Abstract
Dicarboxylic acids are prevalent in the atmosphere and frequently investigated by aerosol and atmospheric scientists. Glutaric acid, being a water-soluble dicarboxylic acid, is commonly used to model water activity in dicarboxylic acid aerosols. Different values associated with glutaric acid aerosols, specifically DRH and hygroscopic data, have been reported by several groups. We hypothesize that this variability is caused by its polymorphism. Glutaric acid exhibits dimorphism, meaning the molecule can form into two different types of crystal structures, an alpha and a beta polymorph. Glutaric acid naturally forms the beta polymorph, which is more stable. The alpha polymorph is the metastable phase. These polymorphs manifest themselves as a bimodal aerosol size distribution when analyzed by Scanning Mobility Particle Sizers or other aerosol mobility sizing methods. In this article, we discuss the formation of the nanoscale alpha glutaric acid polymorph generated from an aqueous glutaric acid solution. The formation of both polymorphs from an aqueous solution has not been documented elsewhere. We have found that the alpha polymorph forms at faster crystallization speeds, with a lower aqueous solution concentration and a lower solution flow rate into the atomizer. This identification of the presence of polymorphism in glutaric acid aerosols under common laboratory generation conditions could explain the variability present in studies associated with glutaric acid aerosols. Understanding the conditions at which the alpha polymorph is present will allow researchers to avoid it when using glutaric acid as a dicarboxylic acid standard and explain previously noted discrepancies.
Copyright © 2019 American Association for Aerosol Research
1. Introduction
To accurately model climate and the atmosphere, the behavior of aerosols must be well understood. The presence of aerosols in the atmosphere plays a large role in cloud formation and therefore climate forcing. Clouds and aerosols are the largest sources of uncertainty in the understanding of climate change (Boucher Citation2013). These organic aerosols typically make up 20%–80% of the fine particles (by mass) in the atmosphere (Goldstein et al. Citation2008; Saxena and Hildemann Citation1996). Within the atmosphere, aerosols have three significant functions. The first is the direct effect, which is aerosols’ ability to scatter light. The indirect aerosol effect is the aerosols' cloud condensation nuclei (CCN) activity or their ability to take on water to form clouds. Lastly, the aerosols play a large role in atmospheric chemistry (Seinfeld and Pandis Citation2016).
Dicarboxylic acids make up a significant portion of atmospheric aerosols (Saxena and Hildemann Citation1996). Glutaric acid, an abundant, water-soluble dicarboxylic acid, is found in the atmosphere all over the globe (Limbeck and Puxbaum Citation1999). Glutaric acid is frequently used as a proxy for understanding less ubiquitous and laboratory intractable dicarboxylic acids (Pope et al. Citation2010). Many properties of glutaric acid have been documented such as hygroscopicity (Pope et al. Citation2010; Cruz and Pandis Citation2000; Chan and Chan Citation2005; Peng, Chan, and Chan Citation2001; Wise et al. Citation2003; Choi and Chan Citation2002; Zardini et al. Citation2008) and CCN activity with respect to Khler theory (Kawamura and Bikkina Citation2016; Pradeep Kumar, Broekhuizen, and Abbatt Citation2003). Glutaric acid is also used in the aerosol field to study its hygroscopic effects in the presence of inorganic aerosols, such as sodium chloride (Pope et al. Citation2010; Pant et al. Citation2004) and ammonium sulfate (Pant et al. Citation2004). The effects when coating other aerosols, such as soot (Xue et al. Citation2009a, Citation2009b) and ammonium sulfate (Cruz and Pandis Citation1998), have also been documented. Along with many other organic aerosols, the deliquescence relative humidity (DRH) of glutaric acid is documented (Pope et al. Citation2010; Pant et al. Citation2004; Parsons et al. Citation2004). Lastly, along with its presence in different atmospheres (Limbeck and Puxbaum Citation1999), its natural chemical formation from other particles in the atmosphere has been studied (Ervens et al. Citation2004; Hamilton et al. Citation2006).
