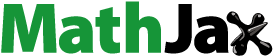
ABSTRACT
Understanding the mixing behavior of anthropogenic primary and biogenic secondary organic aerosol (POA and SOA) is important for characterizing their interactions with water vapor. The following work expands upon previous studies and investigates cloud condensation nuclei (CCN) activity and droplet kinetics of α-pinene SOA formed in an environmental chamber and mixed with diesel or motor oil-diesel fuel POA. The changes in the aerosol mixing are similar to previously published work but this study provides new CCN activity and droplet information. The CCN activity of the unmixed aerosol systems are measured separately; κ = 0.15, 0.11, 0.022 for α-pinene SOA, diesel POA and motor oil-diesel fuel POA, respectively. In the α-pinene SOA + diesel POA mixture, the CCN activity, characterized by κ-hygroscopicity, decreases from κ = 0.15 to 0.06 after an initial injection of the POA but increases to κ = 0.12. The increase in CCN activity occurs after particle collision (coagulation and wall-loss) rates dominate aerosol processes in the chamber. The α-pinene SOA + motor oil-fuel POA does not readily mix and the CCN activity of the complex system increases with time (from κ = 0.022 to 0.10). An empirical equation using unit mass resolution (UMR) AMS data of two different ion fragments reasonably predicts CCN activity of the POA and SOA mixtures. CCN measurement may be a promising tool to gain additional insight into the complex mixtures of organic aerosol and subsequent interactions with water vapor.
Copyright © 2018 American Association for Aerosol Research
EDITOR:
1. Introduction
Ambient aerosols are complex mixtures. Traditionally defined, the mixtures formed may be internal, external or core-shell structures. In internal mixtures, the aerosol is homogeneously mixed to form a single component. External mixtures form from non-homogenous agglomerates of aerosol components. Core-shell mixtures are formed when organic and inorganic species condense onto a black carbon core (Jacobson Citation2001, Moffet and Prather Citation2009). The formation of aerosol mixtures from secondary organic plus primary organic sources is influenced by miscibility, condensability, polarity and entropy (Asa-Awuku et al. Citation2009, Vaden et al. Citation2010). Vaden et al. (Citation2010) showed that secondary organic (SOA) can condense onto hydrophobic primary organic aerosol (POA) and form metastable coated shells.
Asa-Awuku et al. (Citation2009) evaluated the formation of mixtures from SOA, diesel POA, and motor oil-diesel fuel POA. They found more volatile diesel POA and α-pinene SOA formed internal mixtures or core-shell structures (“strong phase” mixtures) whiles β-caryophyllene SOA and motor oil fuel POA formed an external-like (“weak phase”) mixture. The differences in mixing state were evident but did not adhere to traditional definitions. Asa-Awuku et al. (Citation2009) attributed the formation of internal mixtures to readily entropically mixed systems. Our knowledge of mixing state has since evolved to include aerosol viscosity and phase information. The use of the terms weak and strong phase mixtures, employed in Asa-Awuku et al. (Citation2009) is not indicative of the viscous phase states or phase-state terminology employed in the description of aerosol physical properties reported elsewhere (Saukko et al. Citation2012, Lu et al. Citation2014). Yet understanding the propensity of unspeciated organic aerosol to mix is not well known and the ability to characterize transient mixing states of complex organic mixtures is challenging. Thus simplifying assumptions are applied to understand the subsequent ability of the mixed aerosol to uptake-water and form droplets.
The cloud condensation nuclei (CCN) activity of unmixed POA and SOA is relatively well studied (Petters and Kreidenweis Citation2007, Cubison et al. Citation2008, Wang et al. Citation2010, Lambe et al. Citation2011). Freshly emitted soot is only slightly hygroscopic but can become more CCN active due to oxidation and condensation of hydrophilic co-emitted vapors (Khalizov et al. Citation2013). In SOA, there is a direct correlation between the oxidized fraction and the CCN κ-hygroscopicity in ambient and smog chamber datasets (Jimenez et al. Citation2009, Massoli et al. Citation2010). However, when POA and SOA are mixed, predicting the CCN activity becomes more challenging (Nenes and Seinfeld Citation2003, King et al. Citation2007). Wang et al. (Citation2010) observed a rapid mixing of ambient urban non-hygroscopic POA and black carbon (BC) with photochemically aged organic aerosol during the MILAGRO field campaign. CCN activity increased in the subsequent mixtures and κ-hygroscopicity increased from 0 to 0.1. CCN activity was found to be sensitive to the mixing states, especially when non-hygroscopic species (κ < 0.1) contributed significantly to the aerosol volume fraction.
In this study, we explore the CCN activity and droplet formation of mixtures of α-pinene biogenic SOA plus diesel primary organic aerosol (DL POA) as well as α-pinene SOA plus motor-oil and diesel fuel primary organic aerosol (MOF POA). We consider the extent of mixing, if any, on the CCN activity. We further discuss changes in the single parameter κ-hygroscopicity due to mixing the SOA and the POA. The subsequent changes in the droplet kinetics from mixing α-pinene biogenic SOA plus diesel primary organic aerosol (DL POA), as well as α-pinene SOA plus motor-oil fuel primary organic aerosol (MOF POA), is also investigated.
2. Experimental methods and instrumentation
The aerosol formation procedure is the same as Asa-Awuku et al. (Citation2009) and is briefly described here. The experiments were performed in a 12 Carnegie Mellon environmental smog chamber. Temperature and humidity are controlled (Presto et al. Citation2005). SOA was formed by dark ozonolysis of α-pinene precursors in the presence of excess ozone at 21°C and < 5% RH. After an hour, when all the SOA precursors are oxidized and significant SOA mass is formed, the POA is injected. A mixture of 1 mL motor oil and 5 μL diesel fuel was flash vaporized and injected into the smog chamber; the mixture is a controlled surrogate for unburnt diesel emissions (Weitkamp et al. Citation2008, Jathar et al. Citation2013). Depending on load and operating conditions, unburnt oil can make up to 95% of the total emissions from diesel engines (Sakurai et al. Citation2003). Unburnt diesel and lubricants in diesel engines produce organic-rich aerosol similar in composition from the flash vaporized surrogate (Fushimi et al. Citation2011). The aerosol formed from the flash vaporized mixture of motor oil and diesel fuel is hereafter referred to as MOF POA. In a different setup, soot is generated from local commercial diesel combusted in a 4.5 kW single-engine generator at low load. Diesel particle emissions contain black carbon, nitrates, polyaromatic hydrocarbons and volatile organic components (Lamarque et al. Citation2010, Gentner et al. Citation2012). The diesel soot from the generator is hereafter referred to as diesel “POA” (DL POA) and is injected into the smog chamber.
