Abstract
High outputs of respirable solid-phase aerosols were generated from viscous solutions or suspensions of low- and high-molecular weight polyvinylpyrrolidone (PVP) solutions, 10% (w/v) albumin and, gamma globulin solutions as well as 10.3% (w/v) surfactant suspensions. A central fluid flow was aerosolized by coaxial converging compressed air. The water was evaporated from the droplets using warm dilution air and infrared radiation. The resulting aerosol particles were concentrated using a virtual impactor. The aerosols were generated at fluid flow rates between 1 and 3 ml/min and delivered at a flow rate of 44 l/min as 2.6–3.6 μm MMAD aerosols with geometric standard deviations between 1.5 and 2. Increases in viscosity over the range of 4–39 cSt caused a modest increase in MMAD. Increases in aerosol exit orifice diameter were associated with a decrease in aerosol diameter. Increases in compressed air pressure caused a decrease in aerosol diameter. Increases in fluid flow rate resulted in modest increases in MMAD together with proportional increases in output mass. Aerosolizing 10% 8 kDa PVP at 3 ml/min resulted in the delivery of 193 mg/min of PVP at 64% efficiency enabling 1.2 g to be collected in 7 min. Aerosolizing 10.3% surfactant suspensions at 3 ml/min resulted in the delivery of up to 163 mg/min with 59% efficiency. The surface tension of the surfactant was not changed by these processes. SEM showed dimpled particles of PVP, albumin, and gamma globulin indicating that their aerodynamic diameter was less than their morphometric diameter.
Copyright © 2018 American Association for Aerosol Research
Editor:
Introduction
The treatment of respiratory diseases and syndromes by aerosol inhalation has been achieved through the generation and delivery of liquid, dry powder, or propellant-driven aerosols that contain the active pharmaceutical ingredient. Although the positive impact on the control of respiratory disease by these drugs and devices is unquestioned, new technologies are required to meet unmet clinical needs and future challenges. These include the delivery of active pharmaceutical ingredients comprised of proteins, antibodies, surfactant, anti-infectives, genetic constructs, or secretomes. In many cases, therapeutically relevant doses of 20 mg to over 1 g are required to be deposited in the lungs as noted below. These agents can form solutions or suspensions whose viscosity either reduces or prevents aerosol outputs from jet atomizers as well as vibrating mesh and ultrasonic nebulizers (McCallion et al. Citation1995; Weber et al. Citation1997; Chan et al. Citation2012). A pure solid-phase aerosol contains some 25 times the mass of an active agent than a similar-sized aqueous aerosol generated from dilute solutions such as <4% alpha-1 antitrypsin (Brand et al. Citation2009). Dry powder inhalers (Young et al. Citation2004; Pohlmann et al. Citation2013; Weers and Tarara Citation2014), while potentially providing a higher dose of the active pharmaceutical ingredient per puff, often require the addition of excipients and the need for an excessive number of capsules. To address the limitations of present devices, an aqueous liquid to the solid-phase aerosol delivery system was developed and evaluated. This aerosol generation and delivery system, SUPRAER®-CA, aerosolizes relatively viscous solutions or suspensions of proteins and surfactant and delivers them as pure fine particle solid-phase aerosols.
The inability to rapidly deliver aerosols of clinically relevant doses of such active pharmaceutical ingredients limits the therapeutic options available to patients with lung syndromes and diseases. Whether these therapies are administered in the clinic or at home, short treatment times will improve the acceptability and adherence to the treatment regime. Within clinical and domiciliary settings, there is an ever-increasing number of respiratory conditions and therapeutic agents that could benefit from rapid delivery of fine particle therapeutic aerosols and/or an increase in the dose deposited.
Adult patients with acute respiratory distress syndrome have both depleted and impaired surfactant (Dushianthan et al. Citation2014) that contribute to their 40% mortality rate. In rabbit models, depletion of surfactant by repeated lavage or by acid challenge, the instillation of 100 or 200 mg/kg of surfactant at a concentration of 35 mg/ml into the tracheobronchial tree initiates a dramatic and early temporal improvement in arterial oxygen (PaO2) (Walther et al. Citation2014; Ricci et al. Citation2017). Following 2–7 mg/kg of aerosol delivery of surfactant to pre-term lambs indicated some beneficial responses (Lewis et al. Citation1991; Gordon et al. Citation2003; Rahmel et al. Citation2012). These findings suggest that aerosol delivery may only require 1/10 of that administered by instillation. The total surfactant pool in the lungs has been estimated to be 5–10 mg/kg. Given the inflammation-induced degradation of surfactant in patients with ARDS, these data indicate a target dose of 7–20 mg/kg should be delivered to the lungs. Thus, as much as 1.4 g may be required to be delivered in a 70 kg patient with ARDS to elicit clinically meaningful physiologic responses. The inability of sufficient aerosolized surfactant to be deposited in the lungs is one of the reasons given for the failure of this treatment (Anzueto et al. Citation1996; Yeates Citation2018). In addition, the delivery of sufficient surfactant by aerosol to achieve a clinically relevant response to treat respiratory distress syndrome in premature babies is still a subject at large (Yeates Citation2018).
Aerosol delivery of alpha-1 antitrypsin (50–160 mg) has been under development for the treatment of hereditary emphysema (Griese and Scheuch Citation2016). Aerosol therapy of monoclonal antibodies has been evaluated for their potential to treat lung diseases (Guilleminault et al. Citation2014). The treatment of lung cancer has been proposed using aerosols of therapeutic antibodies (Maillet et al. Citation2011) and paclitaxel (Joshi et al. Citation2014). Aerosol inhalation provides a delivery route for anti-infectives that requires a substantial dose to be delivered to the lungs (Maselli et al. Citation2017) and/or have considerable systemic toxicity when administered intravenously. Colistin (40–80 mg) (Gurjar Citation2015) and Amikacin (400 mg) (Liu et al. Citation2017) and its liposomal formulation, Amikacin (560 mg) (Clancy et al. Citation2013) are potential candidates for improved aerosol delivery to treat respiratory infections. Mucolytics such as N-acetylcysteine (350 mg) (Tomioka et al. Citation2005) and mannitol (400 mg) (Zhu et al. Citation2015) have been used to treat patients with cystic fibrosis. Pulmozyme (2.5 mg), a mucolytic (Frederiksen et al. Citation2006) is the only biologic approved for aerosol inhalation therapy in patients with cystic fibrosis. Aerosol systems with lower shear are attractive for the delivery of drug containing liposomes (10–15 mg) (Lehofer et al. Citation2014) as well as gene therapy (Rijnders et al. Citation2008) and anticancer agents (Rudokas et al. Citation2016). Higher doses are warranted when delivering anti-tuberculosis agents (Chan Citation2014; Das et al. Citation2015). Larger molecules including plasmids (Davies Citation2014) and those contained in secretomes present future challenges.
There are biotherapeutic drugs and/or drug candidates for the treatment of lung cancer, pulmonary fibrosis, pneumonia and asthma, emphysema and obstructive lung diseases in development that are destined to be delivered intravenously rather than by inhalation. By comparison, the inhalational route substantially decreases the total dose delivered as well as the systemic side effects. One of the factors limiting the adoption of aerosol inhalation therapy is likely due to the perception that the aerosol delivery technologies have not been developed to deliver these candidates.
The development of technology to generate aerosols from viscous solutions or suspensions and to deliver them as fine particle solid-phase aerosols has been summarized in a series of abstracts (Yeates 2012; Yeates 2013a, 2013b; Yeates and Heng Citation2016a; Citation2016b; Yeates and Heng 2017). Herein, a comprehensive functional description of this aerosol delivery system, SUPRAER-CA, is elucidated and the versatility of its performance demonstrated.
SUPRAER-CA aerosol generation and delivery system
SUPRAER-CA, is a laboratory device designed and constructed for use in bench and animal studies. SUPRAER-CA uses compressed air (CA) to aerosolize an aqueous solution or suspension containing the active agent. The water from the generated droplets is evaporated to form a solid-phase aerosol that is subsequently concentrated and delivered at the output as a respirable solid-phase aerosol. The ability to control the parameters governing aerosol generation, processing, and delivery is necessary for the evaluation and determination of the optimum design specifications related to the use of this technology for clinical and domiciliary treatment of respiratory diseases and syndromes as well as respiratory administration of systemically active drugs.
SUPRAER-CA is comprised of an aerosol processing system atop a console. A photograph of SUPRAER-CA shows the major functional and operational components (). A 3D schematic view of the aerosol processing system is shown in . The major components of the aerosol processing system are (i) a nozzle holder and a nozzle (), (ii) a flow distributor () which contains a counter-flow tube, (iii) a 23 cm long cylindrical quartz evaporation chamber (), (iv) a radially aligned multi-slit aerosol concentrator (, and ), and (v) an aerosol exit cone () with a 21.8 mm inner diameter output port. The functional controls on the console () include, (i) a regulator to control the pressure of compressed air up to 60 psi, (ii) a regulator to control the flow of the dilution air up to 200 l/min, (iii) a proportional–integral–derivative controller (PID) to set the temperature of the compressed air supplied to the nozzle and counter-flow tube, (iv) a PID to set the temperature of the dilution air supplied to the aerosol generation and processing system and (v) a knob to control the intensity of infrared radiation. In addition, each of the heating elements can be switched on and off as desired.
Figure 1. (a) A photograph of SUPRAER-CA with an aerosol generation and processing system atop the console. (b) A 3-d representation of the aerosol processing system with a nozzle in a position to be inserted into the flow distributor. (c) Photograph of a nozzle holder assembly. (d) A photograph of the flow distributor. (e1) Photograph of the radial slit concentrator showing the sculptured acceleration nozzles. (e2) a photograph taken through an exhaust port showing the sculptured backside of the acceleration and deceleration nozzles and the gap between these nozzles.