However, a significant amount of variability in reported DRH and hygroscopicities has been reported in the literature with varying hypothesized explanations including glutaric acid’s higher vapor pressure, the possibility of void spaces, trapped internal water, impurities, or an amorphous structure that would affect water solubility (Hori et al. Citation2003; Bilde and Svenningsson Citation2004; Mikhailov et al. Citation2009; Bilde et al. Citation2015). While other researchers have specifically proposed that variations in reported literature data or deviations within their own experiments may be due to aerosol generation methods and/or drying rates (Wang et al. Citation2010; Sullivan et al. Citation2010; Altaf and Freedman Citation2017), we specifically propose that this variability is ultimately related to glutaric acid’s dimorphism (Yeung, Ling, and Chan Citation2010). As one example specific to glutaric acid, Pope et al. (Citation2010) pointed out the discrepancy between the hygroscopic trends reported between Peng et al. (Citation2001) and Cruz and Pandis (Citation2000) where growth factors reported after deliquescence differed by 20% between these studies.
Earlier experiments demonstrated that while glutaric acid can form a bimodal distribution under certain circumstances, the dry particles can be selected by mobility size in one DMA, rehumidified in a Nafion conditioning tube, and subsequently re-dried at a slow rate to produce a single-mode distribution (Phan Citation2015). These experiments showed that regardless of whether the smaller or larger peak size was selected, the particles resulting from the humidification cycling were all of identical mobility size which was in between the sizes selected from the two-mode peaks. This work led us to believe that the bimodal peak was actually two different polymorphs of glutaric acid with different mobility sizes but identical masses, rather than a simple vaporization of the smallest and perhaps more volatile sizes from the original distribution.
Glutaric acid exhibits dimorphism, which means it is a polymorph that can form two distinct crystalline structures (Myerson Citation2002; Ha et al. Citation2009). Glutaric acid has experimentally been found to form an alpha and a beta polymorph. The beta is the stable phase that naturally occurs. The alpha polymorph is the metastable phase, meaning it is less stable and with time will typically revert back to the liquid phase, and the beta polymorph as is expected under Ostwald’s rule (Espeau, Negrier, and Corvis Citation2013). Furthermore, the alpha polymorph tends to be favored at higher temperatures and is more soluble in water than the beta phase. The transition temperature from the beta to alpha polymorph has experimentally been determined to be 63 °C (Yeung, Ling, and Chan Citation2010), 68 °C (Espeau, Negrier, and Corvis Citation2013), and 76 °C (Ha et al. Citation2009). The alpha polymorph has also been formed in other laboratories at a lower RH, using non-aqueous solvents such as methanol (Ha et al. Citation2009), and with other particles present (Yeung, Ling, and Chan Citation2010).
We investigated the formation of glutaric acid polymorphs in nanoscale aerosols generated from an aqueous glutaric acid solution using common laboratory aerosol generation equipment. Identifying the presence of polymorphism in glutaric acid aerosols will ideally explain the variability present in studies associated with glutaric acid aerosols. We further theorized that the particles are not spherical based on the presence of two mobility size modes. Because the initial size distribution leaving the atomizer is constant, the dual mode distribution infers a differing shape factor (Tavakoli and Olfert Citation2014). Additional factors that may also influence mobility beyond simple crystal shapes may include porosity or more complicated particle structures.
2. Methods
2.1. Materials
99% glutaric acid from Sigma-Aldrich (St Louis, MO, USA) was used for all solutions. Ultra-pure water furthered purified with a Millipore Simplicity UV filter (Burlington, MA, USA) was also used in to create these solutions.
2.2. Apparatus
The formation of the alpha and beta polymorph glutaric aerosols was investigated using the equipment setup shown in .
The particles are created as the aqueous solution of glutaric acid is ejected from a syringe into an aerosol generator (TSI 3076) known to provide a constant and stable flow for aerosol atomization of solutions (Liu and Lee Citation1975). We have verified the stability at the concentrations and flow rates used here for glutaric acid using ammonium sulfate solutions of identical concentrations. These newly created solution particles, suspended in a purified air stream (TSI 3074B air purifier and dryer) which are initially ∼2 μm in diameter, are then dried through a TSI model 306200 diffusion dryer followed by a series of four custom diffusion dryers. The liquid solution particles shrink via water evaporation while in the dryers. Each of the four dryers is a meter long, with 5-cm-radii tubes—two filled with silica gel and the other two with zeolites, each with a clear annular space. A HEPA filter was present before the dryers acting as a vent to equalize all pressures and flows. The particles were then collected in a PIXE Model 1-1 L Cascade Impactor (PIXE International Corporation Citation2018), while being measured by a Scanning Mobility Particle Sizer (SMPS) system, comprised of a CPC (TSI 3776) (Stolzenburg and McMurry Citation1991; McMurry Citation2000) and a DMA (TSI 3080) (Knutson and Whitby Citation1975).
