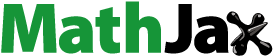
Abstract
Nanomaterials (NMs) are currently treated via recycling, incineration and/or landfilling at their end-of-life. Little is known about the fate of NMs in incineration systems and the efficiency of the available flue-gas cleaning technologies (FGCT) in these systems on the removal of NMs before stack release. In combination with other FGCT such as cyclones, electrostatic precipitator or bag filters, scrubbers participate to limit the release of particulate matter (PM) into the atmosphere. No study has been carried out to investigate wet scrubber collection efficiency regarding nanoparticles under conditions found in a waste incineration plant. In the present study, experimental campaigns were carried out to quantify the performance of a pilot-scale spray scrubber regarding the removal of nanoparticles. The pilot was designed with respect to geometrical, hydrodynamic and residence time scale similitude and operated in gas temperature and humidity conditions representative of full-scale scrubbers in hazardous waste incineration plants. A collection efficiency of 45–62% for a particle size range of 12–90 nm was reported. To evaluate the experimental results, an existing PM collection model based on the 3 main particle collection mechanisms of diffusion, interception and impaction, was adapted for extreme humidity and gas temperature conditions typical of a waste incineration plant. A comparison of the experimental and theoretical results was made indicating that the model results were in good agreement with the experimental results. Contrary to prior studies, the impaction-dominant region occurred at smaller particle sizes (0.1–0.2 µm) corresponding to Stokes number 9 × 10−3 to 4 × 10−2. Numerically, the contribution of the interception mechanism in the collection of nanoparticles (particle sizes 1–100 nm) was found to be negligible (i.e., Interception number 2 × 10−5 to 2 × 10−3).
EDITOR:
1. Introduction
There is a growing concern about the health and environmental effects of ultrafine particles (PM0.1) emissions (Schraufnagel Citation2020; Heinzerling, Hsu, and Yip Citation2016). Inhalable ambient particles consisting of nanoparticles (NPs) with a size range from 1 to 100 nm (Khan, Saeed, and Khan Citation2019) have been associated with cardiovascular and respiratory illnesses (Calderón-Garcidueñas et al. Citation2019a; Thomas, Al Mutairi, and De Citation2013; WHO Citation2006). The release of NPs into the atmosphere have been linked to dust cloud formation, decrease in sun light intensity and ozone depletion (Kabir et al. Citation2018; Smita et al. Citation2012). Several studies have shown that the uptake of NPs by microorganisms and plants could lead to DNA damage, reactive oxygen species production and accumulation in the edible part of plants (Mazari et al. Citation2021; Sidiropoulou et al. Citation2018; Vittori Antisari et al. Citation2018). The unique properties of NPs such as size, shape and high surface area that attract their interest in industrial applications could also affect their toxicity (Kang et al. Citation2011). Indeed, nano-sized or nanostructured particles are more toxic than their corresponding micronic-size particles of the same chemical surface properties due to the former’s increased surface area, substantial adsorption efficiency, better optical properties and increased chemical reactivity (Calderón-Garcidueñas, Reynoso-Robles, and González-Maciel Citation2019b; Teleanu et al. Citation2018; Faisal and Kumar Citation2017; Nurkiewicz et al. Citation2008; Oberdörster, Oberdörster, and Oberdörster Citation2005). This becomes an area of great concern when one considers the recent surge in the manufacturing and use of engineered nanomaterials (NMs) (Part et al. Citation2018). According to the Commission Recommendation of the EU, a nanomaterial is “a natural, incidental or manufactured material containing particles, in an unbound state or as an aggregate or as an agglomerate and where, for 50% or more of the particles in the number size distribution, one or more external dimensions is in the size range 1 nm–100 nm”(EC, Citation2010).
The global NMs market is projected to grow at a compound annual growth rate (CAGR) of 13.1% from 2020 to 2027 from its 2019 value of USD 8.5 billion (Grand View Research Citation2020). For the EU, the NMs Market is projected to reach more than $9 billion by 2022, growing with a CAGR of 20.0% during 2016–2022 (Allied Market Research Citation2016). This rapid growth in the NM market has resultantly attracted increasing concerns from all stakeholders with regards to the management of NM at their end-of-life (Mishra, Arya, and Panchal Citation2020; Campos and López Citation2019; Part et al. Citation2018; Faunce Citation2017).
To date, there are no global/EU standards on the management of nanowaste. Thus, waste containing NMs are treated like any other waste, i.e., via recycling, incineration and/or landfilling without specific requirements. Research by Part et al. (Citation2018) on the fate of NMs in commonly used waste management processes such as composting, recycling, incineration and landfilling revealed that a substantial knowledge gap exists. The authors concluded that incineration appeared to be the least investigated management process for NMs waste. Most of these studies occurred at laboratory-scale (Cernuschi et al. Citation2019; Ounoughene et al. Citation2015, Citation2019; Pourchez et al. Citation2018; Baumann et al. Citation2017; Buonanno and Morawska Citation2015; Massari et al. Citation2014; Buha et al. Citation2014; Vejerano, Holder, and Marr Citation2013, Vejerano et al. Citation2014; Derrough et al. Citation2013; Cernuschi et al. Citation2012; Mueller et al. Citation2012; Buonanno, Ficco, and Stabile Citation2009), making some of their conclusions somewhat study specific as the full complexity involved in waste incineration was not taken into account. For the large-scale studies (Oischinger et al. Citation2019; Baran and Quicker Citation2017; Börner et al. Citation2016; Lang et al. Citation2015; Walser et al. Citation2012), the majority of the NMs ended up in the bottom ash while some fractions of the NMs were detected in the fly ash. These conclusions were also partly supported by some of the lab-scale studies.