SUPRAER-CA flow diagram
A flow diagram of the control systems for SUPRAER-CA is shown in . The compressed air supply is split such that the pressure of the air going to the nozzle and the counter-flow tube is regulated independently from that of the low-pressure dilution air. Each gas flow is measured with a mass flow meter and warmed by an infrared bulb in a ceramic tube. The temperatures of each of these gases are measured within the console using a thermocouple centered in each gas stream. This facilitates the independent control of both the compressed gas and the dilution gas temperatures using the PID controllers. The warm high-pressure gas is split so as to deliver compressed gas to the aerosolizing nozzle and to the counter-flow tube. This counter-flow tube provides a jet of gas of opposite direction to the aerosol plume generated by the nozzle such that the aerosol plume is arrested midway between the nozzle and the end of the counter-flow tube. An orifice upstream from the counter-flow tube restricts the counter-flow gas such that changes in nozzle pressure (velocity) are roughly matched with changes in counter-flow gas velocity. The fluid to be aerosolized is pumped to the nozzle at the same pressure as the compressed gas within the nozzle. The warm dilution gas enters a flow distributor where its velocity and direction are changed using low-resistance baffles such that the gas flow is spatially uniform as it passes into the evaporation chamber. As this gas mixes with the arrested aerosol plume, it augments the evaporation of the water from the aerosol and transports the aerosol through the evaporation chamber to the aerosol concentrator. A rapidly responding infrared bulb within the console located immediately adjacent to the evaporation chamber in the region of augmented aerosol mixing provides much of the latent heat required to evaporate the water from the aerosol. The resulting aerosol particles, now reduced in diameter, flow with a relatively uniform velocity within the evaporation chamber towards the acceleration nozzles of the aerosol concentrator described below. The concentrated aerosol particles suspended in a minor fraction of the total gas flow are collected by the output cone. SUPRAER-CA has substantial safety features that are not included in this schematic.
Generation of the liquid aerosol
The nozzles () and flow distributor () are designed such that the nozzles are easily interchangeable. The nozzle holder has a Luer fitting for connection to the fluid pump (). The long barrel ensures coaxial alignment with the counter-flow tube. It is terminated with a nozzle with a specified aerosol exit diameter (). The nozzles are designed such that the fluid flows through a centrally aligned fluid supply channel to a 230 µm diameter channel into an aerosolizing space. The compressed gas enters the nozzle holder through a port that aligns with an annulus in the flow distributor. This gas flows through two channels to the nozzle. The nozzle is internally configured such that the gas flows uniformly between a conical fluid nozzle and a cone-like outer component to an aerosol exit orifice. The nozzle components are designed to resist wetting and fluid backflow.
During operation, a nozzle of selected aerosol exit orifice diameter () is placed in the barrel of the flow distributor (). This flow distributor delivers warm dry compressed air to the nozzle and to the counter-flow tube as well as warm dry dilution air into the quartz evaporation chamber. When the nozzle holder is fully inserted, ports for the compressed air () on the side of the nozzle holder align with the annulus within the barrel of the flow distributor. The compressed air flows through channels in the nozzle holder to the nozzle. The fluid to be aerosolized is contained within a glass syringe with a Teflon plunger (10 ml, ILS, Germany). It is pumped at a selected flow rate (1–3 ml/min) by a syringe pump (Legato 210, KD Scientific, MA) via a small bore (1 mm ID) flexible tube to the fluid input port of the nozzle holder. The interaction of the axial fluid jet with the surrounding compressed air creates an aqueous columnar-like aerosol plume sheathed in air devoid of particles.
Evaporation of the water from the aerosol droplets
The water contained in the aerosol droplets is evaporated by the infrared radiation and warm dry air from the nozzle, flow distributor and counter-flow tube resulting in a solid-phase aerosol of smaller diameter (2–4 µm) comprising the non-volatile solute/suspension. The energy to provide the latent heat of evaporation of the aqueous aerosol is largely provided by a 250-Watt infrared bulb within the console. The infrared bulb is designed to maximize infrared radiation in the absorption band for water <3500 nm. The cylindrical evaporation chamber is made of quartz to facilitate transmission of this infrared radiation into the chamber. An aluminum reflector on the opposite side of the evaporation chamber reflects the infrared radiation transmitted through the evaporation chamber back to the chamber. The generated solid-phase aerosol enters the radially positioned acceleration nozzles (slits) of the aerosol concentrator ( and ).
Concentration of the aerosol
The radial slit concentrator ( and ) is an extremely low resistance virtual impactor. This low resistance to gas flow was achieved using multiple radially positioned slit nozzles having a total length of 19 cm, together with the roughly parabolic contouring of both the acceleration and deceleration nozzles (). The minor aerosol flow (output flow) containing most of the aerosol particles enters the deceleration nozzles. The major flow within the concentrator is at right angles to the flow within the acceleration and deceleration nozzles. This major flow exits through the gap between these nozzles (). The acceleration nozzle slits have a width of 1.1 mm and are separated by a gap of 1.4 mm from a radially aligned set of deceleration nozzles whose entrance slit width is 1.45 mm. The chamber between the plate containing the acceleration nozzle and the plate containing the deceleration nozzles contains the sculptured backsides of the acceleration and deceleration nozzles, as shown in . This configuration provides low resistance to gas flow between the nozzles to a circumferential plenum and thence to the two exhaust ports located on the opposite sides of the concentrator. This configuration was designed such that this exhaust gas flowing between the nozzles would have minimal interference with the gas flow profiles within the gap between the acceleration and deceleration nozzles. The minor airflow resistance from the evaporation chamber to the output cone is only 0.8 mm of water. Due to the abrupt change in direction (momentum) of the major air flow through the gap between the acceleration and deceleration nozzles, the pressure drop between the evaporation chamber and exhaust ports is 2.5 cm of water. Thus, as the aerosol output is delivered at a low-positive pressure with extremely low-output resistance, the output flow is dependent on the demand at the output cone. The eddies from the deceleration nozzles relax as the flow of aerosol enters the output cone. The resulting solid-phase aerosol is delivered as a relative low-velocity aerosol (∼2 m/s) through the tapered exit cone.
Materials and methods
Materials
Polyvinylpyrrolidone, PVP, is an excipient that is generally recognized as safe. It is available in a large range of molecular weights that are all highly soluble and non-toxic. These characteristics of PVP enabled the determination of particle size with increasing viscosity and solute concentration. PVP was purchased with molecular weights of 8, 29, 40, 58, and 1300 kDa (Acros Organics, USA). Five percent, 10%, 20%, and 40% aqueous solutions (w/v) of PVP were prepared at room temperature (∼23 °C). To evaluate the generation and processing of surfactant aerosols by SUPRAER-CA, a 10.3% (w/v) synthetic surfactant suspension with the following synthetic phospholipids: POPG (21.3 mg/ml), DPPC (50.4 mg/ml), and POPC (31.3 mg/ml) (weight ratio 2:5:3) in 0.9% saline developed by Waring et al. (Citation2016) was obtained from Molecular Express, Inc. (Rancho Dominguez, CA, USA). The capabilities of SUPRAER-CA to generate respirable aerosols from proteins, antibodies, and macromolecules were demonstrated using bovine serum albumin, bovine gamma globulin (Sigma-Aldrich, USA), and 1.3 MDa PVP (Arcos Organics, USA). Aqueous solutions of 10% albumin, 10% gamma globulin, and 5% 1.3MDa PVP were prepared at room temperature (∼23 °C).
Experimental operational parameters
In the experiments described herein, the dilution airflow was set at 140 l/min and the output flow at 44 l/min. The nozzle air flow was dependent on the size of the aerosol exit orifice being evaluated and the compressed air pressure applied. All the airflow greater than 44 l/min was exhausted through the exhaust ports and the filters on each side of the concentrator. At an input flow of 200 l/min and output flow of 44 l/min (∼2 m/s), the resistance to flow from the evaporation chamber to the exit cone is a remarkably low 0.8 mm of water. The compressed air pressure and dilution air flow rate were selected using gas regulators with their desired temperatures set on their respective PID controllers. The intensity of the infrared radiation (IR) was selected using an IR control.
Measurement of mass median aerodynamic diameter (MMAD) and output
To facilitate spectrographic measurements of the mass distributions within the various stages of the cascade impactor, Allura red (Sigma-Aldrich, USA) was added prior to aerosol generation at a concentration of 4% of the total solids content to the PVP, albumin, and gamma globulin solutions and surfactant suspension. The output cone was connected to the input of a Marple–Miller cascade impactor (Model 150, MSP Co., USA) using a short warmed pipe (∼25.4 mm internal diameter). This five-stage cascade impactor with a filter is specified as Apparatus 2 in the USP and is designed to determine the particle size (aerodynamic size distribution) of dry powder inhalers (DPIs). The aerosol was sampled at a flow rate of 30 l/min. The particles collected on each of the six stages were sequentially dissolved in three 1 ml aliquots of distilled water and pipetted into their respective 4.5 ml PS cuvette. The absorbance in each cuvette was measured at a wavelength of 507 nm by a spectrophotometer (LKB Biochrom, UK). Accordingly, the relative mass on each stage was determined based on the absorbance. The cumulative percentage of mass on each stage was calculated and the log-probability plots created. The MMAD was the 50% cumulative percentage of the hand-fitted line. The geometric standard deviation was the ratio between the aerodynamic diameters at 84% and 50%.
To demonstrate that the colorimetric Allura red assay represented the mass of each of the test agents, a filter was placed in each of stage of the cascade impactor. Following aerosolizing each test agent, the mass on each filter was determined gravimetrically. The particles on each filter were washed off with 3 ml of distilled water into a 4.5 ml PS cuvette. The absorbance in each cuvette was measured by the spectrophotometer. The cumulative mass and percentage for each assay were calculated for 10% (w/v) 8 kDa PVP, albumin, and gamma globulin solutions, and 10.3% (w/v) surfactant suspensions. The MMAD obtained from two methods agreed to within less than 5% for all agents.
The mass of particles at the output of the aerosol generation and delivery system was collected on a low-resistance polymeric electrostatic filter (KB-EF-ES-100) at 44 l/min. The output mass was determined gravimetrically. To ensure the accuracy of the measurement or output mass, two tests were done. First, prior to use, warm air was passed through each filter for 5 min to remove any moisture absorbed within the filter. The weight of filter was measured at 1, 2, 3, 4, and 5 min. The convergence of the weights indicated that the moisture within the filter was removed. In the second test, following particle collection, warm dry air (50 °C) was passed through the filter. The weight of filter was measured after 1, 2, 3, 4 and 5 min. The weight did not change.