2.2.1. SMPS
The particles were run through the SMPS setup consisting of a differential mobility analyzer (DMA) and a condensation particle counter (CPC), with a sample flow rate of 0.3 LPM and a sheath flow of 5.0 LPM, to obtain the SMPS data. The SMPS system gives the particle mobility size distribution of the particles formed throughout the previously mentioned process. We used scan times of either 120 or 180 seconds up and 15 seconds down. The instruments were controlled with TSI Aerosol Instrument Manager Software (AIM Version 9.0) and were analyzed using the multiple charging and diffusion corrections. These corrections account for the behavior of idealized particle systems composed of spherical particles. It should be noted that the more particles deviate from spherical, the more errors may be contained in the corrections; however, not using the corrections will also create errors in the data.
2.2.2. PXRD sampling
In this experiment, samples were collected in order to analyze the polymorphs present under different conditions using Powder X-Ray Diffraction (PXRD). The PXRD was used because it has the capacity to distinguish between polymorphs of aerosols and to compare samples to databases of preexisting diffractograms to identify the crystals present. PXRD is also able to distinguish clearly between amorphous phases, crystalline phases, or combinations of the two (Brundle et al. Citation1992).
These samples were collected in parallel with the SMPS using a PIXE Cascade Impactor, as shown in , at a flow rate of 1.0 LPM with a standard cutoff diameter of 60 nm. The lowest stage on the PIXE cascade impactor was altered by removing the three legs, so that the PXRD disk was situated in the base of the impactor. This adjustment somewhat lowered the cutoff diameter below 60 nm and allowed many particles to be collected directly onto the PXRD disk. The samples were then collected continuously over a period of 24 hours; the syringe was replenished with more solution once it emptied, approximately every hour. The PXRD was then run for 18 hours, consisting of 1290 scans of 50 seconds scanning from the position 5 to 50 2θ. This is a relatively long run because the sample is on the smaller scale of typical PXRD samples and the PXRD needs more time to identify peaks. We were able to reduce the range of the angles scanned based on the angles of interest on the preexisting diffractograms for both polymorphs of glutaric acid.
3. Results and discussion
demonstrates the association of the two distinct SMPS peaks and the two glutaric acid polymorphs identified by PXRD. The PXRD diffractograms also show definitively that only specific peaks are present and that no amorphous phase exists in any of the samples analyzed. The lack of an amorphous phase rules out the possibility that the generated samples are anything other than crystalline.
Figure 2. SMPS data (left) and PXRD diffractograms (right) for aerosols generated from three different concentrations of aqueous glutaric acid when the syringe flow rate was 0.35 mL per min. At 0.50 g/L in the feed solution, only the alpha polymorph of glutaric acid is present. The PXRD data for this corresponding alpha polymorph marked with an asterisk are drawn from the Cambridge Structural Database (GLURAC06) (Groom et al. Citation2016). The other two PXRD diffractograms are our original data from samples collected when corresponding SMPS peaks were present. For 1.00 g/L, both the alpha and beta polymorphs are present, as shown by the red and blue peaks, respectively. The 3.33 g/L feedstock PXRD is from a sample we collected when solely the larger beta peak was present on SMPS, and this diffractogram matches the beta polymorph from the GLURAC as simulated by the Cambridge Structural Database (Groom et al. Citation2016).