The resulting PM size distribution after incineration is influenced by parameters such as the nature of the specific NM, the combustion temperature (850–1100 °C), the retention time, the oxygen rate, the gas buoyancy and the temperature gradient at the outlet of the furnace (Lang et al. Citation2015; Mueller et al. Citation2012, Citation2013).
To limit the release of PM into the environment from industrial processes such as waste incineration, wet scrubbers are often combined with dry scrubbing and further flue-gas cleaning technologies (FGCT) such as cyclones, fabric filters and electrostatic precipitators (Neuwahl et al. Citation2019).
Scrubbers are added to the FGCT primarily to treat acid gases but are also capable of handling (flammable and explosive) PM (Vallero Citation2019). In wet scrubbers, the absorption of acid gases is provided inside the scrubbers by pure water or a mixture of water and neutralizing additives, while PM are captured by the droplets. Spray wet scrubbers (Keshavarz et al. Citation2008) are amongst the most common types of scrubbers; others include: Packed bed wet scrubbers (Bhave, Vyas, and Patel Citation2008), Tray wet scrubbers (Schifftner Citation2013), Venturi wet scrubbers (Ali et al. Citation2013) and Gravitational wet scrubbers (Kim et al. Citation2001).
Particle scavenging in scrubbers is governed by several mechanisms such as Brownian diffusion, interception, impaction, thermophoresis, diffusiophoresis, centrifugal forces, condensation and electrostatic attraction. These collection mechanisms are highly dependent on the particle size distribution in the flue-gas stream. Usually, one mechanism becomes dominant for a given particle size range and acts simultaneously with other mechanisms to give a minimum collection efficiency for that particle size distribution. Contrary to wide held beliefs that conventional scrubbers are ineffective for PM < 1.0 µm, Kim et al. (Citation2001) has shown that under favorable operating conditions PM much less than 1.0 µm can be effectively collected. presents literature studies on the effect of operating parameters such as particle size, droplet diameters, gas temperature, gas velocity, and L/G ratio on wet scrubber performance. Only one experimental study (Vasudevan, Gokhale, and Mahalingam Citation1985) was identified with operating conditions close to those encountered in waste incineration regarding the droplet size and inlet gas temperature (resp. 70 μm and 200 °C). No experimental study was identified regarding nanoparticles collection.
Table 1. Overview of experimental and theoretical studies about particle removal by wet scrubber.
In this study, we seek to bridge this knowledge gap by investigating the removal of nanoparticles by a pilot-scale spray scrubber designed with respect to geometrical, hydrodynamic and residence time scale similitude, and operated under realistic conditions in terms of inlet and outlet gas temperature and humidity representative of a full-scale hazardous waste incineration spray scrubber. This study is equally relevant to waste-to-energy processes such as municipal solid waste incineration where NMs form part of the waste mix and a wet scrubbing system is present in the flue gas cleaning line. Lastly, the experimental results are then compared to a theoretical model involving particle collection mechanisms. An investigation of the contribution of the individual particle collection mechanisms is also made.
2. Materials and methods
2.1. Experimental set-up
The principal component of the experimental set-up () is the spray scrubbing tower: the height is 1.9 m (excluding the mist collector), the diameter is 0.3 m. Within the tower, spray headers are located at four different stages with an adjustable number of nozzles; the configuration studied was 3-4-7-7 nozzles for headers 1 (top) to 4 (bottom) corresponding to a total liquid flow of 3.2 L.min−1. The nozzles are single-orifice with a diameter of 0.45 mm and average droplet diameter close to 75 µm (the Sauter mean diameter and the median diameters are 63 µm and 75 µm respectively) according to the manufacturer. The scrubbing tower was designed to be in scale similitude with full-scale towers encountered in the flue-gas treatment line of waste incineration plant (after ESP), in terms of inlet and outlet gas temperatures and humidity, liquid to gas flow rate ratio, ratio between height and diameter of the column, residence time of the gas, turbulent flow regime and droplet diameter.
To operate the set-up, air from the laboratory is supplied to the set-up by a centrifugal fan (located downstream of the scrubber) after being previously filtered by a G4 and F9 filters (EN779:2012). An anemometer is used to measure the velocity flow. The airflow is conditioned in a straight length in two steps. Initially, the airflow is heated to 70 °C and then moistened by steam injection of about 9 kg.h−1. Next, the airflow is further heated to 200 °C and followed by particles injection. The tests were performed with carbon NPs generated by a DNP 2000 (Palas) spark generator. The DNP 2000 generates carbon particles in the size range of 10–100 nm by spark discharge between two graphite electrodes. To evade the oxidation of the carbon, a nitrogen stream at 6 L.min−1 was supplied. The carbon, evaporated in the spark, was transported by the nitrogen flow through the space between the electrodes and condenses to very fine primary particles. Depending on their concentrations, these particles coagulate to big agglomerates. A particle mass flow of 6.5 mg.h−1 was generated by setting the spark frequency to 200 s−1. Agglomerates were reduced by means of an exact dilution of the aerosol with clean pressured air with a volume flow of 33 L.min−1.