Measurement of viscosity
The viscosity of the PVP, albumin, and gamma globulin solutions and surfactant suspension were measured using a capillary rheometer. Briefly, this rheometer is comprised of a four-way fitting with the ports connected to (i) a 15 cm glass capillary tube (0.86 mm, inner diameter 2 R, Harvard Apparatus, MA, USA), and (ii) a pressure transducer (Endevco, Model 8507-2 USA) that measures the pressure difference, , across the capillary tube, (iii) a 1 ml syringe (FMJ-250, Penn-Century Inc., PA, USA) with a syringe pump (Legato, KD Scientific, MA, USA) that controls the fluid flow rate Q and the length L of the fluid pumped in the capillary tube, and (iv) a stopcock. The dynamic viscosity,
, was calculated by the ratio between the shear stress
of the fluid flow in the capillary tube and its rate of strain
.
and
are obtained by
and
, respectively.
Measurement of surfactant surface tension prior to and after aerosolization
To evaluate any degradation of the surface properties of surfactant caused by the aerosolizing and processing of the surfactant suspension, the surface tensions of surfactant suspensions were measured prior to and following aerosolization. Following aerosolization, the collected surfactant aerosols were suspended in water to obtain 2, 4, 6, 8, and 103 mg/ml suspensions; while, the original surfactant suspension was diluted with water to 2, 4, 6, 8, and 103 mg/ml. The FTA-200 Contact Angle Analyzer (First Ten Angstroms, Inc., USA) hanging drop method was used to determine the equilibrium surface tension of surfactant suspensions with concentrations of 2, 4, 6, 8, and 103 mg/ml.
Experimental design
To determine the dependence of SUPRAER-CA’s output aerosol characteristics on (a) nozzle aerosol exit orifice diameter, (b) compressed air pressure, (c) solute concentration, (d) fluid flow rate, and (e) fluid viscosity, a series of experiments were conducted using PVP solutions. All the experiments were performed in triplicate and the average particle size and its standard deviation were calculated for each experiment. To demonstrate the capabilities of SUPRAER-CA to generate fine particle aerosols of proteins, antibodies, surfactant, and high-molecular weight polymers, the MMAD’s and masses of aerosols generated from solutions/suspensions of 10% (w/v) albumin, 10% (w/v) gamma globulin,10.3% (w/v) surfactant, and 5% (w/v) 1.3 MDa PVP were measured. To illustrate the design of experiments, a table of the input parameters and output assays to evaluate the performance of SUPRAER are listed in . The level indicator, 5 is high impact, and 1 is low impact.
Table 1. Design of experiments.
All experiments were conducted using a dilution air flow of 140 l/min. To attain the temperatures within the aerosol plume during aerosolization between 30–40 °C, the temperatures of the compressed air was set at 90 °C and the dilution air at 50 °C. These air temperatures were measured and controlled within the console so they were considerably higher than the temperatures of the air exiting the nozzle and flow distributor, respectively. In a separate study, a fine thermocouple was placed in the region of the plume. When no aerosol was being generated by compressed air at 207 kPa (30 psi) and diluted by air at a flow of 140 l/min, after 5 min, the temperature in the region of the plume was 48 °C. Upon aerosolizing an aqueous fluid at 1 ml/min for 1–5 min under these conditions, the temperature in the region of the plume decreased to 34–38 °C due to evaporative cooling. The transit time of the aerosol in the evaporation chamber was 340 ms. The temperature of the air at the exhaust ports of the concentrator was ∼40 °C. Thus, during an inhalation, the aerosolized agents would be ∼40 °C for about 0.5 s; conditions that are unlikely to degrade either proteins or surfactant. The temperature at an exhaust port was maintained at ∼40 °C for all experiments.
The details of these experiments are summarized in the following sections.
Evaluation of aerosol generation processes in nozzles with aerosol exit orifices with diameters between 150 and 700 μm and their effect on particle size
To evaluate the characteristics of the particles generated by the nozzles with aerosol exit orifice diameters of 150–700 µm, a series of images of aerosol plumes were photographed using high-speed flash macrophotography techniques while generating water aerosols. The photographs were taken using forward light scatters of 25 µs flashes (Nikon Speedlight SB910) of the plume against a black background using a Nikon 810 fitted with a Fotodiox pro adapter, HB-Nik, 2 or 3 Zeiss extension tubes, a 2XE converter, and a Carl Zeiss 120 mm Makroplannar 4/120 lens. The large focal distance (∼70 cm) and depth of field of this configuration enabled the characterization of the plume geometry and detection of the presence of any large particles.
To evaluate the effect of nozzle aerosol exit orifice diameter, KAER’s proprietary nozzles with aerosol exit orifice diameters of 150, 200, 300, 400, 500, 600, and 700 µm (KB-N-xxx) where xxx signifies the aerosol exit diameter were selected to aerosolize 5% and 10% 8 kDa PVP solutions. Nozzles with aerosol exit orifice diameters of 150–400 µm were inserted in a flow distributor with a low-counter flow while nozzles with aerosol exit orifice diameters of 500–700 µm were inserted in a flow distributor with a higher counter flow rate.
The effect of compressed air pressure on particle size
Nozzles with aerosol exit orifice diameters of 300 and 700 µm, KB-N-300 and KB-N-700 (KAER Biotherapeutics), respectively, were each used to aerosolize 10% 8 kDa PVP solution at 1 ml/min. The compressed air pressure was adjusted between 207 and 414 kPa (30 psi and 60 psi). The mean nozzle velocity was calculated from the measured air flow from the nozzle at each pressure and the area of the aerosol exit orifice.
The effect of fluid viscosity on particle size
Using the nozzle with an aerosol exit orifice diameter of 700 µm, 10% and 20% PVP solutions with nominal molecular weights of 8, 29, 40, and 58 kDa were aerosolized at 276 kPa (40 psi) at a fluid flow rate of 1 ml/min.
The effect of solute concentration on particle size
Nozzles with aerosol exit orifice diameters of 300 and 700 µm were each used to aerosolize solutions of 5%, 10%, 20%, and 40% (w/v) 8 kDa PVP with a compressed air pressure of 276 kPa (40 psi) at a fluid flow rate of 1 ml/min.
The effect of fluid flow rate on particle size
Using the nozzle with an aerosol exit orifice diameter of 500 µm, (KB-N-500) 5% and 10% solutions of 8 kDa PVP were aerosolized at 276 kPa (40 psi) at fluid flow rates of 1, 2 and 3 ml/min, respectively.
Generation and delivery of aerosols from solutions/suspensions of albumin, gamma-globulin, surfactant, and 1.3 MDa PVP and their morphologies
Aerosols of the targeted particle sizes (MMAD: 2–5 µm) were generated from 5% 1.3 MDa PVP, 10% albumin, 10% gamma globulin, and 10.3% surfactant solutions/suspensions by the selection of the aerosol exit orifice diameters between 400–700 μm and/or the compressed air pressure between 276–414 kPa (40–60 psi). In addition, the MMAD and geometric standard deviation of aerosols generated from these solutions/suspensions were evaluated using the same operational parameter settings. The morphology of the particles generated from 8 kDa PVP, albumin, and gamma globulin solutions was examined using SEM (Quanta 450, FEI). The output aerosols were collected on a Millipore® filter (diameter: 12.7 mm, pore size: 8 µm, Bedford, MA, USA) and coated with 4-nm-platinum layer using sputter coating machine (QT150, Quorum Technologies, UK) prior to SEM imaging process.
Determination of the output rate, efficiency, and total output deliverable by SUPRAER-CA
Using nozzles with aerosol exit orifice diameters of 500, 600 (KB-N-600), and 700 µm, aerosols were generated from 10% 8 kDa and 5%, 1.3 MDa PVP solutions, 10.3% surfactant, 10% albumin, and 10% gamma-globulin. In addition, 10 and 20 ml of 10% 8 kDa PVP solutions were continuously aerosolized at 3 ml/min with a compressed air pressure of 276 kPa (40 psi) using the nozzle with an aerosol exit orifice diameter of 500 µm. The aerosol was collected in a low-resistance filter bag at the output end of SUPRAER-CA with output flow rate of 44 l/min. The mass of the particles collected was determined gravimetrically.
Evaluation of performance of SUPRAER-CA with on-demand mode
To evaluate the functionality of the nozzles and processing system in an intermittent or “breath” actuated mode, the syringe pump infusion was triggered by a decrease in pressure at the output of SUPRAER-CA during “inspiration”. A “breathing” cycle of 12 breaths/min with an I:E ratio of 1:1 was achieved using sequentially timed rapid activation of normally opened and normally closed (CV = 8) solenoid valves (Model EF80171, Automatic Switch Co., Florham Park, NJ, USA) with the output (“inspiratory”) aerosol flow rate set at 44 l/min. Aerosols were generated from 75 mg PVP of 10% 8 kDa PVP solution by the nozzle with aerosol exit orifice diameter of 500 µm at 276 kPa (40 psi) compressed air pressure with a fluid flow rate of 3 ml/min and the output mass collected on a filter.
Results
Effect of nozzle aerosol exit orifice diameter
When the aerosol exit diameter was smaller than (data not shown) or of similar diameter as the fluid supply channel (230 µm) necking of the fluid exiting the aerosol exit orifice was observed with the formation of a visible aerosol plume external to the aerosol exit orifice. Nozzles with aerosol exit orifice diameters larger than 400 µm and pressures greater than 276 kPa (60 psi), aerosolization of the fluid was seen to occur within an aerosolizing space prior to exiting the aerosol exit orifice. These phenomena are illustrated in a series of images of aerosol plumes generated by nozzles with aerosol exit orifices diameters of 300 µm (KB-N-300) and 700 µm (). The corresponding schematics () were created to clarify the phenomenon as shown in . Aerosol generation using the nozzle with an aerosol exit orifice diameter of 300 µm at 207 kPa (30 psi) and 1 ml/min showed that the fluid jet exited nozzle and broke-up to form the aerosol plume outside the nozzle ( and ). This phenomenon was also observable at 414 kPa (60 psi) with a fluid flow rate of 3 ml/min ( and ). Nozzles with an aerosol exit orifice diameter of ≥400 µm exhibited a transition from the aerosol plume forming, at least predominantly, outside the nozzle to inside the nozzle at compressed air pressures above 276 kPa (40 psi). An example of aerosol generation within an aerosolizing space within the nozzle with an aerosol exit orifice diameter of 700 µm is illustrated in . Aerosol generation was formed within the aerosolizing space within the nozzle and resulted in a columnar-like aerosol plume along the nozzle’s central axis. This columnar-shaped aerosol plume exited the nozzle in a central region of the nozzle surrounded by what appears to be a particle free zone of air flowing between the aerosol plume and the perimeter of the aerosol exit orifice. This particle free zone surrounding the aerosol plume can be seen in and . This phenomenon was also observable at 414 kPa (60 psi) with a fluid flow rate of 3 ml/min ( and ). The particle free zone between the aerosol plume and nozzle edge increased as the aerosol exit orifice diameter increased compared to the fluid supply channel whose diameter was not changed. The aerosol exits the nozzle through a sheath of air devoid of particles eliminated any dripping and clogging under the conditions tested.