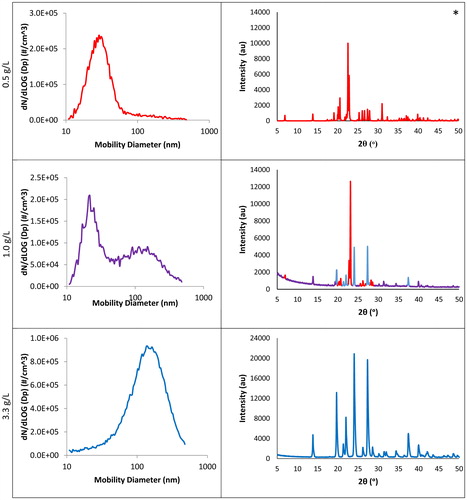
In the particle size distributions shown on the left column of , there is a transition from a dominating smaller diameter peak to a dominating larger diameter peak, in which two peaks are present in between, as the concentration of the glutaric acid solution is increased. Samples were collected from when each of these distinct particle size peaks was present. These samples were run through the PXRD. This allowed us to identify each peak as one of the polymorphs of glutaric acid. We were able to collect and run a sample for 1.00 and 3.33 g/L to obtain a diffractogram from the PXRD. However, the distribution formed under 0.50 g/L was unable to produce purely alpha polymorph data due to the presence of a smaller number of particles but with substantial mass to produce beta peaks as well. Furthermore, the alpha polymorph is only metastable, and some material can revert to the beta phase between collection and analysis. Since we were unable to collect a sufficiently pure sample of the smaller diameter alpha peak, the PXRD diffractogram as simulated for the alpha (GLURAC06) (Groom et al. Citation2016) polymorph from the Cambridge Structural Database is shown in the right column of for 0.50 g/L. We confirmed the presence of purely the beta polymorph in the larger diameter peak by comparing the diffractogram of the 3.33 g/L sample to the PXRD diffractogram as simulated for the beta (GLURAC) (Groom et al. Citation2016) polymorph from the Cambridge Structural Database as shown in .
Figure 3. PXRD diffractograms showing the similarity between the (a) glutaric acid sample collected for this experiment and (b) the beta polymorph of glutaric acid from the Cambridge Structural Database (Groom et al. Citation2016).
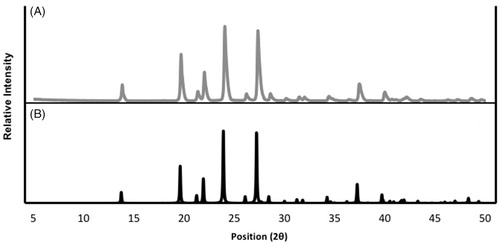
We were able to determine that the larger sized peak, under the concentration of 3.33 g/L, corresponds to the beta polymorph from the PXRD. By eliminating the beta peaks on the 1.00 g/L diffractogram, we identified the remaining peaks to be the alpha polymorph, allowing us to classify the smaller sized peak on the SMPS as the alpha polymorph. The PXRD for 1.00 g/L is clearly a combination of the alpha and beta diffractograms; the peaks are color-coded red and blue, as alpha and beta, respectively, with peaks present in both polymorphs color-coded purple. The PXRD proves that the shifted particle size distribution is not due to water retention, because the particles being measured are both dry and distinct. We therefore successfully identified the peaks and proved that both polymorphs are in fact present under certain conditions. However, it is also imperative that we define under which conditions these peaks are present, such that in further research the polymorphs can be selected accordingly.
To understand when each polymorph is formed, we altered simple variables, while keeping the setup outlined in the methods section constant. With the altering of the concentration of glutaric acid in the aqueous solution and the syringe flow rate, we were able to outline the generation methods for each polymorph as shown in .
Figure 4. SMPS data collected with varying syringe flow rates and concentrations of an aqueous solution of glutaric acid in order to see the variations’ effects on particle size distribution. In this figure, the alpha peak is seen at lower concentrations and flow rates. With increasing glutaric acid concentrations, the alpha peak begins to decrease in size as the beta peak is formed and begins to grow until the alpha peak is no longer observed.