Particle counting was performed a meter away from the generation, upstream of the spray scrubber, using a scanning mobility particle sizer (SMPS, Grimm). The scanning mobility particle sizer (SMPS) consisting of a long differential mobility analyzer (DMA) and a condenser particle counter (CPC) was employed to measure particle size distribution base on real-time selective (mobility-equivalent diameter) number concentration. The 45 particle classifications channels were used. This allowed for the possible detection of particle size range from 10–1000 nm at a sampling flow of 0.3 L.min−1 requiring about four minutes for each measurement. The estimated particle concentration prior to scrubbing was 1.2 × 106 #.cm−3. To simplify the complex matrix of pollutants encountered in waste incineration, only NPs were generated and injected in this study.
The gas flow then made its way into the scrubber via the bottom. Water at 60 °C was used as spraying liquid. The water temperature was set to achieve the target gas outlet temperature of 70 °C. The resulting purge water was collected at the bottom in a tank, filtered and recycled back into the scrubber. At the top of the scrubber, particle counting was ensured after a mist collector. The gas flow was condensed; the recovered water is recirculated into the water tank while the dry airflow is filtered by an H14 filter before final release to the atmosphere. To avoid the adverse effect of droplets on the particle counting by the SMPS, both upstream and downstream sampling lines were heated to 150 °C. Thus, the high relative humidity (∽100%) of the gas downstream of the scrubber was reduced to 6% while the relative humidity of the gas upstream of the scrubber increased from 1% to 5%. A two-stage dilution (100x dilution factor) was then performed to lower the particle concentration, the gas temperature and humidity prior to counting with the SMPS: the first diluter was heated to 150 °C while the second diluter was operated at room temperature. In this way, stable and repeatable particle concentration and size distribution results were measured as the effects of condensation and nucleation were eliminated.
2.2. Modeling of particle collection by scrubber
The particle removal efficiency is expressed in several ways including the efficiency of a single water droplet, the efficiency of the scrubber on a mass basis, or the efficiency of the scrubber on a particle size basis. Usually, an overall efficiency of particle collection is considered. This overall efficiency considers the contributions due to the different particle capture mechanisms. In this study, we assumed that the overall collection efficiency consists of only the contributions of the three principal collection mechanisms of impaction, Brownian diffusion and interception
For particles having larger than 5 µm diameter and/or transported by gas stream velocity greater than 0.3 m.s−1 (Kim et al. Citation2001; Perry, Green, and Maloney Citation1997), impaction is the dominant collection mechanism. The impaction mechanism occurs when the particles possess sufficient inertia to maintain their trajectory leaving their initial gas stream and crash with the droplet collector on their path.
Brownian diffusion is the primary mechanism responsible for collecting fine particles from a gas stream as a result of irregular motion along the gas streamline transporting the PM caused by the random collisions of the particles with gas molecules. Due to their negligible masses, the fine particles undergo diffusion movement and are captured by the liquid droplets. According to Yalamov, Vasiljeva, and Schukin (Citation1977), the collection by Brownian diffusion mechanism occurs for particle sizes lower than 100 nm. Numerous authors (Zhao and Zheng Citation2008; Schnelle and Brown Citation2002; Pilat and Prem Citation1976; Johnstone and Roberts Citation1949) have observed that Brownian diffusion is one of the main particle collection mechanisms in wet scrubbers.
Collection by interception occurs when a particle follows a gas streamline that is within one particle radius of the surface of the liquid droplet, it is intercepted by the liquid droplet. Interception is considered as one of the main mechanisms responsible for particle collection by water droplets in wet scrubbers for particles larger than 0.5 (Keshavarz et al. Citation2008; Schnelle and Brown Citation2002; Kim et al. Citation2001; Jung and Lee Citation1998; Gemci and Ebert Citation1992).
2.2.1. Collection efficiency of a single droplet, 

2.2.1.1. Impaction, 

To characterize the particles captured by the impaction mechanism, Stokes number () is the dimensionless parameter that translates the impaction effect and is defined as the ratio between the stopping distance of the particle and the characteristic length of the obstacle, in other words, it is the ratio of drag-to-viscous forces. The efficiency of particle removal from the gas streamlines increases as the value of the Stokes number increases. The Stokes number is defined by the following equation:
(1)
(1)
Where
is the particle density kg.m−3,
the particle diameter (m), D the droplet diameter (m), µ the viscosity of gas (Pa.s) and U the relative velocity between particles and liquid droplets (m.s−1).
A mathematical correlation for the single droplet removal efficiency due to impaction was developed by Licht (Citation1988) as:
(2)
(2)
Both Seinfeld and Pandis (Citation2006) and Kim et al. (Citation2001) argued that when the size distribution of flue gas particles is represented by a log-normal distribution function, EquationEquation (2)(2)
(2) would not be suitable. The following EquationEquations (3a)
(3a)
(3a) and Equation(3b)
(3b)
(3b) were proposed by Kim et al. (Citation2001) as an alternative to EquationEquation (2)
(2)
(2) .
(3a)
(3a)
(3b)
(3b)
The EquationEquations (4a)(4a)
(4a) , Equation(4b)
(4b)
(4b) , and Equation(4c)
(4c)
(4c) below were developed by Lim, Lee, and Park (Citation2006):
(4a)
(4a)
(4b)
(4b)
(4c)
(4c)
2.2.1.2. Brownian diffusion, 

Peclet number is the dimensionless parameter used to describe the diffusion mechanism and is defined as:
(5)
(5)
is the diffusion coefficient of particle and is defined as:
(6)
(6)
Where T is the gas absolute temperature,
is the Boltzmann constant and
the Cunningham slip correction factor.