Figure 3. (a) Photographs and (b) schematics of aerosol plumes from Nozzle 300 with (a1,b1) 207 kPa (30 psi) at 1 ml/min and with (a2,b2) 414 kPa (60 psi) at 3 ml/min, and Nozzle 700 with (a3,b3) 207 kPa (30 psi) at 1 ml/min and with (a4,b4) 414 kPa (60 psi) at 3 ml/min, respectively. Scale bars represent 2 mm.
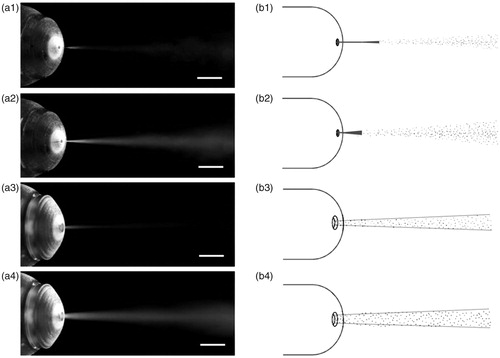
Figure 4. The MMAD of aerosols generated from 5% and 10% 8 kDa PVP solutions at a fluid flow rate of 1 ml/min at compressed air pressure of 276 kPa (40 psi) at the output over the range of aerosol exit orifice diameters.
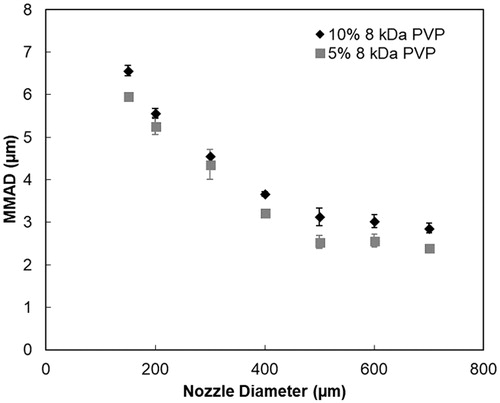
The MMAD of the final solid-phase aerosol decreased with increasing of nozzle aerosol exit orifice diameter (). When the nozzle aerosol exit orifice diameter was increased from 150 (KB-N-150) to 700 µm, aerosolizing 5% 8 kDa PVP solution at a fluid flow rate of 1 ml/min at 276 kPa (40 psi) compressed air pressure, the particle size of the solid-phase output aerosol decreased from 6.0 ± 0.1 µm to 2.4 ± 0 µm. When 10% 8 kDa PVP was aerosolized under the same conditions, the particle MMAD decreased from 6.6 ± 0.1 µm to 2.9 ± 0.1 µm. The geometric standard deviation (σg) of the particle size distributions ranged from 1.5 to 1.9.
Effect of compressed air pressure
Based on the results above, nozzles with aerosol exit orifice diameters of 300 and 700 µm were selected for further evaluation. shows the output particle MMAD’s from 10% 8 kDa PVP solution by the nozzle with an aerosol exit orifice diameter of 300 µm at compressed air pressures of 30, 40, 50, and 414 kPa (60 psi) was 4.9 ± 0.3, 4.6 ± 0.2, 3.7 ± 0.1, and 3.4 ± 0.2 µm, respectively. Corresponding MMAD’s for the nozzle with an aerosol exit orifice diameter of 700 µm were 3.3 ± 0.1, 2.9 ± 0.1, 2.5 ± 0.1, and 2.4 ± 0.2 µm. The geometric standard deviation ranged between 1.6 and 1.8 in all the cases.
Effect of fluid viscosity
The viscosities of 10% albumin, 10% gamma globulin, 10.3% surfactant, and 5% 1.3 MDa PVP solutions/suspensions were 11, 15, 34, and 41 cP, respectively (). To explore the effect of viscosity on MMAD’s, the dynamic viscosities of 10% and 20% PVP solutions were normalized in cSt with consideration of the density of the solution. shows that aerosolizing 10% PVP solutions of 8 kDa, 29 kDa, 40 kDa, and 58 kDa using nozzle with an aerosol exit orifice diameter of 700 µm, the MMAD’s were 2.9 ± 0.1, 2.9 ± 0.3, 3.0 ± 0.2, and 3.1 ± 0.2 µm, respectively. The particles generated from 20% PVP solutions with the same molecular weight, had larger particle size than those generated from 10% PVP solution. The MMAD’s for 20% PVP solutions of 8 kDa, 29 kDa, 40 kDa, and 58 kDa were 3.4 ± 0.4, 3.3 ± 0.2, 3.5 ± 0.3, and 3.8 ± 0.2 µm, respectively. The correlation between viscosity and MMAD was observed with a slope of 0.025 µm/cSt (significance F < 0.001).
Figure 6. The MMAD of aerosols generated at 276 kPa (40 psi) compressed air pressure at a fluid flow rate of 1 ml/min using the nozzle with an aerosol exit orifice diameter of 700 µm corresponding to 10% and 20% PVP solutions over the range of molecular weights.
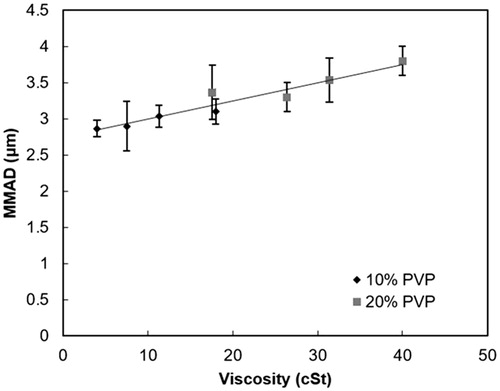
Table 2. Examples of aerosols generated with SUPRAER-CA illustrating its ability to provide a respirable aerosol over a range of compound molecular weights and viscosities.
The effect of solute concentration
As expected, the MMAD of the solid-phase particles increased with increases in the concentration of the dissolved solute in the fluid to be aerosolized. Aerosolizing solutions with concentrations of 5%, 10%, 20%, and 40% 8 kDa PVP using the nozzle with an aerosol exit orifice diameter of 300 µm produced particles with MMAD’s of 4.4 ± 0.4, 4.6 ± 0.2, 5.3 ± 0.1, and 7.1 ± 0.4 µm, respectively (). The geometric standard deviations were between 1.6 and 2.0. The MMAD’s of the aerosols generated from 20% and 40% 8 kDa PVP solutions using this nozzle at 276 kPa (40 psi) were beyond the range of respirable particle size (<5 µm). When using the nozzle with an aerosol exit orifice diameter of 700 µm at 276 kPa (40 psi), the MMAD’s of the generated particles were 2.4 ± 0, 2.9 ± 0.1, 3.4 ± 0.4, and 4.6 ± 0.1 µm, respectively. Notably, all the generated aerosols were within the respirable particle size range. Although the viscosity increases from 4 to 17.6 cP as the concentration of 8 kDa PVP increases from 10% to 20%, the increases in MMAD can be largely accounted for by the increases in solute concentration.
The effect of fluid flow rate
Using the nozzle with an aerosol exit orifice diameter of 500 µm at 276 kPa (40 psi), the MMAD’s increased from 2.5 ± 0.2 to 2.8 ± 0.1 µm as the fluid flow rate of 5% 8 kDa PVP increased from 1 to 3 ml/min. The MMAD’s increased from 3.1 ± 0.2 to 4.2 ± 0.1 µm as the fluid flow rate of 10% 8 kDa PVP increased from 1 to 3 ml/min (), indicative of an increased dependence on flow rate in fluids of higher viscosity.
Generation and delivery of aerosols from solutions/suspensions of albumin, gamma globulin, surfactant, and 1.3 MDa PVP
Through the choice of nozzles with aerosol exit orifice diameters between 400–600 µm and the selection of compressed air pressures of 276–414 kPa (40-60 psi), aerosols generated from 10% albumin, 10% gamma globulin, 10.3% surfactant, and 5% 1.3 MDa PVP at a fluid flow rate of 1 ml/min, had MMAD’s of 2.6–3.6 µm (). For example, 2.6 µm particles were generated from 10.3% surfactant suspension using the nozzle with an aerosol exit orifice diameter of 500 µm with a compressed air pressure of 414 kPa (60 psi) at 1 ml/min. When these solutions/suspensions were aerosolized under the same conditions using the nozzle with an aerosol exit orifice diameter of 400 µm at 276 kPa (40 psi) at 1 ml/min, the MMAD’s were 3.6, 3.2, 3.5, and 5.2 µm for 10% albumin, 10% gamma globulin, 10.3% surfactant, and 5% 1.3 MDa PVP, respectively. A reduction in particle size from 5.2 µm to 3.6 µm MMAD was achieved for the 1.3 MDa PVP solution using the nozzle with an aerosol exit orifice diameter of 600 µm and 414 kPa (60 psi).
The equilibrium surface tensions of 2, 4, 6, 8, and 103 mg/ml surfactant prior to and following aerosolizing with SUPRAER-CA were 30 and 29.4, 22.2 and 22.6, 22.6 and 23.4, 20.3 and 20.9, 20.5 and 21.2 mN/m, respectively. The aerosolizing process did not degrade the surface tension of the surfactant.