The aerosol chemical composition was measured with an Aerodyne Quadrupole Aerosol Mass Spectrometer (Q-AMS). The Q-AMS ion fragments (mass to charge ratio, ) are unit mass resolution (UMR) (Zhang et al. Citation2005). The Q-AMS also provides particle time-of-flight (PTOF) data to produce size-resolved chemical distributions (Figure S1). The contributions of POA and SOA can be observed with UMR data. It has been shown that the evolution of POA and SOA mixing in urban and rural sites can be demonstratively traced with the m/z 44 and m/z 57 ion fragments (McFiggans et al. Citation2005). From high resolution AMS data, m/z 44 has been observed to be a good tracer for predominantly oxygenated organic aerosol (OOA) such as α-pinene SOA, and m/z 57 for the hydrocarbon-like organic aerosol (HOA) from diesel and MOF POA (Song et al. Citation2007, Mohr et al. Citation2009). In this study, there is limited chemical information and we therefore use m/z 44 and m/z 57 as indicators of secondary and primary materials.
The aerosol size distribution was measured with a scanning mobility particle sizer (SMPS, TSI 3081) and a condensation particle counter (CPC, TSI 3772). A DMT cloud condensation nuclei counter (CCNc) was operated downstream of the SMPS and in parallel with the CPC. The theory and operation of the CCNc are thoroughly described elsewhere (Roberts and Nenes Citation2005, Rose et al. Citation2008). The size selected aerosol is sampled by the CCNc at a 0.5 lpm flowrate. The activated fraction (the CCN concentration divided by aerosol number concentration) is measured between supersaturations of 0.3 – 1.5% for different aerosol dry diameters. Scanning mobility CCN analysis (SMCA) is employed and calculates the critical activation diameters (dp50) for each supersaturation every 2.25 min (Moore et al. Citation2010). The CCNc is calibrated with (NH4)2SO4 aerosol for the same experimental supersaturations and flowrate following procedures outlined in (Rose, Gunthe et al. Citation2008) (Table, S1 Supplemental Information). In environmental smog chamber experiments, it is assumed that the composition of the aerosol in suspension is the same as that on the wall and thus the critical diameter is not modified by particle wall-loss. The CCN of DL POA, MOF POA, and α-pinene SOA are also measured independently in addition to the mixtures proposed above.
The CCN activity of aerosol can be characterized by the single-parameter Köhler theory hygroscopicity parameter, κ. κ-hygroscopicity is calculated for each dp50 and supersaturation and is dependent on chemical composition (Petters & Kreidenweis, Citation2007). The average final droplet diameters are measured for critical dry diameters (dp50). The critical dry diameter is the diameter at which more than 50% of the dry aerosol activates into CCN. The reported droplet diameter is the average of the final droplet diameters of the total aerosol activated fraction from Scanning mobility CCN analysis (SMCA) (Moore, Nenes et al. Citation2010). The impacts of mixing are assessed on droplet growth by using the threshold droplet growth analysis (TDGA) method (Asa-Awuku et al. Citation2008, Engelhart et al. Citation2008, Raatikainen et al. Citation2012). In TDGA the average droplet sizes of the mixture systems are compared to the droplet size of calibration (NH4)2SO4 aerosol at the same experimental conditions. Differences in droplet size indicate changes in droplet growth kinetics.
3. Results and discussion
3.1. CCN activity and droplet measurement
The α-pinene SOA formed from the dark ozonolysis experiments was unimodal with a peak size distribution between 200–300 nm. It showed uniform, size-independent chemical composition traced by the AMS signals m/z 43, 44, and 45. The m/z 44 was, however, the abundant ion fraction. Upon injecting the POA, all POA tracers increased. The increase in POA tracers indicates condensing volatile components from the vaporized motor-oil fuel and diesel POA on the SOA. The m/z 57 was the most abundant ion fragment in both the DL POA and the MOF POA systems. From previous thermodenuder studies, MOF POA and DL POA showed higher volatility than the α-pinene SOA (Asa-Awuku et al. Citation2009).
The CCN activity of the unmixed aerosol systems (α-pinene SOA, DL POA, and MOF POA) was measured. In , the α-pinene SOA was the most hygroscopic (κ = 0.15) of the three species. The κ-hygroscopicity of α-pinene SOA is consistent with previously published values (Engelhart et al. Citation2008). The MOF POA was the least hygroscopic (κ = 0.022 ± 0.002). The lower MOF POA hygroscopicity could be due to the presence of hydrophilic fractions from the unburnt motor oil. The diesel POA had a hygroscopicity closer to that of SOA (κ = 0.11 ± 0.03) likely due to the presence of significant amounts of oxidized organics. The similarity in the hygroscopicity of α-pinene SOA and DL POA could be attributed to a significant presence of m/z 44 ion, an indication of oxygenated organic components (Lambe, Onasch et al. Citation2011). It is noted that m/z 43 signals also indicate oxygenation and are found in all three systems. However, only m/z 44 signal can be found in abundance in the more hygroscopic α-pinene SOA and DL POA PTOF spectra (Asa-Awuku et al. Citation2009).
Figure 1. Measured CCN activity of α-pinene SOA (triangles [green], κ = 0.15), DL POA (squares [pink], κ = 0.11) and MOF POA (circles [yellow], κ = 0.022) compared with (NH4)2SO4 (κ = 0.6) and hypothetical insoluble but slightly wettable aerosol (κ = 0.0001).