Jung and Lee (Citation1998) developed the following expression for the diffusion collection efficiency of a single liquid droplet as:
(7)
(7)
Where α is the solid volume fraction, σ the viscosity ratio of liquid to gas, J and K are hydrodynamic factors.
The Cunningham slip correction factor used is based on the Knudsen – Weber equation and was estimated from:
(8)
(8)
Where
is the gas molecules mean free path length; a, b, c = 2.492, 0.84 and 0.435 respectively (Fuchs Citation1964).
2.2.1.3. Interception, 

The interception parameter R, is defined as the ratio of particle diameter to the liquid droplet diameter:
(9)
(9)
R is much less than one when the droplet diameter is larger than the particle diameter. Jung and Lee (Jung and Lee Citation1998) defined the single droplet efficiency by interception:
(10)
(10)
2.2.2. Overall collection efficiency, 

The overall collection efficiency is expressed as follows (Raj Mohan, Biswas, and Meikap Citation2008):
(11)
(11)
Where
is the liquid flow rate (m3.s−1),
is the gas flow rate (m3.s−1),
the terminal settling velocity of droplets (m.s−1),
the gas velocity in the tower (m.s−1),
the height of the tower (m).
Bearing in mind the independence of the contribution of the various particle collection mechanisms, the collection efficiency of a single droplet is given as (Wu et al. Citation2019):
(12)
(12)
3. Results and discussion
3.1. Determination of particle effective density
The elementary density of carbon is 2000 kg.m−3, however, to account for the presence of voids due to the internal structure of the particles, humidity conditions and the non-spherical nature of the carbon NPs generated by the spark generator, an estimation of the particle “effective density” was carried out. As stated by Ristimäki et al. (Citation2002), particle effective density is not a parameter that is determined directly; it can be found if one of the following combinations is known: mobility size – aerodynamic size, mobility size – particle mass, or aerodynamic size – particle mass. Recently, a combination of particle measurements by an aerosol particle mass analyzer (APM) and a differential mobility analyzer (DMA) has been widely employed (Yin et al. Citation2015; Nakao et al. Citation2013) to determine the particle effective density.
In this study, we measured the particle size distribution (PSD) of the carbon NPs expressed with aerodynamic and electrical mobility diameters using an electrical low-pressure impactor (ELPI, Dekati) and a Scanning Mobility Particle Spectrometers (SMPS, Grimm) respectively. The geometric mean particle diameter was 28.5 nm with a geometric standard deviation of 1.5.
DeCarlo et al. (Citation2004) reported that, aerosol effective density () can be determined by simultaneously measuring the electrical mobility diameter (
) and the aerodynamic equivalent diameter (
) as shown below:
(13)
(13)
Where
is the slip correction factor as earlier stated and
is the reference density (1000 kg.m−3). We used the median diameter (D50) from the SMPS PSD with a value of 26.61 nm as dm and the D50 from the ELPI PSD with a value of 33.38 nm as da (). The effective density was found to be 1279 kg.m−3. The shape of carbon NPs are usually non-spherical chain-like aggregates (Lee Citation2008). However, after generation with the PALAS generator, they become loose agglomerate. Hence their effective densities decrease as the particle sizes increase (Park et al. Citation2003).
3.2. Particle fractional collection efficiency according to particle aerodynamic diameter
We investigated the NPs removal efficiency of the pilot-scale scrubber at typical conditions encountered in a waste incineration plant, i.e., a gas inlet temperature of 200 °C, liquid flow of 3.3 L.min−1, vapor flow of ∼ 9 kg.h−1; the gas flow rate was 34 Nm3.h−1. illustrates the collection efficiency versus aerodynamic particle diameter. Due to the low resolution of ELPI in nanoparticle size classifications (Maricq, Xu, and Chase Citation2007), the particle size measurements were initially performed by the SMPS and the results expressed in electrical mobility diameters. Using the calculated particle effective density and EquationEquation (13)(13)
(13) , the PSD expressed in particle mobility diameters were converted to aerodynamic diameters. The results showed a U-shaped curve for the particle size range under study (i.e., 12–90 nm) with a minimum collection efficiency of 45% at particle diameter (dp) of 35 nm. As the dp increases from 35 nm to 90 nm, a gradual increase in the collection efficiency was observed, reaching a maximum value of 62% at dp of 90 nm. Larger particles possess more inertia and hence can persist in their state of motion until they cross the fluid streamlines of the droplets. Likewise, as dp decreases from 35 nm to 12 nm, the removal of the NPs by the scrubber increases, attaining a maximum value of 61% at dp of 12 nm. When the diameter of the particles became smaller, they experienced more random motions leading to their improved scrubbing by the droplets due to the diffusion mechanism. As stated, the minimum collection efficiency occurred at dp of 35 nm. Similar outcomes at varying operational conditions were obtained by other authors (Di Natale et al. Citation2015; Lee et al. Citation2013; Pranesha and Kamra Citation1996). For Lai et al. (Citation1978) the minimum collection efficiency occurred at dp of 0.6 µm. Wang and Pruppacher (Citation1980) noticed the same sudden decrease in collection efficiency for particles of diameter range 2 to 4 µm. Irrespective of the size range, the most penetrating particle size (MPPS) region occurs because neither of the three principal mechanisms is dominant in this region.
