGI on the airborne bacteria. The physical simulation model was based on the test chamber dimensions except that the door was not considered. The UR-UVGI fixture-off and -on cases were evaluated with 3, 6, and 10 air changes per hour (ACH), respectively. Since there is a lack of heat and humidity source in the test chamber, air temperature and relative humidity were considered constant and heat and moisture transfers were not included in the simulation model. The drift flux methodology was applied to simulate the bacteria transport. The UVGI sink term in bacteria transport equation was coupled with the bacteria residence time and it is written as[4] where Suv,i is the sink term for the bacteria i inactivation of UVGI, tr,uv is the bacteria residence time in the upper irradiated zone. tr,uv can be evaluated by the concept of air age. Air age, which is defined as the length of time recorded from the moment air enters the ventilated room, was used to predict the bacteria residence time (Sandberg and Sjöberg Citation1983; Li et al. Citation2003). The natural death of airborne bacteria can be influenced by the air temperature, relative humidity, and photoreactivation (Xu et al. Citation2005; Walker and Ko Citation2007). These effects on the airborne bacteria natural death rate require further investigation. The natural death rate of airborne bacteria is lower than the UVGI inactivation rate; thus, its effect was ignored to highlight the UVGI disinfection in the simulation (Noakes et al. Citation2004a; Gilkeson and Noakes Citation2013; Zhu et al. Citation2014). Therefore, the bacteria deposition sink term did not include the bacteria natural death rate in the simulation model. The simulation methodologies and the numerical procedure are presented in details in Section S1, “Simulation methodologies,” in the online supplementary information (SI).
3. Results and discussion
3.1. Predicted irradiance (Ir) of the UR-UVGI fixture system
Figure S1 in the SI shows a comparison of the measured and predicted irradiance (Ir) of the UR-UVGI fixture. The irradiances distributed by four rows at different y locations are drawn independently in the four-branch figure. The UR-UVGI fixture used in this experiment was purchased in 2009. The maximum irradiance of the UR-UVGI fixture used in Wu et al. (Citation2011) was approximately 1.5 W/m2, at a distance of 0.8 m from the fixture (Case 2). In this study, the maximum irradiance was approximately 1 W/m2 (y = 1.5 m in ). The UVC output drop in the UR-UVGI fixture was considered acceptable. The maximum value was consistently high at the center location (x = 1.15 m) of the UR-UVGI fixture and symmetrically decreased along both sides of the UR-UVGI fixture (Figure S1). The maximum difference between the predicted and measured values was observed at the furthest location (y = 1.0 and 0.5 m) where the values were low and the measurement error was difficult to control. The average irradiance in the irradiated zone (IrV) calculated by Equation (Equation3[3] ) was 0.284 W/m2 and the average value measured at w = 2.05 m was 0.285 W/m2.
Figure 2. The eZ-value of S. marcescens (ATCC 6911). Each value is the average of three measured values and error bar represents the standard deviation.
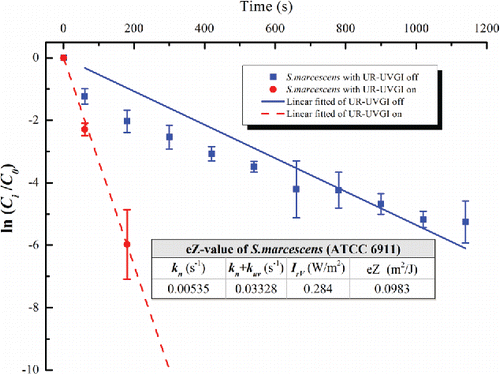
Figure 3. The eZ-value of S. epidermidis (ATCC 12228). Each value for UR-UVGI off and on is the average of four and three measured values, respectively. Error bar represents the standard deviation.
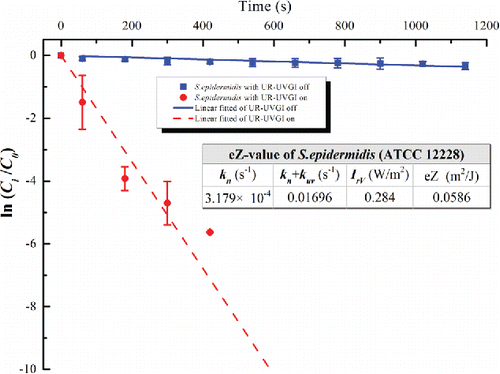
Figure 4. The eZ-value of P. alcaligenes (ATCC 14909). Each value for UR-UVGI off and on is the average of two and three measured values, respectively. Error bar represents the standard deviation.