![Figure 1. Measured CCN activity of α-pinene SOA (triangles [green], κ = 0.15), DL POA (squares [pink], κ = 0.11) and MOF POA (circles [yellow], κ = 0.022) compared with (NH4)2SO4 (κ = 0.6) and hypothetical insoluble but slightly wettable aerosol (κ = 0.0001).](/cms/asset/0ec8a349-1e44-42ab-99b8-f6d18ac093aa/uast_a_1392480_f0001_oc.gif)
The unimodal α-pinene SOA distribution was modified after injecting the DL POA and MOF POA (Figure S1). In the DL + α-pinene SOA experiment, a short-lived bimodal distribution was formed immediately after injecting the POA. The bimodal distribution, however, converged into a single distribution after ∼ 1 h. The α-pinene SOA and volatile components of the DL POA coagulate (due to the short residence time [Zhang and Wexler Citation2004]) and condense onto the black carbon fraction of the DL POA. The particle distribution shifts to larger sizes faster than the rate of coagulation alone (Figure S1). It is likely that the mixed aerosol forms a core of BC coated with a shell of well-mixed organic aerosol on the surface. The formation of core-shell mixtures is more plausible due to the limited miscibility of the black carbon fraction of the DL POA (Jacobson Citation2001, Moffet and Prather Citation2009). This is supported by the single particle distribution observed in both the AMS PToF and SMPS particle distribution (Figures S1a and S2a). And is also congruent with observations made during previous chamber and ambient studies of soot mixtures (Schnaiter et al. Citation2005, Wang, Cubison et al. Citation2010). In the MOF POA + α-pinene SOA experiment, two distinct PToF distributions were formed immediately after the MOF POA injection. The bimodal distribution persisted throughout the experiment (Figure S1b). This suggests an external-like mixture of MOF POA and α-pinene SOA (Cubison, Ervens et al. Citation2008, Wang, Cubison et al. Citation2010). This is consistent with previous observations of mixtures of hydrophobic POA with biogenic SOA (Song, Zaveri et al. Citation2007). More recently, light-scattering single-particle measurements (LSSP) on similar mixtures confirm that the MOF + SOA mixtures remain largely distinct for hours (particles form external-like mixtures, mixing depends on particle collisions) while DL – SOA mixtures show the limited exchange of marker fragments between the two populations (and form internal mixtures) (Robinson et al. Citation2016). The DL POA + α-pinene SOA experiment here form a readily mixed (RM) system and the MOF POA + α-pinene SOA do not readily mix (depending on coagulation alone).
In the α-pinene SOA plus diesel exhaust POA mixture experiments (), the CCN activity decreased immediately after the injection of the DL POA. The hygroscopicity of the mixture was significantly depressed (from κ = 0.15 to κ = 0 .06); below that of α-pinene SOA and DL POA. The nonlinear depression in κ values is likely due to the presence of less hygroscopic components in DL POA. In the first hour more volatile aerosol components of DL POA evaporate and condense (partition) on the larger α-pinene SOA (Figure S2). The resulting low volatility material has hygroscopicity ∼0.06. As the partitioning rate slows down, the physical processes of coagulation and wall-loss dominate changes in the particle number and size distribution. At this process determinate time (PDT), the mode electrical mobility particle diameter shifts less than 2 size bins per minute (Figure S2). PDT occurred 1 h after the DL POA injection and readily mixed aerosol formed. After PDT, there are little to no changes in the normalized size-resolved chemical composition of the mixed system (Asa-Awuku, Miracolo et al. Citation2009). Coagulation occurs after PDT for the α-pinene SOA + DL POA system and κ-hygroscopicity values increase to 0.12. In the α-pinene SOA plus motor oil-fuel POA experiments (), there was a rapid decrease in the CCN activity of the mixture when the MOF POA was injected (from κ = 0.15 to κ = 0.022). The α-pinene SOA + MOF POA system did not readily mix. Coagulation occurred after injection and two distinct chemically size-resolved populations were observed; indicative of an external-like mixture. Little to no partitioning occurred, and PDT was established immediately after injection of MOF POA., The CCN activity of the α-pinene SOA + MOF POA system increased after PDT (to κ = 0.10). Both cases (DL POA and MOF POA plus α-pinene SOA) showed a steady increase in CCN activity after PDT was established; 1 h after injection of the DL POA and immediately for MOF POA.
Figure 2. Measured CCN κ-hygroscopicity of (a) α-pinene SOA (squares [green]) and DL POA (filled circles) and (b) α-pinene SOA (squares [green]) and MOF POA (filled circles). In (a) the hygroscopicity decreases from the α-pinene SOA average (light [green] horizontal dashed line, κ = 0.15) when the DL MOF is injected but increases after 1 h to values above DL POA (dark [purple] horizontal purple dashed line). In (b) the injection of the MOF POA causes an instantaneous decrease in the hygroscopicity. Immediately after injection, hygroscopicity increases, within the average kappa-values of α-pinene SOA (light [green] horizontal dashed line) and MOF POA (bold [yellow] horizontal dashed line). Dashed vertical lines indicate the time of POA injection. The size of the markers represents the critical diameter.