Figure 3. Experimental collection efficiency of the carbon nanoparticles by the spraying scrubber (average diameter N = 3; range).
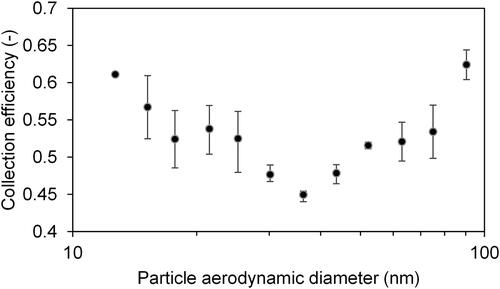
A point to consider is the hygroscopic characteristics of the carbon NPs and how this could affect the spray scrubber collection efficiency. Commodo et al. (Citation2016) investigated the hygroscopic properties of organic carbon NPs and soot NPs formed in premixed flames at different carbon to oxygen (C/O) ratios and residence time. Results from static contact angle measurements by the authors revealed that the organic carbon NPs and the soot NPs from the C/O ratio of 0.67 flame were highly hydrophobic. However, the organic carbon NPs from the C/O ratio of 0.63 flame was found to be hydrophilic. The authors explained that the variation in the hygroscopic properties of the organic carbon NPs was due to the amount of surface oxygen as shown by an X-ray photoelectron spectroscopy. This surface oxygen was 6% and 3% for C/O of 0.63 and 0.67 respectively.
The carbon NPs used in the present study were generated from graphite spark discharge (i.e., graphite sublimation/aggregation at ambient temperature), as such, the C/O ratio is high. We therefore estimate that the carbon nanoparticles have low oxygen surface function and so are rather hydrophobic.
There is currently no study on the potential effect of the hygroscopic behavior of carbon NPs on the efficiency of a spray scrubber under complex conditions such as those encountered in waste incineration plants. However, the influence of relative humidity on a venturi scrubber particle collection efficiency was investigated by Calvert, Lundgren, and Mehta (Citation1972). Three monodisperse particles of size 0.75 µm; pure uranine particles (PU), mixture of uranine and boric acid particles (B&PU), and Methylene blue particles (MB) were used in Calvert, Lundgren, and Mehta (Citation1972) study. The authors observed that the PU particles grew significantly as they travel through the scrubber. Remarkably, a significant number of smaller PU particles were also observed downstream of the scrubber than those introduced upstream. Further investigations under microscope revealed that the B&PU particles also grew when exposed to varying relative humidity. However, the B&PU particles regained their initial sizes as conditions were reversed with dry air. The MB particles did not undergo size changes with changes in the relative humidity. At 0.75 µm particles, Calvert, Lundgren, and Mehta (Citation1972) reported that the collection efficiency of MB was lowered than for both pure PU and B&PU particles. Calvert, Lundgren, and Mehta (Citation1972) concluded that this was a result of hydrophilic particles (PU and B&PU) undergoing rapid growth as the gas contacts and atomizes the liquid in the venture throat.
Calvert, Lundgren, and Mehta (Citation1972) conclusions that the venturi scrubber collection efficiency of hydrophilic particles (pure uranine particles, and a mixture of uranine and boric acid particles) is higher than for hydrophobic particles (Methylene blue particles) will have to be investigated in future works for the collection of nanoparticles by spray scrubbers under waste incineration conditions.
3.3. Particle collection efficiency modeling
3.3.1. Model parameters
An existing mathematical model based on impaction, Brownian diffusion and interception phenomena was valorized for extreme humidity and gas temperature conditions typical of a hazardous waste incineration plant and the result compared with the experimental results. The single droplet contributions due to impaction, Brownian diffusion and interception mechanisms were estimated from EquationEquations (4a)–(4c), (7)(7)
(7) , and Equation(10)
(10)
(10) , respectively. For dp of 1–100 nm and the average droplet diameter of 75 µm, the range of the dimensionless parameters of the mechanisms considered in the model are given below:
Stokes number (
), 7 × 10−7 to 7 × 10−3
Peclet number (
), 2 × 102 to 1 × 106
Interception number (
), 1 × 10−5 to 1 × 10−3
EquationEquation (11)(11)
(11) was used to calculate the overall particle collection efficiency by the spray scrubber.
In we present the parameters used for the model calculations. We calculated the droplet velocity using the continuity equation from the values of the liquid flow (3.2 L.min−1) and internal diameter (0.45 mm) of the nozzles. The packing density or solid volume fraction was determined as the ratio of the liquid volume to the tower volume. Calculating the liquid volume as the product of the liquid flowrate and the (residence) time taken for the droplet to travel from the midpoint of the tower to the base of the tower. The terminal settling velocity of droplets was calculated from the three forces acting on the droplets: drag, buoyancy, gravity forces, with the assumption that the droplets are spherical and did not undergo any form of deformation.
Table 2. Operating parameters used for the model calculations.
3.3.2. Sensitivity analysis of the droplet diameter parameter
The sensitivity of a model parameter, the droplet diameter, was studied. This parameter was studied because (i) it was not measured in the set-up (ii) a mean value was set in the model instead of size distribution. compares the experimental results with the results of the model at the calculated particle effective density of 1279 kg.m−3 and for different droplet diameters. also demonstrates the effect of the droplet diameter (D) on the particle collection efficiency. We observe a U-shaped curve with the minimum collection efficiencies occurring at particle diameter of 40 nm and with the values of 21% for D = 100 µm, 33% for D = 75 µm, 45% for D = 60 µm and 56% at D = 50 µm respectively. Overall, as the droplet diameter decreased, the collection efficiency increased for the entire range of particle sizes under study. This outcome is in agreement with previous studies and can be explained by the fact that the particle-droplet contact area increases as the droplet diameter decreases.