Output mass, efficiency, and particle morphologies
The mass output rates and efficiencies for aerosols generated at increasing fluid flow rates for PVP, surfactant, albumin, and gamma globulin using nozzles with aerosol exit orifice diameters between 500–700 µm and air pressures between 276–414 kPa (40–60 psi) are shown in . The nozzles and compressed air pressures were chosen based on the desire to produce respirable aerosols from the solutions/suspensions above. Explicitly, 5% 1.3 MDa PVP solution was aerosolized using a nozzle with aerosol exit diameter of 600 µm at 414 kPa (60 psi); 10% 8 kDa PVP solution and 10.3% surfactant suspension was aerosolized using a nozzle with an aerosol exit orifice diameter of 500 µm at 276 kPa (40 psi) and 10% albumin and gamma globulin solutions were aerosolized using a nozzle with an aerosol exit orifice diameter of 600 µm at 276 kPa (40 psi). Three notable conclusions are evident in : (i) the mass output increased linearly with fluid flow rate without loss in efficiency for 10% 8 kDa PVP, 10.3% surfactant, 10% albumin, and 10% gamma globulin; (ii) these output rates at 3 ml/min were 3.2, 2.8, 3.3, 2.8 mg/sec for 10% 8 kDa PVP, 10.3% surfactant, 10% albumin, and 10% gamma globulin, respectively and, (iii) aerosols from 5% 1.3 MDa PVP generated at 1 ml/min were delivered at an output rate of 0.4 mg/sec with an efficiency of 46%.
Figure 9. Output efficiency (bars) and output rate (symbols) of solid-phase aerosols generated from 10% 8 kDa PVP solution, 10.3% surfactant suspension, 10% albumin, 10% gamma globulin solutions, and 5% 1.3 MDa PVP solution.
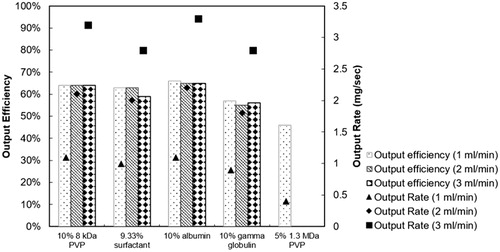
The capability of SUPRAER-CA to deliver high doses of aerosols within the respirable range in a short period was demonstrated at an output flow rate of 44 l/min. Using the nozzle with an aerosol exit orifice diameter of 500 µm at compressed air pressure of 276 kPa (40 psi) to aerosolize 10 ml and 20 ml 10% 8 kDa PVP solutions at a fluid flow rate of 3 ml/min, 0.7, and 1.2 g were collected at the output in 3.3 and 6.7 min, respectively.
SEM images of generated particles show that PVP (), albumin (), and gamma globulin () all have a near-spherical morphology with the dimples suggesting hollow structures. The particles from albumin and gamma globulin solutions had smoother surfaces than that from PVP solution.
Performance of SUPRAER-CA with the production of aerosols only during “inhalation” on demand
In the test of on-demand mode, 44 mg PVP was collected at the output with an efficiency of 58%. As expected, this was marginally lower than the measured 64% when operated with continuous aerosolization.
Discussion
The major advances brought about by this enabling technology include the demonstrated ability through the generation of liquid aerosols with nozzles with large exit orifice diameters together with augmented water evaporation from the droplets using axial counter-flow air, thermal heat transfer and infrared radiation and the concentration of these aerosols using a compact virtual impactor, to deliver high concentrations of 2–4 µm MMAD solid-phase aerosols. These solid-phase aerosols were generated from viscous solutions or suspensions of proteins, polymers, and surfactants. This versatile aerosol generation system represents a new class of aerosol delivery; aqueous liquid to solid phase. This liquid to solid phase aerosol delivery system obviates the need for prior spray drying, the addition of excipients, long-term dry powder storage, and issues related to the aerosolization of dry powders. Certainly, given the range of test agents evaluated herein, excipients could be aerosolized along with the active agent as desired. The inclusion of this technology into a medical device would provide the means to administer clinically relevant doses of agents to the respiratory tract that heretofore was not practical. This need is also present in the treatment of respiratory conditions in equine and livestock. It could result in marked reductions in treatment times and the increase in patient acceptability and related improved outcomes.
This compact aerosol generation and delivery system aerosolizes viscous aqueous fluids and efficiently delivers the non-volatile constituents as highly concentrated pure solid-phase fine particle aerosols at a flow rate of 44 l/min. These aerosols were generated at preselected constant fluid flow rates with single-pass nozzles with aerosol exit orifices between 150 µm and 700 µm in diameter. The columnar axially aligned aerosol plume that exited the orifices ≥400 µm was surrounded by a sheath of apparently particle-free air. The central location of the aerosol plume together with the entrained dilution air from the flow distributor flowing over the convex nozzle surface minimized any deposition of particles in and around the nozzle. Thus, this configuration enabled high throughputs without clogging or dripping. Rapid evaporation of the water from the aerosol droplets was achieved by the combination of generating the aerosol with warm compressed air, the arrest of the aerosol plume by warm counter-flow air and the augmented mixing of this aerosol and further dilution with warm dry air. The counter-flow gas enabled the reduction in the size of the aerosol processing system and the dispersion of the plume, which in turn increased the evaporation of the water from the droplets and limited any coagulation of the aerosol. In addition, infrared radiation within the absorption band of water provided much of the energy for the latent heat of evaporation (540 kcal/g) of water. As a result, evaporative cooling and heat transfer within the droplets resulted in plume temperatures between 34 °C and 40 °C. The temperature of the aerosol flow measured with a thermocouple at the exhaust port of the aerosol concentrator was ∼40 °C.
The absolute humidity of the aerosol at the output was governed by the ratio of the rate of aqueous aerosol generation and the total of the compressed and dilution air flow rates. The absolute humidity was between 5–15 mg/l. Air was removed from the resultant solid-phase aerosol using the radially aligned low-resistance virtual impactor type aerosol concentrator and the resultant concentrated aerosol delivered via the exit cone., This technology provides the ability to deliver respirable aerosols with a controlled water content, although it is not a focus of these investigations.
Although the density of the particles was not measured, rough estimates of the diameter of initial aqueous aerosols of 4–10 µm can be obtained based on the solute concentration (10%, w/v) and the MMAD of the solid-phase aerosols (2–4 µm).
The inverse relationship between nozzle aerosol exit orifice diameter and particle size provides the initial criteria for the selection of the size of the aqueous aerosols generated. Further tailoring of the particle size was achieved through control of the compressed air pressure, solute/suspension concentration, and fluid-flow rate. Outputs of PVP, albumin, gamma globulin, and surfactant aerosols 2.6 µm to 3.6 µm MMAD with narrow size distributions (geometric standard deviation: 1.5–2) were obtained. Of note, the SUPRAER-CA device enabled mass output concentrations of 4 µm MMAD aerosols up to 4.4 mg/l with total mass outputs of 1.2 g in 7 minutes at an output flow rate of 44 l/min. Under the conditions tested herein, our experience demonstrated that even with the intermittent fluid flow, the nozzles were reliable and remained remarkably free from clogging.
Observations on aerosol formation
The nozzles used herein differ from the nozzles used in conventional Venturi jet-type atomizers, where the compressed gas flowing through a central orifice induces a negative pressure, which causes the fluid in a reservoir to flow towards the gas jet with the aerosol being created by an air blast shearing the surrounding fluid. In contrast, the compressed gas in the nozzles used herein converges coaxially on the fluid exiting a central supply channel prior to the aerosol exit orifice such that, at this point, the gas and liquid are ostensibly at the same pressure. The gas pressures inside the nozzles were not measured and are less than the gas pressures measured within the console. As the aerosol exit orifice is sharp-edged, at high-mass gas flows, the sonic gas velocity constraints of choke flow are not met and supersonic gas flow, i.e., Mach number >1, was evident (Kryeziu and Johnson Citation2013) in all the nozzles used herein at both 275 kPa (40 psi) and 414 kPa (60 Psi). Using exit orifices smaller than 400 µm in diameter, an axial fluid jet centered within a high-velocity gas jet as depicted in was similar to that reported by Ganan-Calvo (2005) and Gañán-Calvo and Montanero (Citation2009). The aerosolizing process of this jet appears to occur predominantly downstream from the aerosol exit orifice. These aerosolizing processes likely include Raleigh breakup and aerodynamic loading (Finlay Citation2001). At higher mass gas flows using nozzle exit orifices larger than 400 µm in diameter, there was a transition in the aerosol generating processes with the aerosol generated within an aerosolizing space within the nozzle at pressures above 276 kPa (40 Psi). It is notable that these aerosol-forming processes were constrained to the central core of the gas exiting the aerosol exit orifice and resulted in a relatively columnar aerosol plume confined within an apparently particle-free gas plume that increased with aerosol orifice exit diameter. Since the increase in the size of the particle-free zone increases as the aerosol exit diameter increases beyond the diameter of the fluid supply channel suggests that this concentrically flowing gas does not participate in the aerosolizing processes of the fluid. Of note, this aerosolization methodology avoids the need for the further aerosol breakup on a baffle and any related shear-induced degradation of the solute.
The size of the original droplets generated is a function of the ratio of the mass of liquid to the mass of gas, Weber number (We), and the Ohnesorge number (Oh) for both air blast atomizers (Finlay, Citation2001) and focused flow aerosol generators (Ganan Calvo 2005). Although the geometries of the nozzles described herein differ from these configurations, the general relationship between the original aerosol generated and the ratio of the mass of liquid to the mass of gas is evident. The measured air flow rates exiting the nozzles with aerosol exit diameters of 150 µm, 300 µm, and 700 µm diameter nozzles at 40 psi were 0.6 l/min, 2.2 l/min, and 17 l/min, respectively. At 60 psi, the flow rates of the 300 µm and 700 µm aerosol exit diameters were 4.1 l/min and 26 l/min, respectively. The observed decrease in particle size with increasing aerosol exit orifice diameter, i.e., gas flow () is more pronounced using nozzles with aerosol exit diameters ≤400 µm. Consistent with the concept of the gas in the particle free zone not interacting in the aerosolizing processes, the diameters of the aerosols produced with aerosol exit diameters of 500 µm, 600 µm, and 700 µm appear to be less dependent of gas flow. Increases in mass gas flow through the nozzles are related to the increases in upstream gas density, i.e., pressure. Thus, for a given nozzle, the predictive decrease in particle size with increases in the gas to fluid mass flow rates is evident (). Also, there was an increase in particle size with the increased fluid flow at a constant pressure ().