![Figure 2. Measured CCN κ-hygroscopicity of (a) α-pinene SOA (squares [green]) and DL POA (filled circles) and (b) α-pinene SOA (squares [green]) and MOF POA (filled circles). In (a) the hygroscopicity decreases from the α-pinene SOA average (light [green] horizontal dashed line, κ = 0.15) when the DL MOF is injected but increases after 1 h to values above DL POA (dark [purple] horizontal purple dashed line). In (b) the injection of the MOF POA causes an instantaneous decrease in the hygroscopicity. Immediately after injection, hygroscopicity increases, within the average kappa-values of α-pinene SOA (light [green] horizontal dashed line) and MOF POA (bold [yellow] horizontal dashed line). Dashed vertical lines indicate the time of POA injection. The size of the markers represents the critical diameter.](/cms/asset/effc2906-b4c8-4f25-aac8-3ecca9ee2e9d/uast_a_1392480_f0002_oc.gif)
evaluates the effects of mixing on the droplet kinetics at 0.94% supersaturation and 0.5l pm CCNc flowrate. (Additional droplet information at other SS are presented in Figure S3). In all cases, the droplet diameter is evaluated at the critical particle diameter, dp50. The single system average droplet sizes are compared to the two-phase mixed system. The α-pinene SOA average droplet size is larger than those of both DL POA (
and MOF POA (
). The reported droplet diameters were corrected for supersaturation depletion (Asa-Awuku et al. Citation2011, Lathem and Nenes Citation2011). The difference in droplet sizes is within 1 (0.5 µm) and 2 (1 µm) bins for DL POA and MOF POA, respectively. α-pinene SOA and (NH4)2SO4
form comparable droplet sizes at the same SS and flowrate conditions. For the DL POA plus α-pinene SOA mixture, the droplet diameters 20 min after injection are reduced by ∼12% to sizes similar to that of the DL POA alone; at this time before PDT, internal mixing has not been attained (). Over time, the droplet diameter increases and converges towards the α-pinene SOA droplet size (within measurement uncertainty of the OPC). In the MOF POA plus α-pinene SOA system, the droplet diameter
is similar to the α-pinene SOA at the point of injection (). Overall the droplet diameters in the MOF POA plus α-pinene SOA did not vary significantly during the course of the experiment
).
3.2. CCN prediction of complex mixtures
The above observations of CCN activity suggest that (a) the organic aerosol water uptake is sensitive to the differences in the oxidized organic composition and (b) kappa values after PDT are consistent with volume additive mixtures of the SOA and POA. Here, we hypothesize and test an empirical equation to predict the CCN activity of mixtures derived from these observations. Previous work shows:
• | the oxidized, water-soluble fraction of complex organic aerosol (OA) is soluble and hygroscopic. OA can depress the surface tension of the activating droplet up to 10% (Moore et al. Citation2008, Ruehl et al. Citation2012). The | ||||
• | for multiple-component systems, a solubility influenced, volume additive approach reasonably estimates the κ-hygroscopicity (Petters and Kreidenweis Citation2008). | ||||
• | α-pinene SOA is mostly hygroscopic and soluble material (Engelhart, Citation2008). Thus the contribution of non-hygroscopic mass is injected with the POA. Moreover, the POA can be traced with the m/z 57 fragment ion (Alfarra et al. Citation2004, McFiggans, Alfarra et al. Citation2005, Canagaratna et al. Citation2010). |
If the above observations are true, then UMR data can predict CCN activity of the SOA and POA mixtures. We predict the experimental observations with the following empirical equations,[1]
[1]
[2]
[2]
Where is the relative molar contribution of the more hygroscopic, soluble organic component (m/z 44) to the non-hygroscopic but wettable organic component (m/z 57). This binary representation of the hygroscopicity may only be robust for mixed systems where a single fragment ion dominates an aerosol component. The use of fragment ion data for CCN activity prediction is empirical and is not derived from Köhler theory.
Here the volume additive hygroscopicity, is simplified into a binary mixture of the more hygroscopic soluble organics,
, and a less hygroscopic but wettable components with
. The value of the
is based on previous laboratory and modeling studies of fresh diesel emissions (Zaveri et al. Citation2010, Vu et al. Citation2015, Vu et al. Citation2017), values are above zero and within 0.001 order of magnitude. It is assumed that hygroscopic and non-hygroscopic OA is of similar density and thus molar ratios are equivalent to volume ratios. Therefore, the hygroscopicity can be represented by the chemcial tracers in lieu of the traditional volume fractions (Petters and Kreidenweis Citation2007).
In , κ-hygroscopicity values estimated from Equation [Equation2[2]
[2] ] are compared to measured CCN κ-hygroscopicity. The time series plots ( and ) show that the prediction follows the trends of the observed CCN activity. In the DL POA plus α-pinene SOA mixture, the modeled hygroscopicity captures the non-linearity observed in the first hour; non-hygroscopic material (traced with m/z 57) is injected with DL POA and reduces the apparent κ to 0.08, less than the κ of DL POA but slightly greater than the measured value of 0.06. The minimum predicted and measured hygroscopicity differ; the AMS signal does not account for the entire non-volatile and non-hygroscopic material at the smaller dry diameters in the range of CCN activity (35 – 70nm, Compare Figure S1a to S2a). The CCN predicted values become more hygroscopic after PDT (1 h after DL POA injection, ). The predicted and measured hygroscopicity also increases in the MOF POA plus α-pinene SOA mixture; suggesting that even MOF POA and SOA continue to experience some chemical oxidation as the relative contribution of m/z 57 to m/z 44 decreases over time. The empirical equation tends to overpredict the MOF POA plus α-pinene SOA system.
Figure 4. Modeled UMR κ-hygroscopicity compared with CCN measured κ-hygroscopicity. (a) α-pinene SOA + DL POA. (b) α-pinene SOA + MOF POA.
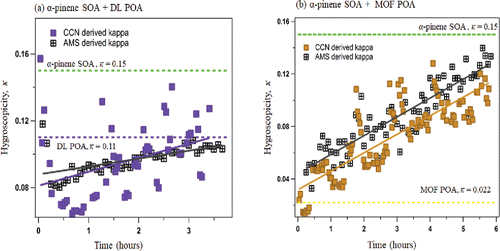
In , the simple UMR model (Equation [Equation2[2]
[2] ]) predicts hygroscopicity to within ∼50% uncertainty (). The UMR model captures evolving chemical changes that influence monotonic and non-monotonic hygroscopicity in both the MOF POA and DL POA plus α-pinene SOA mixtures. There is a strong positive correlation between the measured CCN hygroscopicity and the UMR model parameter (
, ). We also explore correlations with previously suggested AMS-CCN empirical relationships. The elemental oxygen to carbon (O:C) ratio indicates the degree of oxidation of oganic aerosol (Aiken et al., Citation2008). The hygroscopicity of organic aerosol has been modeled using a previously developed empirical relationships between the aerosol oxidation (O:C) of ambient organic aerosol and the supersaturated hygroscopicity (Jimenez, Canagaratna et al. Citation2009, Chang et al. Citation2010, Frosch et al. Citation2011, Tritscher et al. Citation2011). The fraction of m/z 44 in the total organic aerosol signal (f44) is used to calcluate the O:C ratio (Eqn. S1) (Chang et al. Citation2010). The estimated O:C value is less than 0.1 and is insensitive to changes in the measured CCN hygroscopicity (). With low aerosol oxidation (O:C < 0.3), the Chan et al. (2010) empirical CCN relationship is unable to robustly model the hygrosocopicity. Thus by including the m/z 57 fragment in the UMR based relationship, the degrees of freedom in the parameterization of oxidation-based organic aerosol hygroscopicity are increased and the
ratio provides a robust mechanism for estimating the supersaturated hygroscopicity of organic aerosol mixed with significantly less oxidized components.