The model results considering the average droplet diameter of 60 µm are in good agreement with the experimental results for the particle size range studied. The better agreement at the 60 µm droplet diameter than at the value given by the nozzle manufacturer (75 µm) may be explained by the physical phenomenon encountered in the scrubber. As the tower gas inlet temperature is 200 °C and its exit gas temperature is 70 °C, this will lead to a temperature gradient in the tower with certain regions with temperatures that are slightly higher than the boiling point of the droplets. This will result in the vaporization of the droplet surfaces, decreasing their sizes from 75 µm at the exit of the nozzles to smaller diameters. This evaporation rate is moderate and its controlling parameter is the vapor diffusion rate. This is in line with studies by Aguilar et al. (Citation2001) and Xiong and Sun (Citation2017). We postulate that, certain regions in the tower with lower temperature (lower than 70 °C) and high humidity rate could lead to droplet condensation on preexisting droplets and/or particles. This evaporation/condensation phenomena could lead to a local thermal hot/cold point with reference to the gas temperature. This could also contribute to thermophoresis effects which we did not considered in the collection efficiency model.
3.3.3. Contributions of the various particle collection mechanisms
A knowledge gap observed by Kim et al. (Citation2001), was the lack of experimental test to validate the numerous theoretical studies carried out on the PM collection by wet scrubbing. As a result, prior studies have come to the conclusion that the impaction mechanism is the dominant particle removal mechanism for PM greater than 5.0 µm while Brownian diffusion is believed to be dominant for “small” PM (Lim, Lee, and Park Citation2006; Kim et al. Citation2001; Gemci and Ebert Citation1992; Yalamov, Vasiljeva, and Schukin Citation1977). As represented in our experimental results (), the impaction-dominant region can occur at much smaller PM range. This outcome is reinforced in , where we present the theoretical contribution of the individual particle collection mechanisms considered in this study. At dp of 0.1 µm and 0.2 µm, the collection efficiency due to impaction alone were 80.9% and 88.7% respectively. At the same dp, the contributions due to diffusion and interception were only 16.3% and 6.8%, and 2.8% and 4.4% respectively. Therefore, a better approach to determine if a mechanism is dominant or not, that accounts for the operating conditions (not only the particle sizes) of the scrubber is to use the dimensionless parameter that represent that mechanism (EquationEquations (1), (5)(5)
(5) , and Equation(9)
(9)
(9) ). For the PM range studied (1–100 nm) and at the fitted droplet diameter 60 µm, the Stokes (
Peclet (
) and Interception (
) numbers were 9 × 10−7 to 9 × 10−3, 1 × 102 to 1 × 106, and 2 × 10−5 to 2 × 10−3 respectively. Impaction was found to be dominant for
3 × 10−3 to 9 × 10−3 at dp 60–100 nm, diffusion was dominant for Pe 1 × 102 to 8 × 104 at dp 1–25 nm, while the contribution due to interception mechanism was negligible. Future investigations have to evaluate the lower/upper limits of the dimensionless parameters.
4. Conclusions
In this study, an investigation of the removal of nanoparticles by a pilot-scale spray scrubber designed with respect to geometrical, hydrodynamic and residence time scale similitude and operated in inlet and out gas temperatures and humidity conditions representative of a full-scale scrubber in hazardous waste incineration plant was carried out. The experimental results were compared to an adapted PM collection model.
Spray scrubber participate in limiting the release of nanoparticles from industrial processes such as waste incineration plants as we report a collection efficiency of 45–62% for particle size range of 12–90 nm in aerodynamic diameter, with a minimum collection efficiency of 45% at particle size of 35 nm.
The results of the model at four varying droplets sizes 50, 60, 75 and 100 µm showed that the nanoparticle collection efficiency improved as the droplet sizes decreased. The model results at average droplet sizes of 60 µm are in good agreement with the experimental results.
Contrary to prior studies that the impaction-dominant region occurs at PM > 5.0 µm, under favorable operating conditions, the impaction-dominant region can occur at much smaller PM sizes as is the case in the present study where nanoparticles collection due to impaction mechanism alone was 80.9% and 88.7% at PM sizes of 0.1 µm and 0.2 µm respectively. We therefore propose that the dimensionless parameters that represents the mechanisms, Stokes number in the case of impaction, Peclet number for Brownian diffusion and Interception number for the interception mechanism be used as the variables to consider if a mechanism is dominant or not. Future investigations have to evaluate the lower/upper limits of these dimensionless parameters.
Under the operating conditions typical of a spray scrubber in a waste incineration plant, the contribution due to the interception mechanism in the collection of nanoparticles was found to be negligible (Interception number 2 × 10−5 to 2 × 10−3 corresponding to particle size 1–100 nm). Hence, the collection of nanoparticles by the spray scrubber is dominated by impaction and Brownian diffusion mechanisms.
Declaration of conflicting interests
The authors declare that they have no conflict of interest.
Additional information
Funding
References
- Achiles, A., and V. Guerra. 2020. Performance of a cyclone scrubber in removal of fine particulate matter. CI&CEQ. 26 (1):31–40. doi:10.2298/CICEQ181220022A.