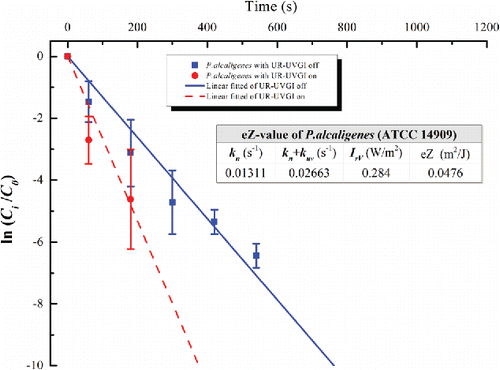
According to the manual of the TB series UVC fixture provided by American Ultraviolet Inc., there is no ozone by-product or other secondary contaminants during its operation. During experimental measurement, we used the Model 205 Dual Beam Ozone Monitor (2B Technologies, Inc., Boulder, CO, USA) to detect the ozone produced by the TB-12-W in the middle of the chamber. The background concentration in the test chamber was 6 parts per billion (ppb) during ventilation and with the UR-UVGI fixture off, while the average value was still 6 ppb for 1 h with the ventilation off and the UR-UVGI fixture on. Consequently, the contaminants produced by the TB-12-W can be ignored.
3.2. eZ-values of the tested bacteria
The eZ-values of the tested bacteria are displayed in –. Each figure shows the decay with a logarithmic function of a dimensionless concentration for the UR-UVGI fixture-off and -on cases, respectively. The corresponding decay rates (kn or kn+kuv) are the slopes of the best linear fitted lines. The results indicated that S. marcescens was the most sensitive to UVC disinfection among all the tested bacteria, whereas M. luteus was the least susceptible. The eZ-values of S. marcescens, S. epidermidis, P. alcaligenes, and M. luteus were 0.0983, 0.0586, 0.0476, and 0.0115 m2/J, respectively. The eZ-values from this study are listed with those reported in the literature (). The results show that the eZ-values obtained from the UR-UVGI system differ from those obtained with the single-pass UVGI systems. The eZ-values of S. marcescens from this study are similar to those reported by Yang et al. (Citation2012). One reason may be that both studies used the average irradiance of the irradiated zone to evaluate the eZ-values. According to Equation (Equation2[2] ), the average irradiance is a key factor for determining the eZ-value. If the average irradiance is defined using different volumes, different values may result for the same UR-UVGI fixture, rendering the eZ-values of the airborne bacteria calculated from different average irradiances incomparable. Xu et al. (Citation2003) recommended the use of the average irradiance of a room to evaluate the eZ-value. Specifically, the average irradiance was calculated from the average of the values measured in the upper-room. The calculation of the eZ-value is expressed as
[5] where hr is the height of the room and hi is the height of the irradiated zone. Beggs et al. (Citation2006) also suggested a similar calculation method and their method is derived from the relationship of the exposure time and the computational time:
[6]
Figure 5. The eZ-value of M. luteus (ATCC 4698). Each value is the average of four measured values and error bar represents the standard deviation.
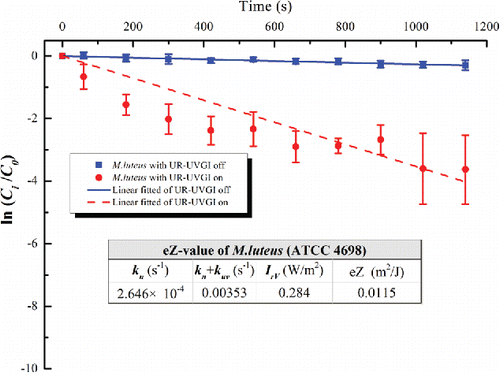
Table 1. The UV susceptibility constants (Z) of test bacteria reported in references Beggs et al. (Citation2006), Kowalski (Citation2009), Noakes et al. Citation(2004a), and Yang et al. (Citation2012).