Figure 5. (a) CCN measured κ-hygroscopicity compared with the UMR modeled κ-hygroscopicity. (b) Correlation between the measured CCN hygroscopicity with m/z 57 and m/z 44 ratios (closed markers) and the correlation between the oxidized fraction, the estimated O:C ratio and the CCN measured κ-hygroscopicity (open markers).
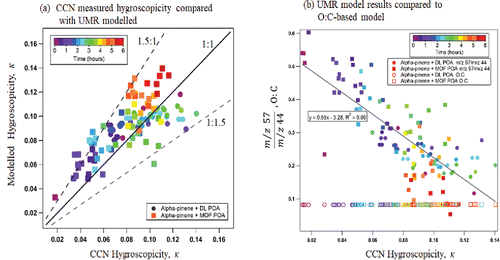
4. Implications
Asa-Awuku et al. (Citation2009) were able to conclusively show that organics from anthropogenic and biogenic origins can exist in multiple mixing states. The propensity of these mixtures to form droplets in the supersaturated regime is significantly modified with chemical aging. Here we observed that the hygroscopicity of motor oil POA (κ = 0.022) and diesel exhaust POA (κ = 0.11) are remarkably different from each other even though their chemical fingerprints (Fig. 3 in Asa-Awuku et al. Citation2009) are similar. Thus, in this work, we also observe that the previous elemental composition information from the higher resolution AMS mass spectrum (unavailable here) did not provide sufficient information to describe the molecular force interactions that govern specific aerosol properties (i.e., volatility, miscibility or separation and now hygroscopicity). The propensity for aerosol mixing and miscibility may be similar to hygroscopicity and the ability to form droplets; molecular level interactions (such as those that govern an affinity for water) drive organic mixing (without collisions). The apparent hygroscopicities of diesel exhaust and α-pinene SOA are within the same order of magnitude and the two components readily mixed to form an internally well-mixed aerosol. The apparent hygroscopicities of MOF POA and α-pinene SOA are an order of magnitude different and do not readily mix. Hence, the comparison of the CCN activity in κ-hygroscopicity space may be a good indicator of the ability of the complex organic aerosol from distinct sources to form internal mixtures.
The process of aerosol mixing is time-dependent and we observe the effects of evaporation, condensation, coagulation, and oxidation for complex CCN. The measured and predicted κ-hygroscopicity of the MOF POA + α-pinene SOA and DL POA + α-pinene SOA mixtures are transient; once collision processes dominate (by coagulation and wall-loss) particles become more CCN active. The CCN activity after injection is non-monotonic for DL POA + α-pinene SOA but monotonic for MOF POA + α-pinene SOA. To our knowledge, this is the first time non-monotonic CCN activity of an aerosol system has been published in the literature.
From threshold droplet growth analysis (TDGA), unmixed DL POA and MOF POA grow to similar average droplet sizes. This is consistent with the similarity in “chemical fingerprints” as described by Asa-Awuku et al. (Citation2009). The modifications in hygroscopicity observed upon mixing the POA with the α-pinene SOA does not translate into a significant difference in droplet growth. In the α-pinene SOA + DL POA, the observed suppression in the droplet sizes upon injecting the POA may be due to the formation of mixtures with lower mass accommodation coefficients. This may slow down the water uptake and hence result in smaller final droplet sizes (Ruehl et al. Citation2008). The co-condensation of the initially volatile DL POA may have influenced the slight increase in the droplet diameters after entropic mixing equilibrium was attained (Topping et al. Citation2013). The differences in the droplet diameters observed for both systems are all within two bin sizes hence the changes may not be significant considering optical particle counter sizing sensitivity (Moore, Nenes et al. Citation2010). Future improvements in the CCNc optical particle counter sensitivity are therefore needed to enable a robust exploration of droplet kinetics.
The proposed UMR model method is different than other AMS-CCN techniques. The UMR method applies the ratio of fragments; an absolute amount (typically requiring density and wall-loss correction) is not required. It should also be noted that simultaneous CCN and High-Resolution AMS data was not available for this study. Although, a direct relationship exists between m/z 44 ion and O:C (Chang, Slowik et al. Citation2010, Ng et al. Citation2010), this UMR-CCN model also accounts for the contribution of non-hygroscopic material (as traced with m/z 57). The inclusion of the non-hygroscopic aerosol fractions (m/z 57) may improve the prediction of hygroscopicity from AMS data in less oxidized, complex aerosol systems. However, all AMS coupled CCN predictions have limitations. The AMS bulk information is applied to the entire distribution and does not account for size-resolved mixing state. In addition, AMS-CCN models work best after PDT and as particles move to larger sizes above the lower detection range of the AMS aerodynamic lens. Furthermore Equation Equation2[2]
[2] may be most applicable to mixtures that can be resolved with a binary system of hygroscopic and non-hygroscopic components.
Nonetheless, the ability to predict CCN activity from UMR AMS fragment ions and the nature of mixing from the CCN hygroscopicity may be a promising tool to gain further insight into the evolution of organic aerosol mixtures and their interaction with water vapor. When available, CCN information coupled with fragment ion data will provide both hygroscopicity and mixing insights that may lead to the robust modeling of CCN activity and droplet growth kinetics in transient aerosol systems.