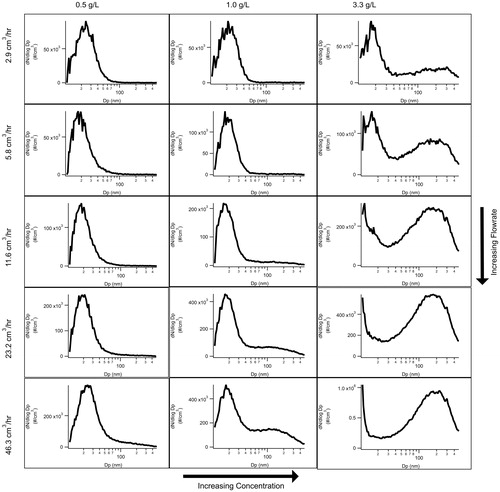
As previously identified in , the two distinct peaks, one at ∼22 nm and the other at ∼170 nm, correspond to the alpha and beta polymorph, respectively. Through varying the concentration of the glutaric acid in the solution as well as the speed of the syringe as shown in , a clear trend becomes apparent among the peaks present for particles against the mobility diameter of the particles. The transition of peaks seen in is most clearly seen at a syringe flow rate of 46.3 cm3/hr, as there is only the alpha peak present at 0.5 g/L, and as the concentration increases to 1.0 g/L the beta peak has appeared. This trend continues through 3.33 g/L, as the alpha peak is non-existent and the beta peak remains. The trend is also seen with increasing syringe flow rates. The increasing tail of the particle mobility size distribution at sizes smaller than 10 nm in mobility diameter is an artifact of the diffusion correction within the TSI Aerosol Instrument Manager software. The trends are consistent both with and without the diffusion correction. The data shown in are just one of three replicate experiments that were conducted by three different operators at three different times separated by several months in order to verify the repeatability of the experiments. Stability of the system is demonstrated in Figure S1 where ammonium sulfate particles are generated under identical conditions to those in . The ammonium sulfate aerosol does not demonstrate the same transitions as the glutaric acid aerosol showing the stability of the aerosol generation system.
Both the increase in concentration of the solution and the syringe flow rate decrease the drying and crystallization rate within the system. When these variables are increased, more glutaric acid is present per particle due to a higher solution concentration (g/L solute) or larger particle concentration (flow rate). When the drying rate increases, there is less time for the glutaric acid to arrange itself into its most stable beta crystallization structure and it instead forms the metastable alpha phase. Alternatively, as the drying rate is decreased, the more stable beta phase is favored. We can conclude that the alpha peak occurs when the crystals are given less time and space to dry and form, as it is present under lower glutaric acid concentration, as well as a lower syringe speed. The exact drying rate is not able to be determined due to how rapidly all of the samples are dried. Literature values for diffusion dryer drying rates usually exceed 85 RH s−1 (Wang et al. Citation2010; Altaf and Freedman Citation2017) meaning that RH measured within a standard diffusion dryer system would not yield accurate results on the scales necessary for crystallization.
Having identified each peak as a distinct polymorph, the conditions under which each peak occurs has allowed us to outline the generation methods of each polymorph from an aqueous solution on a nanoscale. As mentioned in the background section, the formation of these two polymorphs is believed to be a result of heat and mass transfer from the evaporation of the solution and therefore the drying of the particles. Because the initial size distribution leaving the atomizer is identical (as verified by ammonium sulfate control experiments), we assume there to be a differential in shape factor (non-spherical) between the polymorphs. Additionally, it is possible that the different polymorphs are not forming single crystals of glutaric acid but rather more complicated structures with varying degrees of porosity and/or complexity that affect their mobility size. This polymorph-driven difference in particle mobility, therefore, causes two distinct peaks to be present when both polymorphs form from the same solution droplets. Additional evidence for this unusual mobility diameter for glutaric acid is seen in the paper form Mikhailov et al. (Citation2009) where their solutions of constant concentration were prepared and nebulized to produce particles ∼100 nm in mobility diameter from solutions of ammonium sulfate and oxalic acid but particles ∼140 nm in mobility diameter from the same concentration of glutaric acid. Such size differences cannot be attributed to density alone and thus must also involve a shape and/or porosity–structure component. This larger-than-expected size distribution is similar to the larger size mode seen in the data presented here.