Both approaches mentioned above use the ratio of the height, but the expressions are reciprocal. The eZ-values of the bacteria tested in this study were also evaluated with these methods and the results are presented in . According to the VI used in Equation (Equation2[2] ), hi is 0.1 m and it was determined by the distribution of Ir in the vertical direction, while hi in Xu et al.'s (Citation2003) and Beggs et al.'s (Citation2006) work was the height from the bottom surface of UR-UVGI fixture to the ceiling. According to Equations (Equation5
[5] ) and (Equation6
[6] ), if hi was evaluated from different method, the eZ-value will also be much different. Thus, to make the eZ-value from different works comparable is importance to keep consistent definition of the variables in the calculation of the eZ-value. The eZ-value obtained from both methods for a given tested bacteria can be considered as the lower and upper limits, respectively, e.g., 0.0043–2.2619 m2/J for S. marcescens. The eZ-value of S. marcescens reported by Beggs et al. (Citation2006) (in ) was obtained with Equation (Equation6
[6] ) and their kuv was evaluated by steady-state experiment conducted with continuous contamination source. Since their environment factors and the values of the variables in Equation (Equation6
[6] ) were different to present study, the eZ-value of S. marcescens was undoubtedly unequal for present study and Beggs et al. (Citation2006). To the best of our knowledge, the eZ-values for P. alcaligenes and M. luteus have never been reported.
Studies have shown that it is difficult to conduct UR-UVGI disinfection experiments because the disinfection can be influenced by many factors, such as, airflow pattern, relative humidity (RH), Z-value, irradiance field, and photoreactivation (Xu et al. Citation2005; Walker and Ko Citation2007). Although the eZ-values of tested bacteria were evaluated under zero ventilation and with illuminative lamps' switched off conditions, the results may still be affected by the bacteria mixing level, the measurement of irradiance, the location of the UR-UVGI fixture, and the relative humidity in our experimental system.
3.3. Effects of the ventilation rate on UR-UVGI disinfection
The inactivation of S. marcescens using UR-UVGI has been investigated in our recent study (Yang et al. Citation2012), wherein the concentration of S. marcescens was measured in all three locations on the w = 2.05 m plane under a well-mixed ventilation scheme with 2.9 ACH for UR-UVGI fixture-off and -on cases. To validate our present numerical method, we utilized the present eZ-value of S. marcescens, which is calculated from Equation (Equation2[2] ), to numerically simulate the identical case in the experiment by Yang et al. (Citation2012). The decay rate was obtained from linearly fitted lines of the average concentration of the three locations. displays the comparison of the kuv value obtained from the experiment in Yang et al. (Citation2012), the value predicted in this study, and the value predicted by Yang et al. (Citation2016). The results show that the kuv obtained using the present eZ-value is lower than the experimental value, while the value obtained in Yang et al. (Citation2016) is higher. The error is acceptable and is likely caused by measurement uncertainty. The sink term in this article considers the difference between the exposure time and computational time, while the eZ-value obtained from the experiment doses not (Equation (Equation1
[1] )). The results also demonstrate the challenge to accurately evaluate the exposure time for airborne bacteria exposed to UR-UVGI under turbulence airflow pattern. Although Beggs et al. (Citation2006) have presented the relationship between the exposure time and computational time, the results are based on plug flow in the room and the simple relationship is not appropriate for the actual complex airflow pattern. Therefore, the eZ-value obtained from Beggs' method is significantly higher. Undoubtedly, the kuv obtained with this eZ-value will significantly exceed the experimental value.
Figure 6. Comparisons of the present simulation and the experiment of Yang et al. (Citation2012) and the simulation of Yang et al. (Citation2016). The inactivation rate (kuv) of experiment is the difference of the slopes derived from “Ventilation” and “Ventilation + UR-UVGI.” Each point value is the average values of location A, B, and C at w = 2.05 m (Figures 5(d) and 6(d) in Yang et al. Citation2012). Error bar represents the standard deviation.