The modest observed increases in particle diameter shown in over the range of viscosities from 4 to 39 cP are due to the increased damping of viscous forces inhibiting liquid deformation and break-up.
The observed increases in MMAD as the concentration of 8 kDa PVP solution increased from 5 to 40% (), is consistent with the increase in solute mass concentration with the effect of the viscosities of the solutions tested playing a lesser role. The overall consistency of the aerosol outputs with efficiencies of ∼64% from the aerosol processing system at fluid flow rates from 1 to 3 ml/min over the range of test substances, as demonstrated in , attests to the robustness of the technology. When generating aerosols from the 10.3% surfactant suspension, the minor decrease in efficiency to 60% at 1 ml/min with a further reduction to 56 at 3 ml/min may be related to surfactant-induced retardation in evaporation of the water (Gugliotti et al. Citation2005), especially at the higher fluid flow rate.
The scanning electron micrographs show that the final particles have dimpled surfaces indicative of the formation of shell structures. When the diffusion of solute molecules to the droplet’s center is slower than the reduction of droplet surface area during evaporation, the solid-phase particle forms a shell structure with additional shrinking as indicated by the Peclet number (Vehring Citation2008). Large molecules have a small diffusion coefficient and thus tend to be associated with shell/hollow particles. However, the final morphology is also dependent on the physicochemical properties of the solute or suspension as the particles generated from gamma globulin appear smaller than those generated from either 8 kDa PVP or albumin.
Advantages of the technology incorporated in SUPRAER-CA
Compared to breath-driven dry powder inhalers, SUPRAER-CA has the potential to provide constant aerosol generation throughout each and every consecutive breath until the desired dose is inhaled. This reduces the risk of agglomeration at high-aerosol concentrations as may occur with some dry powder inhalers. The potential for repetitive aerosol inhalations eliminates the need to interrupt the treatment to reload capsules. However, the compact size of dry powder inhalers provides a portability advantage. The exclusion of excipients to facilitate aerosolizing dry powders simplifies formulation and eliminates any of their untoward side effects. This can be important when testing new API’s for efficacy and toxicity in animal models. As the aerosol is delivered under positive pressure, its potential to provide inhalation therapy to young children, respiratory compromised patients, and the elderly is clear.
The aerosolizing nozzles overcome a number of limitations inherent in jet-type atomizers and mesh-type nebulizers. All the fluid that enters these single-pass nozzles is aerosolized to form an aqueous aerosol with a relatively narrow size distribution (geometric standard deviation: 1.5–2). The elimination of the need for further droplet break-up on a baffle that is common in jet-type atomizers eliminates this as a cause of shear degradation of the molecular structure of the agent being aerosolized (Lentz et al. Citation2006). The single-pass nozzle also eliminates recirculation and the associated nozzle-associated shearing, concentration build-up, and potential foaming of low-surface tension agents. It is readily apparent that aerosol exit orifice diameters of 400–700 µm, are more amenable to generating aerosols from solutions of higher viscosity than the 2–4 µm diameter orifices utilized in mesh-type nebulizers. Mesh-type nebulizers aerosolize solutions with viscosities below 4–6 CP (Ghazanfari et al. Citation2007; Chan et al. Citation2012; Davies et al. Citation2014). Ultrasonic nebulizers and some jet-type atomizers operate below 6 cP (McCallion et al. Citation1995; Davies et al. Citation2014). Herein, we demonstrate aerosolizing solutions up to 40 cP and surfactant suspensions as high as 34 cP. It is notable that the aerosol generation rate with SUPRAER-CA is about 10 times that reported for jet-type atomizers (Davies et al. Citation2014).
Although the use of a fluid pump adds to the complexity of SUPRAER-CA, its use provides a constant aerosolization rate in contrast to decreasing outputs observed in both jet-type atomizers and mesh-type nebulizers (Hutten et al. 2015). As there is no recirculation of the fluid to be aerosolized, there is no increase in solute or suspension concentration, decrease in output or change in particle size due to an increase in solute concentration and viscosity as observed in both Venturi-type jet atomizers and mesh-type nebulizers. Over the course of a treatment, in these latter devices, these factors can result in decreasing dose rates and prolonged treatment times. Using the atomizing nozzles described herein, neither the fluid to be aerosolized nor the aerosolized particles created come in contact with the aerosol exit orifice. This avoids the associated shear forces and eliminates nozzle clogging and dripping under conditions tested. Although thermal energy is supplied to evaporate the water from the aerosol, the plume temperatures of ∼40 °C are favorable compared to the 150–200 °C reached in a capillary aerosol generator (Shen et al. Citation2004). In the capillary aerosol generator, the fluid is vaporized as it is passed through a heated capillary with the final aerosol comprising the condensate on the nuclei created from the solute or suspension being aerosolized. Altogether, these advantageous factors should lead to both more predictability and reliability of the dose of an aerosolized agent delivered to the patient using the technology described herein.
There are several advantages of initially generating a liquid aerosol and transforming these droplets into a solid-phase respirable aerosol compared to generating a similar-sized aqueous aerosol. The generation of 4–10 µm diameter liquid aerosols requires the creation of considerably less surface area for the same mass of 2–3 µm diameter liquid aerosol. Thus, the shear forces necessary to generate 4–10 µm is a fraction required to generate 2–3 µm diameter aerosols. The volume of a 10 μm diameter liquid particle generated by the aerosol generation and delivery system described herein is 25 times larger than a 3.4 μm diameter particle. It follows that solutions with a concentration of 40 mg/ml of a unit density solute would give solid phase particles of 3.4 μm in diameter. Thus, the dose per 3.4 μm particle is 25 times higher than that generated from a 40 mg/ml solution by a nebulizer used for the delivery of liquid aerosols.
Rapid evaporation of the water from the droplets within a compact space was achieved by warming the compressed air and arresting the aerosol plume with a jet of warm dry counter-flow air that radially dispersed the plume. The confined solid angle of the aerosol plume likely was more effective in the arrest of the plume by the counter-flow jet compared to the generation of a plume with a broader cone. Additional warm dry dilution air from the flow distributor as well as infrared radiation was used to rapidly evaporate the liquid from the aerosol. In case of the surfactant, the resultant aerosol will be solid phase when it is below its transition temperature. This rapid evaporation also reduces the deposition of aerosol on the internal walls of the aerosol processing system. The polished surface of aluminum on the reflector, reflects 95% of the incident infrared radiation and thus reflects infrared radiation transmitted through the chamber back into the chamber. This rapid spray drying process provides the potential for generating solid-phase particles with morphologies such that their density is less than that of solid particles containing the same mass of the solid. Thus, particles can be generated with a smaller aerodynamic diameter while carrying the same drug mass and thus when inhaled, penetrate deeper into the peripheral lung.
To concentrate the aerosol in the evaporation chamber flowing at 150–200 l/min into a practical inhalation rate of 44 l/min, a compact aerodynamically designed, radially aligned multi-slit aerosol concentrator was used. Sculptured parabolic-like inner and outer surfaces of acceleration nozzles and deceleration nozzles provided low resistance to aerosol flow as well as minimized aerosol deposition on these surfaces. The major pressure drop of ∼2.5 cm of water was from the evaporation chamber to the exhaust ports whereas the pressure drop from the chamber to the output was just ∼0.8 mm of water. Thus, the output aerosol was provided at a small positive pressure. As the momentum of the particles carries the particles across the gap between the acceleration nozzles and the deceleration nozzles, the concentration efficiency is particle size dependent. The present aerosol concentrator was designed to concentrate aerosols greater than 1 µm.
SUPRAER-CA was designed for preclinical studies and to derive the specifications of a medical device for use in a clinical or home setting. The aerosol processing system comprises 5 easy to clean components. It is envisaged that the clinical counterpart will be a simple, more compact system without the built-in functional flexibility of SUPRAER-CA. The ability to deliver high concentrations of aerosols in the 2–4 µm will provide clinics with enhanced time-saving aerosol delivery capabilities. When used to deliver aerosols to a patient, a mouthpiece can be connected to the output cone. Alternatively, a snug-fitting silicone face-mask can be connected to the output cone with a flexible tube. In this case, an expiratory port will have a filter and a one-way valve. To minimize waste of the active pharmaceutical ingredient, SUPRAER-CA can be operated in a “demand” mode where the flow of fluid to be aerosolized synchronized with the inhalation while maintaining a high-delivery efficiency.
Limitations of SUPRAER-CA
This technology is likely not suitable for ambulatory use as a small rescue inhaler but rather to provide rapid and efficient aerosol delivery in clinical or domiciliary settings. SUPRAER-CA was manufactured with versatile off-the-shelf components where it is possible to enable the determination of operating conditions and specifications of a more compact unit. A simplified smaller medical device incorporating this technology and clinically related controls could readily be achieved.
When high doses were delivered in a short time, the slits of the concentrator started to clog after 1 g particles were collected. This may be, in part, due to the static electricity on the plastic concentrator. Some liquid particles that did not completely evaporate may have deposited on or within the concentrator. Also, liquid aerosols that deposit on the surface of counter-flow tube decrease the output efficiency.
The effects of concentration and viscosity of the aerosolized surrogates on the output particles characteristics were evaluated. However, additional physicochemical properties of the formulations to be aerosolized such as surface tension, molecular weight, and macromolecular structure also may affect the particle characteristics at the output. In this study, the selection of nozzles and compressed air pressure to generate respirable aerosols was determined experimentally. For instance, MMAD’s of particles from 10% PVP (58 kDa, 64 mN/m) and 10% albumin (70 kDa, 49 mN/m) solutions were 4.3 and 3.6 µm, respectively, using the nozzle with an aerosol exit orifice diameter of 400 µm at 276 kPa (40 psi) at 1 ml/min. This is consistent with the larger particles being generated from 10% 58 kDa PVP solution with larger surface tension. To decrease MMAD of particles generated from this PVP solution, the nozzle with an aerosol exit orifice diameter of 700 µm was used to generate 3.1 µm MMAD particles. It is also likely that the macromolecular structure of the 5% 1.3 MDa PVP solution resulted in a larger particle size (∼5.2 µm), using the nozzle with an aerosol exit orifice diameter of 400 µm at compressed air pressure 276 kPa (40 psi) and 1 ml/min fluid flow rate) than predicted based on the particles sizes obtained with the other agents tested herein. This is likely due to macromolecular structure-induced retardation of the aerosolization efficiency of the nozzle.