4. Conclusions
Through this investigation, we have found that both the alpha and beta polymorph can be formed on the nanoscale from an aqueous solution using a simple atomizer/SMPS setup. These polymorphs, due to their differing shape factor and structure, manifested in two distinctly sized peaks in SMPS data. The correspondence of each peak to a specific polymorph was confirmed with PXRD. By varying many simple variables, the conditions under which the alpha polymorph forms were outlined. The alpha polymorph forms when the crystallization rate is faster, with lower concentrations of glutaric acid and lower flow rates entering the atomizer. These findings are relevant because they could explain the variability associated with the hygroscopicity of glutaric acid in the past. These findings are also important to anyone studying glutaric acid, or other solutions that form polymorphs on the nanoscale, such that they can avoid unwanted variability in their research in the future or at least identify when it may be occurring (by observation of unusually small or large peaks and/or the presence of multiple modes). We recommend running at higher flows and higher concentrations when producing glutaric acid aerosols to ensure that alpha polymorph is not present unless the presence of this polymorph is desired. Even under conditions that produce only the beta polymorph, the mobility size may be affected to have a larger shape or structural differences significantly deviating from spherical.
Author contributions
HT ran initial exploratory experiments. PB designed experiments and carried them out with assistance from HT under the supervision of TR and DD. PB prepared manuscript with contributions from all coauthors.
Supplemental Material
Download Zip (2 MB)Acknowledgments
We’d like to thank Brad Jordan for his help with the X-Ray Diffraction, as well as Ziev Basson and Judy Phan for their previous work.
Disclosure statement
The authors declare that they have no conflict of interest.
References
- PIXE International Corporation. 2018. PIXE Cascade Impactors. http://www.pixeintl.com/impactor.asp.
- Altaf, M. B., and M. A. Freedman. 2017. Effect of drying rate on aerosol particle morphology. J. Phys. Chem. Lett. 8 (15):3613–3618. doi: 10.1021/acs.jpclett.7b01327.
- Bilde, M., K. Barsanti, M. Booth, C. D. Cappa, N. M. Donahue, E. U. Emanuelsson, G. McFiggans, U. K. Krieger, C. Marcolli, D. Topping, et al. 2015. Saturation vapor pressures and transition enthalpies of Low-Volatility organic molecules of atmospheric relevance: From dicarboxylic acids to complex mixtures. Chem. Rev. 115 (10):4115–4156. doi: 10.1021/cr5005502.
- Bilde, M., and B. Svenningsson. 2004. CCN activation of slightly soluble organics: The importance of small amounts of inorganic salt and particle phase. Tellus, Ser. B. 56 (2):128–134. doi: 10.1111/j.1600-0889.2004.00090.x.
- Boucher, O. 2013. Clouds and aerosols. In Climate change 2013: The physical science basis. Contribution of working group I to the fifth assessment report of the intergovernmental panel on climate change, eds. T.F. Stocker, D. Qin, G.-K. Plattner, M. Tignor, S.K. Allen, J. Boschung, A. Nauels, Y. Xia, V. Bex, and P.M. Midgley. Cambridge, United Kingdom: Cambridge University Press.
- Brundle, C. R., C. A. Evans, Jr., and S. Wilson. 1992. Encyclopedia of materials characterization. Stoneham, MA, USA: Butterworth-Heinemann.
- Chan, M. N., and C. K. Chan. 2005. Mass transfer effects in hygroscopic measurements of aerosol particles. Atmospheric Chemistry Physics 5 (10):2703–12.
- Choi, M. Y., and C. K. Chan. 2002. The effects of organic species on the hygroscopic behaviors of inorganic aerosols. Environ. Sci. Technol. 36 (11):2422–28. doi: 10.1021/es0113293.
- Cruz, C. N., and S. N. Pandis. 2000. Deliquescence and hygroscopic growth of mixed inorganic − Organic atmospheric aerosol. Environ. Sci. Technol. 34 (20):4313. doi: 10.1021/es9907109.
- Cruz, C. N., and S. N. Pandis. 1998. The effect of organic coatings on the cloud condensation nuclei activation of inorganic atmospheric aerosol. J. Geophys. Res.: Atmos. 103 (D11):13111–23. doi: 10.1029/98JD00979.
- Ervens, B., G. Feingold, G. J. Frost, and S. M. Kreidenweis. 2004. A modeling study of aqueous production of dicarboxylic acids: 1. Chemical pathways and speciated organic mass production. J. Geophys. Res.: Atmos. 109: D15205. doi: 10.1029/2003JD004387.