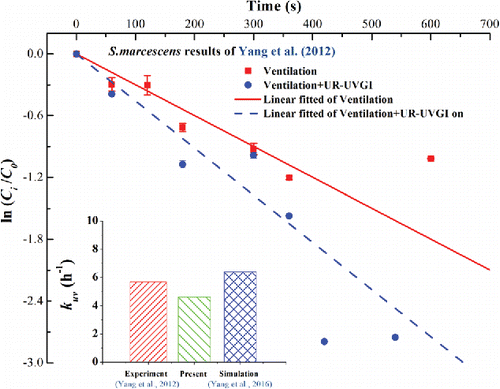
Simulations were carried out to investigate the UR-UVGI performance with the obtained eZ-values under different ACH cases. The inlet was simulated as four-way ceiling diffuser and the airflow pattern can be found in Figure S2 in the SI. In a well-mixed ventilation scheme, fresh air enters from the ceiling diffuser inlet and the airborne bacteria in the room follow the carrier fluid and finally flow out of the room through the ceiling outlet. The UR-UVGI fixture installed near the ceiling outlet is more likely to inactivate bacteria (Yang et al. Citation2016). Figure S3 in supplementary file shows the bacteria residence time (tr) characterized by air age for different ACH cases at t = 720 s. It is clear that tr decreases with the increase of ACH. When air velocity is enforced, air will easily flow out through the outlet, resulting in a low residence time. When the ACH is 6, air wanders and follows the vortex below the center of the diffuser where tr is high. Figures S4 and S5 in the SI show the overlapped contours of the concentration of s. marcescens (Cs. marcescens) and the inactivation rate (Suv,s. marcescens) at t = 720 s for different ACH cases. As described in Equation (Equation4[4] ), Suv,s. marcescens denotes the fraction of bacteria inactivated by UR-UVGI per unit time. Cs. marcescens is represented with colors and Suv,s. marcescens is represented with black lines and values in Figures S4 and S5. The highest value of Suv,s. marcescens is always found in the 3 ACH case (Figures S4a and S5a) because the bacteria residence time is high in low ACH cases and the value of Suv,s. marcescens decreases with increasing ACH. Accordingly, low Cs. marcescens occurs in the zone where Suv,s. marcescens is high. Although the inactivation rate by UVGI is low in high ACH cases, the maximum value for Cs. marcescens is low in these cases because the elimination of ventilation has compensated the reduction of Suv,s. marcescens.
In Figure S6 in the SI, the horizontal bars display a comparison of Puv, Pv, Pd, and Ps for different bacteria at 3, 6, and 10 ACH for the UR-UVGI fixture-off and -on cases. Puv, Pv, Pd, and Ps are the percentages of bacteria inactivated by UVGI inactivation, removed by ventilation, subsided by deposition, and those still suspended in air, respectively. Their calculations are found using Equations (S4) and (S5) in Section S1 in the SI. As shown in Figure S6a, Puv decreased in the order of S. marcescens, S. epidermidis, P. alcaligenes, and M. luteus, whereas Pv just increased accordingly. S. marcescens was the easiest to be inactivated by UR-UVGI, whereas M. luteus was the most difficult. When the UR-UVGI was switched off with a ventilation rate of 3 ACH (Figure S6d), ventilation became the leading removal mechanism and Pv exhibited little difference among the tested bacteria. Because the size difference between the tested bacteria was insignificant and Pd mainly depended on the gravitational settling, Pd did not notably change in either the UR-UVGI fixture-off or -on cases. For a given type of bacteria, Puv decreased as the ventilation rate increased; the Puv for S. marcescens was 36.4%, 29.6%, and 24.6% with 3, 6, and 10 ACH, respectively. Although Puv was the lowest in the 10 ACH case (Figure S6c), Pv compensated for the reduction of Puv, leading to the lowest suspended bacteria fraction (Ps) in this case. shows the average concentration of the tested bacteria in the breathing zone (Cb) for the various ACH cases. As shown in the figure, Cb nearly overlapped into a single trend line for the different tested bacteria in the UR-UVGI fixture-off cases for different ACH conditions. This implied that the average concentration of the tested bacteria in the breathing zone (Cb) removed by ventilation was identical for all bacteria. This agreed with the observed Pv in the UR-UVGI fixture-off case (Figure S6). With 3 ACH, the Cb value of S. marcescens was the lowest (approximately 0.25 at 720 s). The instantaneous Cb increased because the bacteria eZ-value was augmented during specific ACH cases; the increase in the different bacteria lessened when the ventilation rate rises.
Figure 7. The average concentration of the tested bacteria in breathing zone (w ≤ 1.68 m) (Cb) with IrV = 0.284 W/m2 for different ACHs.
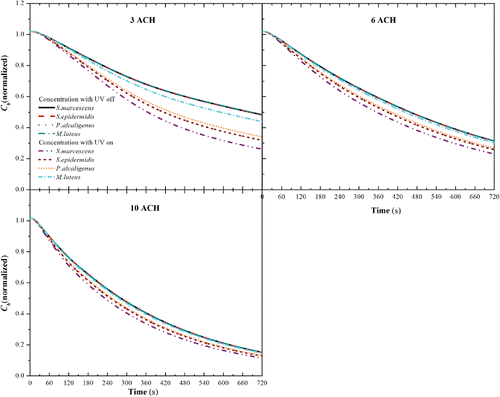
In our simulation model, one of the limitations was that the deposition of the rod-shaped airborne bacteria was calculated as the spherically shaped bacteria and the natural death rate was not considered. These factors will complicate the UR-UVGI disinfection analysis. To simplify these factors, we focused on the UR-UVGI disinfection of these airborne bacteria. However, the disinfection efficiency was different if the natural death rate of airborne bacteria was high.