UAST_1392480_Supplemental_data
Download PDF (742.5 KB)Additional information
Funding
References
- Aiken, A. C., Decarlo, P. F., Kroll, J. H., Worsnop, D. R., Huffman, J. A., Docherty, K. S., Ulbrich, I. M., Mohr, C., Kimmel, J. R., and Sueper, D. (2008). O/C and OM/OC ratios of primary, secondary, and ambient organic aerosols with high-resolution time-of-flight aerosol mass spectrometry. Environ. Sci. Technol., 42(12):4478–4485.
- Alfarra, M. R., Coe, H., Allan, J. D., Bower, K. N., Boudries, H., Canagaratna, M. R., Jimenez, J. L., Jayne, J. T., Garforth, A. A., Li, S. M., and Worsnop, D. R. (2004). Characterization of Urban and Rural Organic Particulate in the Lower Fraser Valley Using Two Aerodyne Aerosol Mass Spectrometers. Atmos. Environ., 38(34):5745–5758.
- Asa-Awuku, A., Miracolo, M. A., Kroll, J. H., Robinson, A. L., and Donahue, N. M. (2009). Mixing and Phase Partitioning of Primary and Secondary Organic Aerosols. Geophys. Res. Lett., 36(15):L15827. doi:10.1029/2009GL039301.
- Asa-Awuku, A., Moore, R. H., Nenes, A., Bahreini, R., Holloway, J. S., Brock, C. A., Middlebrook, A. M., Ryerson, T. B., Jimenez, J. L., DeCarlo, P. F., Hecobian, A., Weber, R. J., Stickel, R., Tanner, D. J., and Huey, L. G. (2011). Airborne cloud condensation nuclei measurements during the 2006 Texas Air Quality Study. J. Geophys. Res.-Atmos., 116(D11).
- Asa-Awuku, A., Sullivan, A. P., Hennigan, C. J., Weber, R. J., and Nenes, A. (2008). Investigation of Molar Volume and Surfactant Characteristics of Water-Soluble Organic Compounds in Biomass Burning Aerosol. Atmos. Chem. Phys., 8(4):799–812.
- Canagaratna, M. R., Onasch, T. B., Wood, E. C., Herndon, S. C., Jayne, J. T., Cross, E. S., Miake-Lye, R. C., Kolb, C. E., and Worsnop, D. R. (2010). Evolution of Vehicle Exhaust Particles in the Atmosphere. J. Air Waste Manag. Assoc., 60(10):1192–1203.
- Chang, R.-W., Slowik, J., Shantz, N., Vlasenko, A., Liggio, J., Sjostedt, S., Leaitch, W., and Abbatt, J. (2010). The Hygroscopicity Parameter (κ) of Ambient Organic Aerosol at a Field Site Subject to Biogenic and Anthropogenic Influences: Relationship to Degree of Aerosol Oxidation. Atmos. Chem. Phys., 10(11):5047–5064.
- Chang, R. Y. W., Slowik, J. G., Shantz, N. C., Vlasenko, A., Liggio, J., Sjostedt, S. J., Leaitch, W. R., and Abbatt, J. P. D. (2010). The Hygroscopicity Parameter (Kappa) of Ambient Organic Aerosol at a Field Site Subject to Biogenic and Anthropogenic Influences: Relationship to Degree of Aerosol Oxidation. Atmos. Chem. Phys., 10(11):5047–5064.
- Cubison, M., Ervens, B., Feingold, G., Docherty, K., Ulbrich, I., Shields, L., Prather, K., Hering, S., and Jimenez, J. (2008). The Influence of Chemical Composition and Mixing State of Los Angeles Urban Aerosol on CCN Number and Cloud Properties. Atmos. Chem. Phys., 8(18):5649–5667.
- Duplissy, J., DeCarlo, P. F., Dommen, J., Alfarra, M. R., Metzger, A., Barmpadimos, I., Prevot, A. S. H., Weingartner, E., Tritscher, T., Gysel, M., Aiken, A. C., Jimenez, J. L., Canagaratna, M. R., Worsnop, D. R., Collins, D. R., Tomlinson, J., and Baltensperger, U. (2011). Relating Hygroscopicity and Composition of Organic Aerosol Particulate Matter. Atmos. Chem. Phys., 11(3):1155–1165.
- Engelhart, G., Asa-Awuku, A., Nenes, A., and Pandis, S. (2008). CCN Activity and Droplet Growth Kinetics of Fresh and Aged Monoterpene Secondary Organic Aerosol. Atmos. Chem. Phys., 8(14):3937–3949.
- Frosch, M., Bilde, M., DeCarlo, P., Juranyi, Z., Tritscher, T., Dommen, J., Donahue, N., Gysel, M., Weingartner, E., and Baltensperger, U. (2011). Relating Cloud Condensation Nuclei Activity and Oxidation Level of α‐Pinene Secondary Organic Aerosols. J. Geophys. Res.: Atmos., 116:D22212. doi:10.1029/2011JD016401
- Fushimi, A., Saitoh, K., Fujitani, Y., Hasegawa, S., Takahashi, K., Tanabe, K., and Kobayashi, S. (2011). Organic-Rich Nanoparticles (Diameter: 10–30nm) in Diesel Exhaust: Fuel and Oil Contribution Based on Chemical Composition. Atmos. Environ., 45(35):6326–6336.
- Gentner, D. R., Isaacman, G., Worton, D. R., Chan, A. W., Dallmann, T. R., Davis, L., Liu, S., Day, D. A., Russell, L. M., and Wilson, K. R. (2012). Elucidating Secondary Organic Aerosol from Diesel and Gasoline Vehicles Through Detailed Characterization of Organic Carbon Emissions. Proc. Nat. Acad. Sci., 109(45):18318–18323.
- Jacobson, M. Z. (2001). Strong Radiative Heating Due to the Mixing State of Black Carbon in Atmospheric Aerosols. Nature, 409(6821):695–697.
- Jathar, S. H., Miracolo, M. A., Tkacik, D. S., Donahue, N. M., Adams, P. J., and Robinson, A. L. (2013). Secondary Organic Aerosol Formation from Photo-Oxidation of Unburned Fuel: Experimental Results and Implications for Aerosol Formation from Combustion Emissions. Environ. Sci. Technol., 47(22):12886–12893.