- Espeau, P., P. Negrier, and Y. Corvis. 2013. Crystallographic and Pressure-Temperature state diagram approach for the phase behavior and polymorphism study of glutaric acid. Cryst. Growth Des. 13 (2):723–30. doi: 10.1021/cg301442f.
- Goldstein, A. H., D. R. Worton, B. J. Williams, S. V. Hering, N. M. Kreisberg, O. Panić, and T. Górecki. 2008. Thermal desorption comprehensive Two-Dimensional gas chromatography for in-Situ measurements of organic aerosols. J. Chromatogr. A 1186 (1–2):340–7. doi: 10.1016/j.chroma.2007.09.094.
- Groom, C. R., I. J. Bruno, M. P. Lightfoot, and S. C. Ward. 2016. The Cambridge Structural Database. Acta Crystallogr., Sect. B: Struct. Sci., Cryst. Eng. Mater. 72 (2):171–9. doi: 10.1107/S2052520616003954.
- Ha, J.-M., B. D. Hamilton, M. A. Hillmyer, and M. D. Ward. 2009. Phase behavior and polymorphism of organic crystals confined within nanoscale chambers. Cryst. Growth Des. 9 (11):4766–77. doi: 10.1021/cg9006185.
- Hamilton, J. F., A. C. Lewis, J. C. Reynolds, L. J. Carpenter, and A. Lubben. 2006. Investigating the composition of organic aerosol resulting from cyclohexene ozonolysis: Low molecular weight and heterogeneous reaction products. Atmos. Chem. Phys. 6 (12):4973–84. doi: 10.5194/acp-6-4973-2006.
- Hori, M., S. Ohta, N. Murao, and S. Yamagata. 2003. Activation capability of water soluble organic substances as CCN. J. Aerosol. Sci., 34:419–448.
- Kawamura, K., and S. Bikkina. 2016. A review of dicarboxylic acids and related compounds in atmospheric aerosols: Molecular distributions, Sources and transformation. Atmos. Res. 170:140–160. doi: 10.1016/j.atmosres.2015.11.018.
- Knutson, E. O., and K. T. Whitby. 1975. Aerosol classification by electric mobility: Apparatus, theory, and applications. J. Aerosol Sci. 6 (6):443–51. doi: 10.1016/0021-8502(75)90060-9.
- Limbeck, A., and H. Puxbaum. 1999. Organic acids in continental background aerosols. Atmos. Environ. 33 (12):1847–52. doi: 10.1016/S1352-2310(98)00347-1.
- Liu, B. Y., and K. W. Lee. 1975. An aerosol generator of high stability. Am. Ind. Hyg. Assoc. J. 36 (12):861–865. doi: 10.1080/0002889758507357.
- McMurry, P. H. 2000. The history of condensation nucleus counters. Aerosol Sci. Technol. 33 (4):297–322. doi: 10.1080/02786820050121512.
- Mikhailov, E., S. Vlasenko, S. T. Martin, T. Koop, and U. Poschl. 2009. Amorphous and crystalline aerosol particles interacting with water vapor – Part 1: Microstructure, phase transitions, hygroscopic growth and kinetic limitations. Atmos. Chem. Phys. Discuss., 9, 7333–7412.
- Myerson, A. 2002. Handbook of industrial crystallization. Oxford, UK: Butterworth-Heinemann.
- Pant, A., A. Fok, M. T. Parsons, J. Mak, and A. K. Bertram. 2004. Deliquescence and crystallization of ammonium sulfate‐glutaric acid and sodium chloride‐glutaric acid particles. Geophys. Res. Lett. 31:L12111. doi: 10.1029/2004GL020025.
- Parsons, M. T., J. Mak, S. R. Lipetz, and A. K. Bertram. 2004. Deliquescence of malonic, Succinic, Glutaric, and adipic acid particles. J. Geophys. Res.: Atmos. 109:D06212. doi: 10.1029/2003JD004075.