Previous studies have demonstrated that UR-UVGI disinfection under a uniform irradiance field was less influenced by the airflow pattern (Xu et al. Citation2003; Yang et al. Citation2016). Simulations should be conducted with a uniform irradiance field. However, in this study, the UR-UVGI fixture required fixed settings for the experimental conditions, hence, uniform irradiance produced by multiple UR-UVGI fixtures were not performed. For non-uniform irradiance fields, the airflow pattern is a key factor that affects the UV dose received by bacteria. This subject is an interesting topic for further investigation of the UR-UVGI performance with other ventilation schemes, such as displacement ventilation and symmetrical airflow pattern.
4. Conclusions
The Z-value obtained from the UR-UVGI experiment is referred to as the effective Z-value (eZ-value), which is different from the single-pass Z-value (sZ-value). Although the air disinfection capabilities of UR-UVGI have been recognized, the eZ-value is not commonly reported in the literature. In this study, we selected four types of bacteria that are commonly used in laboratory tests. The bacteria's eZ-values were evaluated by performing experiment in a full-scale chamber with one UR-UVGI fixture installed on a wall. In the evaluation of the eZ-value, the irradiance of the UR-UVGI fixture was predicted with a view factor mathematical model and compared with the measured value at first. Then, the average irradiance of the irradiated zone was integrated with the spatial irradiance of the upper irradiated zone. The eZ-value was calculated with the average irradiance of the irradiated zone and the experimental inactivation rates. Simulation cases were performed with the obtained eZ-values to investigate the UR-UVGI disinfection on the tested bacteria under three different rates of ACH: 3, 6, and 10 h−1. The drift flux model was modified by considering the difference of the exposure time and computational time in the sink term of the bacteria transport equations.
The experimental results show that the eZ-values of S. marcescens, S. epidermidis, P. alcaligenes, and M. luteus were 0.0983, 0.0586, 0.0476, and 0.0115 m2/J, respectively. The eZ-values obtained from the present UR-UVGI experiment differ from those obtained from the single-pass UVGI experiments reported previously. The average irradiance is a key parameter for determining the eZ-values. The eZ-values calculated by the methods recommended by Beggs et al. (Citation2006) and Xu et al. (Citation2003) were also studied. Limited available evidence indicates that the eZ-values of one bacterium obtained with an identical definition of average irradiance in different experiments are similar. To keep consistent definition of the eZ-value is importance to make the results of different research works comparable. The simulation results in this study further show that higher fractions of bacteria can be inactivated by UR-UVGI under a low ventilation rate. The average concentration of airborne bacteria in the breathing zone (w ≤ 1.68 m) can be significantly reduced by the UR-UVGI system under low ventilation rate (3ACH). Future development of a comprehensive database of the eZ-values acquired from UR-UVGI experiments is critical for the emerging application of UR-UVGI systems in airborne pathogen control disinfection.
UAST_1334108_Supplementary_File.zip
Download Zip (1.8 MB)Acknowledgments
The authors sincerely thank Professor Dan Norbäck from Uppsala University in Sweden for improving the manuscript.
Funding
The work described in this article was partially supported by a grant from the Research Grants Council of the Hong Kong Special Administrative Region, China [grant number CityU 115213].
References
- Beggs, C. B., Kerr, K. G., Donnelly, J. K., Sleigh, P. A., Mara, D. D., and Cairns, G. (2000). A Engineering Approach to the Control of Mycobacterium Tuberculosis and Other Airborne Pathogens: A UK Hospital Based Pilot Study. Trans. Roy. Soc. Trop. Med. Hyg., 94:141–146.
- Beggs, C. B., Noakes, C. J., Sleigh, P. A., Fletcher, L. A., and Kerr, K. G. (2006). Methodology for Determining the Susceptibility of Airborne Microorganisms to Irradiation by an Upper-Room UVGI System. J. Aerosol Sci., 37:885–902.
- Beggs, C. B., and Sleigh, P. A. (2002). A Quantitative Method for Evaluating the Germicidal Effect of Upper Room UV Fields. J. Aerosol Sci., 33:1681–1699.
- CDC. (2005). Guidelines for Preventing the Transmission of Mycobacterium Tuberculosis in Health-Care Settings. Centers for Diseases Control and Prevention, Morbidity and Mortality Weekly Report, CDC, MMWR2005; 54 (No.RR-17), pp. 1–141. WHO, Geneva, Switzerland.