- Aguilar, G., B. Majaron, W. Verkruysse, Y. Zhou, J. S. Nelson, and E. Lavernia. 2001. Theoretical and experimental analysis of droplet diameter, temperature, and evaporation rate evolution in cryogenic sprays. Int. J. Heat Mass Transf. 44 (17):3201–11. doi:10.1016/S0017-9310(00)00363-X.
- Ahmed, S., H. Mohsin, K. Qureshi, A. Shah, W. Siddique, K. Waheed, N. Irfan, M. Ahmad, and A. Farooq. 2018. Investigation of dust particle removal efficiency of self-priming venturi scrubber using computational fluid dynamics. Nucl. Eng. Technol. 50 (5):665–72. doi:10.1016/j.net.2018.01.016.
- Ali, M., C. Yan, Z. Sun, H. Gu, and K. Mehboob. 2013. Dust particle removal efficiency of a venturi scrubber. Ann. Nucl. Energy. 54:178–83. doi:10.1016/j.anucene.2012.11.005.
- Allied Market Research. 2016. Europe Nanomaterials Market to Reach $9,078 Million, Globally, By 2022. Accessed December 14, 2020. //www.alliedmarketresearch.com/press-release/europe-nanomaterials-market.html.
- Attaullah, N. A., M. B. K. Niazi, M. Ahsan, and M. Ali. 2020. Computational fluid dynamics simulation for the prediction of the venturi scrubber performance using finite volume method. IJCSM. 11 (4):338–46. doi:10.1504/IJCSM.2020.107601.
- Bal, M., H. Siddiqi, S. Mukherjee, and B. C. Meikap. 2019. Design of self priming venturi scrubber for the simultaneous abatement of HCl gas and particulate matter from the flue gas. Chem. Eng. Res. Des. 150:311–9. doi:10.1016/j.cherd.2019.08.005.
- Bandyopadhyay, A., and M. N. Biswas. 2007. Fly ash scrubbing in a novel dual flow scrubber. Waste Manag. 27 (12):1845–59. doi:10.1016/j.wasman.2006.10.013.
- Baran, P., and P. Quicker. 2017. Fate and behavior of nanoparticles in waste incineration. Österr. Wasser- Und Abfallw. 69 (1–2):51–65. doi:10.1007/s00506-016-0362-z.
- Baumann, W., N. Teuscher, M. Hauser, J. Gehrmann, H. R. Paur, and D. Stapf. 2017. Behaviour of engineered nanoparticles in a lab-scale flame and combustion chamber. Energy Procedia. 120:705–12. doi:10.1016/j.egypro.2017.07.194.
- Bhave, A. G., D. K. Vyas, and J. B. Patel. 2008. A wet packed bed scrubber-based producer gas cooling-cleaning system. Renew. Energy 33 (7):1716–20. doi:10.1016/j.renene.2007.08.014.
- Bianchini, A., F. Cento, L. Golfera, M. Pellegrini, and C. Saccani. 2016. Performance analysis of different scrubber systems for removal of particulate emissions from a small size biomass boiler. Biomass Bioenerg. 92:31–9. doi:10.1016/j.biombioe.2016.06.005.
- Bianchini, A., M. Pellegrini, J. Rossi, and C. Saccani. 2018. Theoretical model and preliminary design of an innovative wet scrubber for the separation of fine particulate matter produced by biomass combustion in small size boilers. Biomass Bioenerg. 116:60–71. doi:10.1016/j.biombioe.2018.05.011.
- Biswas, S., B. Rajmohan, and B. C. Meikap. 2008. Hydrodynamics characterization of a counter-current spray column for particulate scrubbing from flue gases. Asia-Pacific J. Chem. Eng. 3 (5):544–9. doi:10.1002/apj.177.
- Börner, R., M. Meiller, J. Oischinger, and R. Daschner. 2016. Untersuchung möglicher Umweltauswirkungen bei der Entsorgung nanomaterialhaltiger Abfälle in Abfallbe-handlungsanlagen.
- Buha, J., N. Mueller, B. Nowack, A. Ulrich, S. Losert, and J. Wang. 2014. Physical and chemical characterization of fly ashes from Swiss waste incineration plants and determination of the ash fraction in the nanometer range. Environ. Sci. Technol. 48 (9):4765–73. doi:10.1021/es4047582.
- Buonanno, G., G. Ficco, and L. Stabile. 2009. Size distribution and number concentration of particles at the stack of a municipal waste incinerator. Waste Manag. 29 (2):749–55. doi:10.1016/j.wasman.2008.06.029.
- Buonanno, G., and L. Morawska. 2015. Ultrafine particle emission of waste incinerators and comparison to the exposure of urban citizens. Waste Manag. 37:75–81. doi:10.1016/j.wasman.2014.03.008.
- Calderón-Garcidueñas, L., A. González-Maciel, P. S. Mukherjee, R. Reynoso-Robles, B. Pérez-Guillé, C. Gayosso-Chávez, R. Torres-Jardón, J. V. Cross, I. A. M. Ahmed, V. V. Karloukovski, et al. 2019a. Combustion- and friction-derived magnetic air pollution nanoparticles in human hearts. Environ. Res. 176:108567. doi:10.1016/j.envres.2019.108567.
- Calderón-Garcidueñas, L., R. Reynoso-Robles, and A. González-Maciel. 2019b. Combustion and friction-derived nanoparticles and industrial-sourced nanoparticles: The culprit of Alzheimer and Parkinson’s diseases. Environ. Res. 176. doi:10.1016/j.envres.2019.108574.