- Jimenez, J., Canagaratna, M., Donahue, N., Prevot, A., Zhang, Q., Kroll, J. H., DeCarlo, P. F., Allan, J. D., Coe, H., and Ng, N. (2009). Evolution of Organic Aerosols in the Atmosphere. Science, 326(5959):1525–1529.
- Khalizov, A. F., Lin, Y., Qiu, C., Guo, S., Collins, D., and Zhang, R. (2013). Role of OH-Initiated Oxidation of Isoprene in Aging of Combustion Soot. Environ. Sci. Technol., 47(5):2254–2263.
- King, S. M., Rosenoern, T., Shilling, J. E., Chen, Q., and Martin, S. T. (2007). Cloud Condensation Nucleus Activity of Secondary Organic Aerosol Particles Mixed with Sulfate. Geophys. Res. Lett., 34:L24806. doi:10.1029/2007GL030390.
- Lamarque, J. F., Bond, T. C., Eyring, V., Granier, C., Heil, A., Klimont, Z., Lee, D., Liousse, C., Mieville, A., and Owen, B. (2010). Historical (1850–2000) Gridded Anthropogenic and Biomass Burning Emissions of Reactive Gases and Aerosols: Methodology and Application. Atmos. Chem. Phys., 10(15):7017–7039.
- Lambe, A., Onasch, T., Massoli, P., Croasdale, D., Wright, J., Ahern, A., Williams, L., Worsnop, D., Brune, W., and Davidovits, P. (2011). Laboratory Studies of the Chemical Composition and Cloud Condensation Nuclei (CCN) Activity of Secondary Organic Aerosol (SOA) and Oxidized Primary Organic Aerosol (OPOA). Atmos. Chem. Phys, 11(17):8913–8928.
- Lathem, T. L., and Nenes, A. (2011). Water Vapor Depletion in the DMT Continuous-Flow CCN Chamber: Effects on Supersaturation and Droplet Growth. Aerosol Sci. Technol., 45(5):604–615.
- Lu, J. W., Rickards, A. M., Walker, J. S., Knox, K. J., Miles, R. E., Reid, J. P., and Signorell, R. (2014). Timescales of Water Transport in Viscous Aerosol: Measurements on Sub-Micron Particles and Dependence on Conditioning History. Phys. Chem. Chem. Phys, 16(21):9819–9830.
- Massoli, P., Lambe, A., Ahern, A., Williams, L., Ehn, M., Mikkilä, J., Canagaratna, M., Brune, W., Onasch, T., and Jayne, J. (2010). Relationship Between Aerosol Oxidation Level and Hygroscopic Properties of Laboratory Generated Secondary Organic Aerosol (SOA) Particles. Geophys. Res. Lett., 37:L24801. doi:10.1029/2010GL045258.
- McFiggans, G., Alfarra, M. R., Allan, J., Bower, K., Coe, H., Cubison, M., Topping, D., Williams, P., Decesari, S., and Facchini, C. (2005). Simplification of the Representation of the Organic Component of Atmospheric Particulates. Faraday Discuss., 130:341–362.
- Moffet, R. C., and Prather, K. A. (2009). In-Situ Measurements of the Mixing State and Optical Properties of Soot with Implications for Radiative Forcing Estimates. Proc. Nat. Acad. Sci., 106(29):11872–11877.
- Mohr, C., Huffman, J. A., Cubison, M. J., Aiken, A. C., Docherty, K. S., Kimmel, J. R., Ulbrich, I. M., Hannigan, M., and Jimenez, J. L. (2009). Characterization of Primary Organic Aerosol Emissions from Meat Cooking, Trash Burning, and Motor Vehicles with High-Resolution Aerosol Mass Spectrometry and Comparison with Ambient and Chamber Observations. Environ. Sci. Technol., 43(7):2443–2449.
- Moore, R. H., Ingall, E. D., Sorooshian, A., and Nenes, A. (2008). Molar Mass, Surface Tension, and Droplet Growth Kinetics of Marine Organics from Measurements of CCN Activity. Geophys. Res. Lett, 35:L07801. doi:10.1029/2008GL033350.
- Moore, R. H., Nenes, A., and Medina, J. (2010). Scanning Mobility CCN Analysis—A Method for Fast Measurements of Size-Resolved CCN Distributions and Activation Kinetics. Aerosol Sci. Technol., 44(10):861–871.
- Nenes, A., and Seinfeld, J. H. (2003). Parameterization of Cloud Droplet Formation in Global Climate Models. J. Geophys. Res., 108(D14):4415. doi:10.1029/2002JD002911.
- Ng, N., Canagaratna, M., Zhang, Q., Jimenez, J., Tian, J., Ulbrich, I., Kroll, J., Docherty, K., Chhabra, P., and Bahreini, R. (2010). Organic Aerosol Components Observed in Northern Hemispheric Datasets from Aerosol Mass Spectrometry. Atmos. Chem. Phys., 10(10):4625–4641.
- Petters, M., and Kreidenweis, S. (2008). A Single Parameter Representation of Hygroscopic Growth and Cloud Condensation Nucleus Activity–Part 2: Including Solubility. Atmos. Chem. Phys., 8(20):6273–6279.
- Petters, M. D., and Kreidenweis, S. M. (2007). A Single Parameter Representation of Hygroscopic Growth and Cloud Condensation Nucleus Activity. Atmos. Chem. Phys., 7(8):1961–1971.
- Presto, A. A., Huff Hartz, K. E., and Donahue, N. M. (2005). Secondary Organic Aerosol Production from Terpene Ozonolysis. 1. Effect of UV Radiation. Environ. Sci. Technol., 39(18):7036–7045.
- Raatikainen, T., Moore, R., Lathem, T., and Nenes, A. (2012). A Coupled Observation–Modeling Approach for Studying Activation Kinetics from Measurements of CCN Activity. Atmos. Chem. Phys., 12(9):4227–4243.