- Chen, F., Yu, S. C. M., and Lai, A. C. K. (2006). Modeling Particle Distribution and Deposition in Indoor Environments with a New Drift-Flux Model. Atmos. Environ., 40:357–367.
- Escombe, A. R., Moore, D. A., Gilman, R. H., Navincopa, M., Ticona, E., Mitchell, B., Noakes, C., Martínez, C., Sheen, P., Ramirez, R., Quino, W., Gonzalez, A., Friedland, J. S., and Evans, C. A. (2009). Upper-Room Ultraviolet Light and Negative Air Ionization to Prevent Tuberculosis Transmission. PLos Med., 6(3):e43.
- Gilkeson, C. A., and Noakes, C. J. (2013). Application of CFD Simulation to Predicting Upper-Room UVGI Effectiveness. Photochem. Photobiol., 89:799–810.
- Hejazi, A., and Falkiner, F. R. (1997). Serratia Marcescens. J. Med. Microbiol., 46:903–912.
- Hwang, G. B., Jung, J. H., Jeong, T. G., and Lee, B. U. (2010). Effect of Hybrid UV-Thermal Energy Stimuli on Inactivation of S. Epidermidis and B. Subtilis Bacterial Bioaerosols. Sci. Total Environ., 408:5903–5909.
- Kanaan, M., Ghaddar, N., Ghali, K., and Araj, G. (2014). New Airborne Pathogen Transport Model for Upper-Room UVGI Spaces Conditioned by Chilled Ceiling and Mixed Displacement Ventilation: Enhancing Air Quality and Energy Performance. Energ. Convers. Manage., 85:50–16.
- Kanaan, M., Ghaddar, N., Kamel, G., and Araj, G. (2015). Upper Room UVGI Effectiveness with Dispersed Pathogens at Different Droplet Sizes in Spaces Conditioned by Chilled Ceiling and Mixed Displacement Ventilation System. Build. Environ., 87:117–128.
- Ko, G., First, M. W., and Burge, H. A. (2000). Influence of Relative Humidity on Particle Size and UV Sensitivity of Serratia Marcescens and Mycobacterium Bovis BCG Aerosols. Tuber. Lung Dis., 80:4–5.
- Ko, G., First, M. W., and Burge, H. A. (2002). The Characterization of Upper-Room Ultraviolet Germicidal Irradiation in Inactivating Airborne Microorganisms. Environ. Health Perspect., 110:95–101.
- Kowalski, W. J. (2009). Ultraviolet Germicidal Irradiation Handbook: UVGI for Air and Surface Disinfection. Springer, Heidelberg, Germany.
- Kowalski, W. J., Bahnfleth, W. P., and Rosenberger, J. L. (2003). Dimensional Analysis of UVGI Air Disinfection Systems. HVAC&R Res., 9:347–362.
- Lai, A. C. K., and Cheng, Y. C. (2007). Study of Expiratory Droplet Dispersion and Transport Using a New Eulerian Modeling Approach. Atmos. Environ., 41:7473–7484.
- Lai, A. C. K., Cheung, A. C. T., Wong, M. M. L., and Li, W. S. (2016). Evaluation of Cold Plasma Inactivation Efficacy Against Different Airborne Bacteria in Ventilation Duct Flow. Build. Environ., 98:39–46.
- Lai, A. C. K., and Nazaroff, W. W. (2000). Modeling Indoor Particle Deposition from Turbulent Flow onto Smooth Surfaces. J. Aerosol Sci., 31:463–476.
- Lai, K. M., Burge, H. A., and First, M. W. (2004). Size and UV Germicidal Irradiation Susceptibility of Serratia Marcescens When Aerosolized from Different Suspending Media. Appl. Environ. Microb., 70:2021–2027.
- Li, X., Li, D., Yang, X., and Yang, J. (2003). Total Air Age: An Extension of the Air Age Concept. Build. Environ., 38:1263–1269.
- Lidwell, O. M. (1994). Ultraviolet Radiation and the Control of Airborne Contamination in the Operating Room. J. Hosp. Infect., 28:245–248.
- McDevitt, J. J., Lai, K. M., Rudnick, S. N., Houseman, E. A., First, M. W., and Milton, D. K. (2007). Characterization of UVC Light Sensitivity of Vaccinia Virus. Appl. Environ. Microb., 73(8):5760–5766.
- McDevitt, J. J., Milton, D. K., Rudnick, S. N., and First, M. W. (2008). Inactivation of Poxviruses by Upper-Room UVC Light in a Simulated Hospital Room Environment. PLos Med., 3:e3186.