It is considered unlikely that thermal degradation of proteins aerosolized with SUPRAER-CA is a major issue. Although the 90° temperature of the compressed air set on the control panel may appear of concern, the temperature of the air in the aerosol plume and counter-flow is ∼40 °C due to, (a) cooling between the temperature sensor and the evaporation chamber, (b) adiabatic expansion of the compressed gas in the nozzle and counter-flow tube and (c) evaporative cooling due to the substantial 540 cal/gm latent heat of evaporation. As discussed above, a thermocouple placed in the region of the plume showed that the temperature of the aerosol plume was 34–38 °C. The transit time through the evaporation chamber was 340 ms and the temperature at the output <40 °C. The temperature of the particles does not reach the temperature of the air until evaporation of the water from the surfaces of the particles ceases. Thus, any thermal degradation is likely to be minimal. This is consistent with our preliminary data showing retention of enzymatic (Yeates Citation2012), structural, and immunologically functional integrity (Yeates Citation2013). The protein, insulin, together with its excipients, was spray dried at an inlet temperature of 181 °C and an outlet temperature of 87 °C (Sadrzadeh et al. Citation2010). Monoclonal antibodies, with and without excipients, have been spray dried with an inlet temperature of 100 °C while largely maintaining their monomeric structure and without fragmentation (Ramezani et al. Citation2014). The effect of spray drying albumin with and without trehalose or histidine-HCl buffer of the temperature range of 80–100 °C demonstrated that bovine serum albumin stability with these excipients was maintained during processing at 100 °C (Rajagopal et al. Citation2013). Pulmonary surfactant has been spray-dried, for 60–200° and preferably 85–150 °C (Eistetter Citation2001; Taut Citation2006). High temperatures required to produce dry protein powders to improve long-term storage (Maa et al. Citation1998) are not an issue in this application. The generation of respirable aerosols under less severe conditions as described herein support the potential for this technology to be used to deliver aerosols comprised of functional proteins and surfactant to the respiratory tract. Notwithstanding these data, the formulation, structural, and functional studies of each agent and their combined germane physicochemical and functional properties should be evaluated prior and following aerosolizing and processing prior to their use in clinical studies.
Conclusion
These studies indicate that the technology incorporated in SUPRAER-CA expands the potential of aerosol drug delivery in the following aspects: (i) it extends the viscosity (e.g., 40 cSt), molecular weight (e.g., 1.3 MDa), and concentration range (e.g., 40%) deliverable in a respirable aerosol; (ii) it delivers these respirable aerosols at high-dose rates; (iii) it enables the optimal selection of particle size and aerosol delivery rate for an active agent comprising a protein, antibody, surfactant, or polymer; and (iv) it minimizes any shear degradation and thus retains the physical properties of the drug such as surfactant’s surface tension.
This technology addresses the long-standing need to deliver clinically relevant doses of surfactant, and anti-infectives to the peripheral airways of the lungs. In adults, this can require aerosol delivery of 300 mg to 1 g of the active agent with a mass median aerodynamic diameter (MMAD) of less than 4 µm. More recently, the potential for the delivery of aerosolized antiproteases, molecular constructs, monoclonal antibodies, and secretomes have become potential therapeutic agents to treat ailments of the lungs. Adherence to an inhalation regime in a domiciliary setting and expeditious drug delivery in a clinical setting are important for acceptance by the patient and physician alike as well as result in a major impact on the efficacy of the treatment of the patient. These factors provide the drive for new applications exploiting the new innovations in aerosol delivery as herein described.
Disclosure statement
Xin Heng and Donovan B. Yeates conducted these studies as employees and shareholders of KAER Biotherapeutics Corporation. The technologies described herein are protected by a substantial international patent portfolio of which KAER Biotherapeutics is the beneficiary.
The content of the research reported in this publication is solely the responsibility of the authors and does not necessarily represent the official views of the National Institutes of Health.
Additional information
Funding
References
- Anzueto, A., Baughman, R. P., Guntupalli, K. K., Weg, J. G., Wiedemann, H. P., Raventos, A. A., Lemaire, F., Long, W., Zaccardelli, D. S., and Pattishall, E. N. (1996). Aerosolized Surfactant in Adults with sepsis-induced acute respiratory distress syndrome. Exosurf Acute Respiratory Distress Syndrome Sepsis Study Group. N. Engl. J. Med., 334:1417–1422.
- Brand, P., Schulte, M., Wencker, M., Herpich, C. H., Klein, G., Hanna, K., and Meyer, T. (2009). Lung Deposition of Inhaled Alpha1-Proteinase Inhibitor in Cystic Fibrosis and Alpha1-Antitrypsin Deficiency . Eur. Respir. J., 34:354–360.
- Chan, J. G., Traini, D., Chan, H. K., Young, P. M., and Kwok, P. C. (2012). Delivery of High Solubility Polyols by Vibrating Mesh Nebulizer to Enhance Mucociliary Clearance. J. Aerosol Med. Pulm. Drug. Deliv., 25:297–305.
- Chan, J. G. Y., Tyne, A. S., Pang, A., Chan H. K., Young, P. M., Britton, W. J., Duke, C. C., and Traini, D. (2014). A Rifapentine-Containing Inhaled Triple Antibiotic Formulation for Rapid Treatment of Tubercular Infection. Pharm. Res., 31:1239.
- Clancy, J. P., Dupont, L., Konstan, M. W., Billings, J., Fustik, S., Goss, C. H., Lymp, J., Minic, P., Quittner, A. L., Rubenstein, R. C., Young, K. R., Saiman, L., Burns, J. L., Govan, J. R. W., Ramsey, B., and Gupta, R. (2013). Phase II Studies of Nebulised Arikace in CF Patients with Pseudomonas aeruginosa Infection. Thorax, 68:818–825.
- Das, S., Tucker, I., and Stewart, P. (2015). Inhaled Dry Powder Formulations for Treating Tuberculosis. Curr Drug Deliv, 12:26–39.
- Davies, L. A., Nunez-Alonso, G. A., McLachlan, G., Hyde, S. C., and Gill, D. R. (2014). Aerosol Delivery of DNA/Liposomes to the Lung for Cystic Fibrosis Gene Therapy. Hum. Gene Ther. Clin. Dev., 25:97–107.
- Dushianthan, A., Goss, V., Cusack, R., Grocott, M. P., and Postle, A. D. (2014). Altered Molecular Specificity of Surfactant Phosphatidycholine Synthesis in Patients with Acute Respiratory Distress Syndrome. Respir. Res., 15:128.
- Eistetter, K. (2001). Process for the production of powdered pulmonary surfactant preparations. Patent No US 6215983.
- Finlay, W. H. (2001). The Mechanics of Inhaled Pharmaceutical Aerosols: An Introduction. London: Academic Press.
- Frederiksen, B., Pressler, T., Hansen, A., Koch, C., and Høiby, N. (2006). Effect of Aerosolized rhDNase (Pulmozyme) on Pulmonary Colonization in Patients with Cystic Fibrosis. Acta Paediatr., 95:1070–1074.
- Gañán-Calvo, A. M. (2005). Enhanced Liquid Atomization: From Flow-Focusing to Flow-Blurring. Appl. Phys. Lett., 86:214101.
- Gañán-Calvo, A. M., and Montanero, J. M. (2009). Revision of Capillary Cone-Jet Physics: electrospray and Flow Focusing. Phys. Rev. E Stat. Nonlin. Soft Matter Phys., 79:066305
- Ghazanfari, T., Elhissi, A. M., Ding, Z., and Taylor, K. M. (2007). The Influence of Fluid Physicochemical Properties on vibrating-mesh nebulization. Int. J. Pharm., 339:103–111.
- Gordon, M. S., Thomas, T., and Weers, J. (2003). Inhalation lung surfactant therapy. Patent application 60333729.
- Griese, M., and Scheuch, G. (2016). Delivery of Alpha-1 Antitrypsin to Airways. Annals Ats., 13:S346–S351.
- Gugliotti, M., Baptista, M. S., and Politi, M. J. (2005). Reduction of Evaporation of Natural Water Samples by Monomolecular Films. J. Braz. Chem. Soc., 16:1186–1190.
- Guilleminault, L., Azzopardi, N., Arnoult, C., Sobilo, J., Hervé, V., Montharu, J., Guillon, A., Andres, C., Herault, O., Le Pape, A., Diot, P., Lemarié, E., Paintaud, G., Gouilleux-Gruart, V., and Heuzé-Vourc'h, N. (2014). Fate of Inhaled Monoclonal Antibodies after the Deposition of Aerosolized Particles in the Respiratory System. J Control Release, 196:344–354.
- Gurjar, M. (2015). Colistin for Lung Infection: An Update. J Intensive Care, 3:3.
- Hsiang, L. P., and Faeth, G. M. (1992). Near-Limit Drop Deformation and Secondary Breakup. Int. J. Multiph. Flow, 18:635–652.
- Hütten, M. C., Kuypers, E., Ophelders, D. R., Nikiforou, M., Jellema, R. K., Niemarkt, H. J., Fuchs, C., Tservistas, M., Razetti, R., Bianco, F., and Kramer, B. W. (2015). Nebulization of Poractant Alfa via a Vibrating Membrane Nebulizer in Spontaneously Breathing Preterm Lambs with Binasal Continuous Positive Pressure Ventilation. Pediatr. Res., 78:664–669.
- Joshi, N., Shirsath, N., Singh, A., Joshi, K. S., and Banerjee, R. (2014). Endogenous Lung Surfactant Inspired pH Responsive Nanovesicle Aerosols: Pulmonary Compatible and Site-Specific Drug Delivery in Lung metastases. Sci. Rep., 4:7085.