- Peng, C., M. N. Chan, and C. K. Chan. 2001. The hygroscopic properties of dicarboxylic and multifunctional acids: Measurements and UNIFAC predictions. Environ. Sci. Technol. 35 (22):4495–4501. doi: 10.1021/es0107531.
- Phan, J. 2015. Effects of drying rates on size and morphology of nanoparticles. Master’s Thesis, Bucknell University.
- Pope, F. D., B. J. Dennis-Smither, P. T. Griffiths, S. L. Clegg, and A. Cox. 2010. Studies of single aerosol particles containing malonic acid, Glutaric acid, and their mixtures with sodium chloride. I. Hygroscopic growth. J. Phys. Chem. A. 114 (16):5335. doi: 10.1021/jp100059k.
- Pradeep Kumar, P., K. Broekhuizen, and J. P. D. Abbatt. 2003. Organic acids as cloud condensation nuclei: Laboratory studies of highly soluble and insoluble species. Atmos. Chem. Phys. 3 (3):509–20. doi: 10.5194/acp-3-509-2003.
- Saxena, P., and L. M. Hildemann. 1996. Water-Soluble organics in atmospheric particles: A critical review of the literature and application of thermodynamics to identify candidate compounds. J Atmos. Chem. 24 (1):57. doi: 10.1007/BF00053823.
- Seinfeld, J. H., and S. N. Pandis. 2016. Atmospheric chemistry and physics: From air pollution to climate change. Hoboken, NJ: John Wiley & Sons.
- Sullivan, R. C., M. J. K. Moore, M. D. Petters, S. M. Kreidenweis, O. Qafoku, A. Laskin, G. C. Roberts, and K. A. Prather. 2010. Impact of particle generation method on the apparent hygroscopicity of insoluble mineral particles. Aerosol Sci. Technol. 44 (10):830–46. doi: 10.1080/02786826.2010.497514.
- Stolzenburg, M. R., and P. H. McMurry. 1991. An ultrafine aerosol condensation nucleus counter. Aerosol Sci. Technol. 14 (1):48–65. doi: 10.1080/02786829108959470.
- Tavakoli, F., and J. S. Olfert. 2014. Determination of particle mass, Effective density, Mass–mobility exponent, and dynamic shape factor using an aerodynamic aerosol classifier and a differential mobility analyzer in tandem. J. Aerosol. Sci. 75: 35–42. doi: 10.1016/j.jaerosci.2014.04.010.
- Wang, Z., S. M. King, E. Freney, T. Rosenoern, M. L. Smith, Q. Chen, M. Kuwata, E. R. Lewis, U. Pöschl, W. Wang, et al. 2010. The dynamic shape factor of sodium chloride nanoparticles as regulated by drying rate. Aerosol Sci. Technol. 44 (11):939–953. doi: 10.1080/02786826.2010.503204.
- Wise, M. E., J. D. Surratt, D. B. Curtis, J. E. Shilling, and M. A. Tolbert. 2003. Hygroscopic growth of ammonium sulfate/dicarboxylic acids. J. Geophys. Res.: Atmos. 108 (D20):n/a.
- Xue, H., A. F. Khalizov, L. Wang, J. Zheng, and R. Zhang. 2009a. Effects of coating of dicarboxylic acids on the mass − Mobility relationship of soot particles. Environ. Sci. Technol. 43 (8):2787–2792. doi: 10.1021/es803287v.
- Xue, H., A. F. Khalizov, L. Wang, J. Zheng, and R. Zhang. 2009b. Effects of dicarboxylic acid coating on the optical properties of soot. Phys. Chem. Chem. Phys. 11 (36):7869–7875. doi: 10.1039/b904129j.
- Yeung, M. C., T. Y. Ling, and C. K. Chan. 2010. Effects of polymorphic transformation of glutaric acid particles on their deliquescence and hygroscopic properties. J. Phys. Chem. 114 (2):898–903. doi: 10.1021/jp908250v.
- Zardini, A. A., S. Sjogren, C. Marcolli, U. K. Krieger, M. Gysel, E. Weingartner, U. Baltensperger, and T. Peter. 2008. A combined particle trap/HTDMA hygroscopicity study of mixed inorganic/Organic aerosol particles. Atmos. Chem. Phys. 8 (18):5589–5601.