- Miller, S. L., Hernandez, M., Fennelly, K., Martyny, J., Macher, J., Kujundzic, E., Xu, P., Fabian, P., Peccia, J., and Howard, C. (2002). Efficacy of Ultraviolet Irradiation in Controlling the Spread of Tuberculosis. Final Report, Contract no. 200-97-2602; NTIS No. PB2003-103816. U.S. Department of Health and Human Services, Centers for Disease Control and Prevention, National Institute for Occupational Safety and health, Cincinnati, OH. Available at: www.cdc.gov/niosh/reports/contract/pdfs/ultrairrTB.pdf
- Mphaphlele, M., Dharmadhikari, A. S., Jensen, P. A., Rudnick, S. N., Reenen, T. H. V., Pagano, M. A., Leuschner, W., Sears, T. A., Milonova, S. P., Walt, M. V. D., Stoltz, A. C., Weyer, K., and Nardell, E. A. (2015). Institutional Tuberculosis Transmission: Controlled Trial of Upper Room Ultraviolet Air Disinfection: A Basis for New Dosing Guidelines. Am. J. Respir. Crit. Care Med., 192(4):477–484.
- Mui, K. W., Wong, L. T., Wu, C. L., and Lai, A. C. K. (2009). Numerical Modeling of Exhaled Droplet Nuclei Dispersion and Mixing in Indoor Environments. J. Hazard. Mater., 167:736–744.
- Nakamura, H. (1987). Sterilization Efficacy of Ultraviolet Irradiation on Microbial Aerosols Under Dynamic Airflow by Experimental Air Conditioning Systems. Bull. Tokyo Med. Dental Univ., 34(2):25–40.
- NIOSH. (2009). Environmental Control for Tuberculosis: Basic Upper-Room Ultraviolet Germicidal Irradiation Guidelines for Healthcare Settings. DHHS(NIOSH) Publication No. 2009 – 105. Department of Health and Human Services, Centers for Disease Control and Prevention, National Institute for Occupational Safety and Health, Atlanda, GA.
- Noakes, C. J., Beggs, C. B., and Sleigh, P. A. (2004a). Modelling the Performance of Upper Room Ultraviolet Germicidal Irradiation Devices in Ventilated Rooms: Comparison of Analytical and CFD Methods. Indoor Built Environ., 13:477–488.
- Noakes, C. J., Fletcher, L. A., Beggs, C. B., Sleigh, P. A., and Kerr, K. G. (2004b). Development of a Numerical Model to Simulate the Biological Inactivation of Airborne Microorganisms in the Presence of Ultraviolet Light. J. Aerosol Sci., 35:489–507.
- Noakes, C. J., Sleigh, P. A., Fletcher, L. A., and Beggs, C. B. (2006). Use of CFD Modeling to Optimise the Design of Upper-Room UVGI Disinfection Systems for Ventilated Rooms. Indoor Built Environ., 15:347–356.
- Peccia, J., and Hernandez, M. T. (2001). Photoreactivation in Airborne Mycobacterium Parafortuitum. Appl. Environ. Microb., 67:4225–4232.
- Peccia, J., Werth, H. M., Miller, S., and Hernandez, M. (2001). Effects of Relative Humidity on the Ultraviolet Induced Inactivation of Airborne Bacteria. Aerosol Sci. Tech., 35:728–740.
- Pichurov, G., Srebric, J., Zhu, S., Vincent, R. L., Brickner, P. W., and Rudnick, S. N. (2015). A Validated Numerical Investigation of the Ceiling Fan's Role in the Upper-Room UVGI Efficacy. Build. Environ., 86:109–119.
- Reed, N. G. (2010). The History of Ultraviolet Germicidal Irradiation for Air Disinfection. Public Health Rep., 125:15–27.
- Riley, R. L., Knight, M., and Middlebrook, G. (1976). Ultraviolet Susceptibility of BCG and Virulent Tubercle Bacilli. Am. Rev. Respir. Dis., 113:413–418.
- Riley, R. L., and Permutt, S. (1971). Room Air Disinfection by Ultraviolet Irradiation of Upper Air: Air Mixing and Germicidal Effectiveness. Arch. Environ. Health, 22:208–219.
- Rudnick, S. N., First, M. W., Vincent, R. L., and Brickner, P. W. (2009). In-Place Testing of In-Duct Ultraviolet Germicidal Irradiation. HVAC&R Res., 15:525–535.