- Kryeziu, O., and Johnson, E. R. (2013). Subsonic to Supersonic Nozzle Flows. SIAM J. Appl. Math, 73:175–194.
- Lehofer, B., Bloder, F., Jain, P. P., Marsh, L. M., Leitinger, G., Olschewski, H., Leber, R., Olschewski, A., and Prassl, R. (2014). Impact of Atomization Technique on the Stability and Transport Efficiency of Nebulized Liposomes Harboring Different Surface Characteristics. Eur. J. Pharm. Biopharm., 88:1076–1085.
- Lentz, Y. K., Anchordoquy, T. J., and Lengsfeld, C. S. (2006). Rationale for the Selection of an Aerosol Delivery System for Gene delivery. J. Aerosol Med., 19:372–384.
- Lewis, J. F., Ikegami, M., Jobe, A. H., and Tabor, B. (1991). Aerosolized Surfactant Treatment of Preterm Lambs. J. Appl. Physiol., 70:869–876.
- Liu, C., Zhang, Y. T., Peng, Z. Y., Zhou, Q., Hu, B., Zhou, H., and Li, J. G. (2017). Aerosolized Amikacin as Adjunctive Therapy of Ventilator-Associated Pneumonia Caused by Multidrug-Resistant Gram-Negative Bacteria: A Single-Center Randomized Controlled Trial. Chin. Med. J., 130:1196.
- Maa, Y. F., Nguyen, P. A., Andya, J. D., Dasovich, N., Sweeney, T. D., Shire, S. J., and Hsu, C. C. (1998). Effect of Spray Drying and Subsequent Processing Conditions on Residual Moisture Content and Physical/Biochemical Stability of Protein Inhalation Powders. Pharm. Res., 15:768–775.
- Maillet, A., Guilleminault, L., Lemarié, E., Lerondel, S., Azzopardi, N., Montharu, J., Congy-Jolivet, N., Reverdiau, P., Legrain, B., Parent, C., Douvin, D.-H., Hureaux, J., Courty, Y., De Monte, M., Diot, P., Paintaud, G., Le Pape, A., Watier, H., and Heuzé-Vourc'h, N. (2011). The Airways, a Novel Route for Delivering Monoclonal Antibodies to Treat Lung Tumors. Pharm. Res., 28:2147–2156.
- Majithia, A. K., Hall, S., Harper, L., and Bowen, P. J. (2008). Droplet breakup quantification and processes in constant and pulsed air flows. In Proceedings of the 22nd Conference on Liquid Atomization and Spray Systems (ILASS-Europe), Como Lake, Italy, September 8–10.
- Maselli, D. J., Keyt, H., and Restrepo, M. I. (2017). Inhaled Antibiotic Therapy in Chronic Respiratory Diseases. IJMS., 18:1062.
- McCallion, O. N., Taylor, K. M., Thomas, M., and Taylor, A. J. (1995). Nebulization of Fluids of Different Physicochemical Properties with Air-Jet and Ultrasonic Nebulizers. Pharm. Res., 12:1682–1688.
- Pohlmann, G., Iwatschenko, P., Koch, W., Windt, H., Rast, M., de Abreu, M. G., Taut, F. J., and De Muynck, C. (2013). A Novel Continuous Powder Aerosolizer (CPA) for Inhalative Administration of Highly Concentrated Recombinant Surfactant Protein-C (rSP-C) Surfactant to Preterm Neonates. J. Aerosol Med. Pulm. Drug Deliv., 26:370–379.
- Rahmel, D. K., Pohlmann, G., Iwatschenko, P., Volland, J., Liebisch, S., Kock, H., Mecklenburg, L., Maurer, C., Kemkowski, J., and Taut, F. J. (2012). The Non-Intubated, Spontaneously Breathing, Continuous Positive Airway Pressure (CPAP) Ventilated Pre-Term Lamb: A Unique Animal Model. Reprod. Toxicol., 34:204–215.
- Rajagopal, K., Wood, J., Tran, B., Patapoff, T. W., and Nivaggioli, T. (2013). Trehalose Limits BSA Aggregation in Spray-Dried Formulations at High Temperatures: Implications in Preparing Polymer Implants for Long-Term Protein Delivery. J. Pharm. Sci., 102:2655–2666.
- Ramezani, V., Vatanara, A., Najafabadi, A. R., Shokrgozar, M. A., Khabiri, A., and Seyedabadi, M. (2014). A Comparative Study on the Physicochemical and Biological Stability of IgG 1 and Monoclonal Antibodies during Spray Drying Process. Daru J., 22:31.
- Ricci, F., Salomone, F., Kuypers, E., Ophelders, D., Nikiforou, M., Willems, M., Krieger, T., Murgia, X., Hutten, M., Kramer, B. W., and Bianco, F. (2017). In Vivo Evaluation of the Acute Pulmonary Response to Poractant Alfa and Bovactant Treatments in Lung-Lavaged Adult Rabbits and in Preterm Lambs with Respiratory Distress Syndrome. Front. Pediatr., 5:186.
- Rijnders, B. J., Cornelissen, J. J., Slobbe, L., Becker, M. J., Doorduijn, J. K., Hop, W. C. J., Ruijgrok, E. J., Löwenberg, B., Vulto, A., Lugtenburg, P. J., and de Marie, S. (2008). Aerosolized Liposomal Amphotericin B for the Prevention of Invasive Pulmonary Aspergillosis during Prolonged Neutropenia: A Randomized, placebo-controlled trial. Clin. Infect. Dis., 46:1401–1408.
- Rudokas, M., Najlah, M., Alhnan, M. A., and Elhissi, A. (2016). Liposome Delivery Systems for Inhalation: A Critical Review Highlighting Formulation Issues and Anticancer Applications. Med. Princ. Pract., 25:60–72.
- Sadrzadeh, N., Miller, D. P., Lechuga-Ballesteros, D., Harper, N. J., Stevenson, C. L., and Bennett, D. B. (2010). Solid-State Stability of Spray-Dried Insulin Powder for Inhalation: Chemical Kinetics and Structural Relaxation Modeling of Exubera above and below the Glass Transition Temperature. J. Pharm. Sci., 99:3698–3710.
- Shen, X., Hindle, M., and Byron, P. R. (2004). Effect of Energy on Propylene Glycol Aerosols Using the Capillary Aerosol Generator. Int. J. Pharm., 275:249–258.
- Taut, F. (2006). Use of pulmonary surfactant. US Patent No 6982075.
- Tomioka, H., Kuwata, Y., Imanaka, K., Hashimoto, K., Ohnishi, H., Tada, K., Sakamoto, H., and Iwasaki, H. (2005). A Pilot Study of Aerosolized N-acetylcysteine for idiopathic pulmonary fibrosis. Respirology, 10:449–455.
- Vehring, R. (2008). Pharmaceutical Particle Engineering via Spray Drying. Pharm. Res., 25:999–1022.
- Walther, F. J., Hernandez-Juviel, J. M., and Waring, A. J. (2014). Aerosol Delivery of Synthetic Lung Surfactant. PeerJ. 2:e403.
- Waring, A. J., Gupta, M., Gordon, L. M., Fujii, G., and Walther, F. J. (2016). Stability of an Amphipathic Helix-Hairpin Surfactant Peptide in Liposomes. Biochem. Biophys. Acta. Biomembranes, 1858:3113–3119.
- Weber, A., Morlin, G., Cohen, M., Williams-Warren, J., Ramsey, B., and Smith, A. (1997). Effect of Nebulizer Type and Antibiotic Concentration on Device performance. Pediatr. Pulmonol., 23:249–260.
- Weers, J., and Tarara, T. (2014). The PulmoSphere™ Platform for Pulmonary Drug Delivery. Ther. Deliv. 5:277–295.
- Yeates, D. B. (2012). A Platform for High Dose Rate Delivery of Biotherapeutics. [Abstract]. Presented at Respiratory Drug Delivery conference, Phoenix, Arizona. Respiratory Drug Delivery. 565-568.
- Yeates, D. B. (2013a). High Dose Rate Generation of Fine Particle Aerosols of Antibodies with SUPRAER® Compared to Two Mesh Nebulizers. Resp. Drug Deliv., 313–316.
- Yeates, D. B. (2013b). Generation of Respirable Antibody Aerosols. J. Aerosol Med. Pulm. Drug Deliv., 26 (2):P090.
- Yeates, D. B. (2018). Surfactant Aerosol Therapy for nRDS and ARDS, in Inhalation Aerosols, Physical and Biological Basis for Therapy, A.J. Hickey and H. M. Mansour, eds., 3rd ed., Boca Raton: InformaHealthCare/CRC Press/Taylor & Francis (In Press).
- Yeates, D. B., and Heng, X. (2016a). Generation of Respirable Particles from Surfactant Suspensions and Viscous Solutions at High Dose Rates. [Abstract]. Presented at Drug Delivery to the Lungs 27, Edinburgh, UK, December. Drug Deliv. Lungs, 205–208.
- Yeates, D. B., and Heng, X. (2016b). Targeting the Optimal Particles Size and Output for Aerosol Drug Delivery Using SUPRAER®. [Abstract]. Presented at Respiratory Drug Delivery conference, Phoenix, Arizona, April. Resp. Drug Deliv., 395–400.
- Yeates, D. B., and Heng, X. (2017). Augmentation of the Generation, Processing and Delivery of Surfactant and Macromolecule Aerosols with Heliox. [Abstract]. Presented at 21 ISAM-Congress, Santa Fe, NM, June. J. Aerosol Med. Pulm. Drug. Deliv., 30(3):164.
- Young, P. M., Thompson, J., Woodcock, D., Aydin, M., and Price, R. (2004). The Development of a Novel High-Dose Pressurized Aerosol Dry-Powder Device (PADD) for the Delivery of Pumactant for Inhalation Therapy. J. Aerosol Med., 17:123–128.
- Zhu, B., Young, P. M., Ong, H. X., Crapper, J., Flodin, C., Qiao, E. L., Phillips, G., and Traini, D. (2015). Tuning Aerosol Performance Using the Multibreath Orbital® Dry Powder Inhaler Device: Controlling Delivery Parameters and Aerosol Performance via Modification of Puck Orifice Geometry. J. Pharm. Sci., 104:2169–2176.