- Roberts, G., and Nenes, A. (2005). A Continuous-Flow Streamwise Thermal-Gradient CCN Chamber for Atmospheric Measurements. Aerosol Sci. Technol., 39(3):206–221.
- Robinson, E. S., Donahue, N. M., Ahern, A. T., Ye, Q., and Lipsky, E. (2016). Single-Particle Measurements of Phase Partitioning Between Primary and Secondary Organic Aerosols. Faraday Discuss., 189(0):31–49.
- Rose, D., Gunthe, S. S., Mikhailov, E., Frank, G. P., Dusek, U., Andreae, M. O., and Pöschl, U. (2008). Calibration and Measurement Uncertainties of a Continuous-Flow Cloud Condensation Nuclei Counter (DMT-CCNC): CCN Activation of Ammonium Sulfate and Sodium Chloride Aerosol Particles in Theory and Experiment. Atmos. Chem. Phys., 8(5):1153–1179.
- Ruehl, C., Chuang, P., and Nenes, A. (2008). How Quickly do Cloud Droplets form on Atmospheric Particles? Atmos. Chem. Phys., 8(4):1043–1055.
- Ruehl, C. R., Chuang, P. Y., Nenes, A., Cappa, C. D., Kolesar, K. R., and Goldstein, A. H. (2012). Strong Evidence of Surface Tension Reduction in Microscopic Aqueous Droplets. Geophys. Res. Lett., 39:L23801. doi:10.1029/2012GL053706.
- Sakurai, H., Tobias, H. J., Park, K., Zarling, D., Docherty, K. S., Kittelson, D. B., McMurry, P. H., and Ziemann, P. J. (2003). On-Line Measurements of Diesel Nanoparticle Composition and Volatility. Atmos. Environ., 37(9):1199–1210.
- Saukko, E., Lambe, A., Massoli, P., Koop, T., Wright, J., Croasdale, D., Pedernera, D., Onasch, T., Laaksonen, A., and Davidovits, P. (2012). Humidity-Dependent Phase State of SOA Particles from Biogenic and Anthropogenic Precursors. Atmos. Chem. Phys., 12(16):7517–7529.
- Schnaiter, M., Linke, C., Möhler, O., Naumann, K. H., Saathoff, H., Wagner, R., Schurath, U., and Wehner, B. (2005). Absorption Amplification of Black Carbon Internally Mixed with Secondary Organic Aerosol. J. Geophys. Res., 110:D19204. doi:10.1029/2005JD006046.
- Song, C., Zaveri, R. A., Alexander, M. L., Thornton, J. A., Madronich, S., Ortega, J. V., Zelenyuk, A., Yu, X. Y., Laskin, A., and Maughan, D. A. (2007). Effect of Hydrophobic Primary Organic Aerosols on Secondary Organic Aerosol Formation from Ozonolysis of α‐Pinene. Geophys. Res. Lett., 34:L20803. doi:10.1029/2007GL030720.
- Topping, D., Connolly, P., and McFiggans, G. (2013). Cloud Droplet Number Enhanced by Co-Condensation of Organic Vapours. Nat. Geosci., 6:443–446.
- Tritscher, T., Jurányi, Z., Martin, M., Chirico, R., Gysel, M., Heringa, M. F., DeCarlo, P. F., Sierau, B., Prévôt, A. S., and Weingartner, E. (2011). Changes of Hygroscopicity and Morphology During Ageing of Diesel Soot. Environ. Res. Lett., 6(3):034026.
- Vaden, T. D., Song, C., Zaveri, R. A., Imre, D., and Zelenyuk, A. (2010). Morphology of Mixed Primary and Secondary Organic Particles and the Adsorption of Spectator Organic Gases During Aerosol Formation. Proc. Nat. Acad. Sci., 107(15):6658–6663.
- Vu, D., Short, D., Karavalakis, G., Durbin, T., and Asa-Awuku, A. (2015). Integrating Cloud Condensation Nuclei Predictions with Fast Time Resolved Aerosol Instrumentation to Determine the Hygroscopic Properties of Emissions Over Transient Drive Cycles. Aerosol Sci. Technol., 49(11):1149–1159.
- Vu, D., Short, D. Z., Karavalakis, G., Durbin, T. D., and Asa-Awuku, A. (2017). Will Aerosol Hygroscopicity Change with Biodiesel, Renewable Diesel Fuels and Emission Control Technologies? Environ. Sci. Technol 51(3):1580–1586. doi: 10.1021/acs.est.6b03908.
- Wang, J., Cubison, M., Aiken, A., Jimenez, J., and Collins, D. (2010). The Importance of Aerosol Mixing State and Size-Resolved Composition on CCN Concentration and the Variation of the Importance with Atmospheric Aging of Aerosols. Atmos. Chem. Phys., 10(15):7267–7283.
- Weitkamp, E. A., Lambe, A. T., Donahue, N. M., and Robinson, A. L. (2008). Laboratory Measurements of the Heterogeneous Oxidation of Condensed-Phase Organic Molecular Makers for Motor Vehicle Exhaust. Environ. Sci. Technol., 42(21):7950–7956.
- Zaveri, R. A., Barnard, J. C., Easter, R. C., Riemer, N., and West, M. (2010). Particle‐Resolved Simulation of Aerosol Size, Composition, Mixing State, and the Associated Optical and Cloud Condensation Nuclei Activation Properties in an Evolving Urban Plume. J. Geophys. Res.: Atmos., 115(D17).
- Zhang, K. M., and Wexler, A. S. (2004). Evolution of Particle Number Distribution Near Roadways—Part I: Analysis of Aerosol Dynamics and Its Implications for Engine Emission Measurement. Atmos. Environ., 38(38):6643–6653.
- Zhang, Q., Alfarra, M. R., Worsnop, D. R., Allan, J. D., Coe, H., Canagaratna, M. R., and Jimenez, J. L. (2005). Deconvolution and Quantification of Hydrocarbon-Like and Oxygenated Organic Aerosols Based on Aerosol Mass Spectrometry. Environ. Sci. Technol., 39(13):4938–4952.