- Rudnick, S. N., McDevitt, J. J., Hunt, G. M., Stawnychy, M. T., Vincent, R. L., and Brickner, P. W. (2015). Influence of Ceiling Fan's Speed and Direction on Efficacy of Upper-Room Ultraviolet Germicidal Irradiation-Part: Experimental. Build. Environ., 92:756–763.
- Ryan, K., McCabe, K., Clements, N., Hernandez, M., and Miller, S. L. (2010). Inactivation of Airborne Microorganisms Using Novel Ultraviolet Radiation Sources in Reflective Flow-Through Control Devices. Aerosol Sci. Tech., 44:541–550.
- Sandberg, M., and Sjöberg, M. (1983). The Use of Moments for Assessing Air Quality in Ventilated Rooms. Build. Environ., 18(4):181–197.
- Sharp, D. G. (1940). The Effects of Ultraviolet Light on Bacteria Suspended in Air. J. Bacteriol., 39:535–547.
- Sung, M., and Kato, S. (2010). Method to Evaluate UV Dose of Upper-Room UVGI System Using the Concept of Ventilation Efficiency. Build. Environ., 45:1626–1631.
- Sung, M., and Kato, S. (2011). Estimating the Germicidal Effect of Upper-Room UVGI System on Exhaled Air of Patients Based on Ventilation Efficiency. Build. Environ., 46:2326–2332.
- VanOsdell, D., and Foarde, K. (2002). Defining the Effectiveness of UV Lamps Installed in Circulating Air Ductwork. Report No. 2002, ARTI-21CR/610-40030-01. Air-Conditioning and Refrigeration Technology Institute, Arlington, VA.
- Vuong, C., and Otto, M. (2002). Staphylococcus Epidermidis Infections. Microbes Infect., 4:481–489.
- Walker, C. M., and Ko, G. (2007). Effect of Ultraviolet Germicidal Irradiation on Viral Aerosols. Environ. Sci. Technol., 41:5460–5465.
- Wu, C. L., Yang, Y., Wong, S. L., and Lai, A. C. K. (2011). A New Mathematical Model for Irradiance Field Prediction of Upper-Room Ultraviolet Germicidal Systems. J. Hazard. Mater., 189:173–185.
- Xu, P., Fisher, N., and Miller, S. L. (2013). Using Computational Fluid Dynamics Modeling to Evaluate the Design of Hospital Ultraviolet Germicidal Irradiation Systems for Inactivating Airborne Mycobacteria. Photochem. Photobiol., 89:792–798.
- Xu, P., Kujundzic, E., Peccia, J., Schafer, M., Moss, G., Hernandez, M., and Miller, S. L. (2005). Impact of Environmental Factors on Efficacy of Upper-Room Air Ultraviolet Irradiation for Inactivating Airborne Mycobacteria. Environ. Sci. Technol., 39:9656–9664.
- Xu, P., Peccia, J., Fabian, P., Martyny, J. W., Fennelly, K. P., Hernandez, M., and Miller, S. L. (2003). Efficacy of Ultraviolet Germicidal Irradiation of Upper-Room Air in Inactivating Airborne Bacterial Spores and Mycobacteria in Full-Scale Studies. Atmos. Environ., 37:405–419.
- Yang, Y., Chan, W. Y., Wu, C. L., Kong, R. Y. C., and Lai, A. C. K. (2012). Minimizing the Exposure of Airborne Pathogens by Upper-Room Ultraviolet Germicidal Irradiation: An Experimental and Numerical Study. J. R. Soc. Interface, 9:3184–3195.
- Yang, Y., Lai, A. C. K., and Wu, C. (2016). Study on the Disinfection Efficiency of Multiple Upper-Room Ultraviolet Germicidal Fixtures System on Airborne Microorganisms. Build. Environ., 103:117–128.
- Zhao, B., Chen, C., and Tan, Z. (2009). Modeling of Ultrafine Particle Dispersion in Indoor Environments with an Improved Drift Flux Model. J. Aerosol. Sci., 40:29–43.
- Zhu, S., Srebric, J., Rudnick, S. N., Vincent, R. L., and Nardell, E. A. (2014). Numerical Modeling of Indoor Environment with a Ceiling Fan and an Upper-Room Ultraviolet Germicidal Irradiation System. Build. Environ., 72:116–124.
- Zhu, S. W., Srebric, J., Rudnick, S. N., Vincent, R. L., and Nardell, E. A. (2013). Numerical Investigation of Upper-Room UVGI Disinfection Efficacy in an Environmental Chamber with a Ceiling Fan. Photochem. Photobiol., 89:782–791.