- Calvert, S., D. Lundgren, and D. S. Mehta. 1972. Venturi scrubber performance. J. Air Pollut. Control Assoc. 22 (7):529–32. doi:10.1080/00022470.1972.10469674.
- Campos, A., and I. López. 2019. Current status and perspectives in nanowaste management. In Handbook of environmental materials management, ed. C. M. Hussain, 2287–314. Springer International Publishing.
- Carotenuto, C., F. Di Natale, and A. Lancia. 2010. Wet electrostatic scrubbers for the abatement of submicronic particulate. Chem. Eng. J. 165 (1):35–45. doi:10.1016/j.cej.2010.08.049.
- Centner, P., H. Büttner, and F. Ebert. 1989. Investigation of a wet dust scrubber with a pneumatic nozzle: Dust collection based on turbulent diffusion. Chem. Eng. Technol. 12 (1):439–44. doi:10.1002/ceat.270120163.
- Cernuschi, S., G. Lonati, S. Ozgen, R. Tardivo, and S. Signorini. 2019. Ultrafine and nanoparticles emissions from clinical waste incineration: characterization and chemical speciation. In 16th International Conference on Environmental Science and Technology. Rhodes, Greece, 4.
- Cernuschi, S., M. Giugliano, S. Ozgen, and S. Consonn. 2012. Number concentration and chemical composition of ultrafine and nanoparticles from WTE (waste to energy) plants. Sci. Total Environ. 420:319–326. doi:10.1016/j.scitotenv.2012.01.024.
- Chen, Z., C. You, H. Liu, and H. Wang. 2018. The synergetic particles collection in three different wet flue gas desulfurization towers: A pilot-scale experimental investigation. Fuel Process. Technol. 179:344–50. doi:10.1016/j.fuproc.2018.07.025.
- Cho, K. S., Y. Y. Lee, S. Jang, J. Nae, Yun, J. Kwon, H. J. Park, and Y. Seo. 2020. Removal of particulate matter from pork belly grilling gas using an orifice wet scrubber. J. Environ. Sci. Health. A Tox. Hazard. Subst. Environ. Eng. 55 (9):1125–30. doi:10.1080/10934529.2020.1773712.
- Commodo, M., G. De Falco, R. Larciprete, A. D’Anna, and P. Minutolo. 2016. On the hydrophilic/hydrophobic character of carbonaceous nanoparticles formed in laminar premixed flames. Exp. Therm. Fluid Sci. 73:56–63. doi:10.1016/j.expthermflusci.2015.09.005.
- Cui, H., N. Li, J. Peng, J. Cheng, N. Zhang, and Z. Wu. 2017. Modeling the particle scavenging and thermal efficiencies of a heat absorbing scrubber. Build. Environ. 111:218–27. doi:10.1016/j.buildenv.2016.11.006.
- Danzomo, B. A., M. J. E. Salami, S. Jabirin, M. R. Khan, and I. M. Nor. 2012. Performance evaluation of wet scrubber system for industrial air pollution control.
- DeCarlo, P. F., J. G. Slowik, D. R. Worsnop, P. Davidovits, and J. L. Jimenez. 2004. Particle morphology and density characterization by combined mobility and aerodynamic diameter measurements. Part 1: Theory. Aerosol Sci. Technol. 38 (12):1185–205. doi:10.1080/027868290903907.
- Derrough, S., G. Raffin, D. Locatelli, P. Nobile, and C. Durand. 2013. Behaviour of nanoparticles during high temperature treatment (Incineration type). J. Phys. Conf. Ser. 429 (1): 012047.
- Di Natale, F., C. Carotenuto, L. D’Addio, A. Jaworek, A. Krupa, M. Szudyga, and A. Lancia. 2015. Capture of fine and ultrafine particles in a wet electrostatic scrubber. J. Environ. Chem. Eng. 3 (1):349–56. doi:10.1016/j.jece.2014.11.007.
- EC. 2010. Commission recommendations. Nurs. Stand. 24 (26):6. doi:10.7748/ns.24.26.6.s4.
- Faisal, N., and K. Kumar. 2017. Polymer and metal nanocomposites in biomedical applications. Biointerface Research in Applied Chemistry 7:2286–2294.
- Faunce, T. 2017. POLICY AREA: 2030 Agenda Nanowaste: Need for disposal and recycling standards.
- Fuchs, N. 1964. The mechanics of aerosols, Rev. and enl. ed. Pergamon Press, Oxford.
- Gemci, T., and F. Ebert. 1992. Prediction of the particle capture efficiency based on the combined mechanisms (turbulent diffusion, inertial impaction, interception, and gravitation) by a 3-D simulation of a wet scrubber. J. Aerosol Sci. 23 (Suppl. 1):769–772. doi:10.1016/0021-8502(92)90525-Z.
- Grand View Research. 2020. Global nanomaterials market size report, 2020–2027. Accessed November 23, 2020. //www.grandviewresearch.com/industry-analysis/nanotechnology-and-nanomaterials-market.
- Ha, T. H., O. Nishida, H. Fujita, and H. Wataru. 2010. Enhancement of diesel particulate matter collection in an electrostatic water-spraying scrubber. J. Mar. Sci. Technol. 15 (3):271–279. doi:10.1007/s00773-010-0086-x.
- Heinzerling, A., J. Hsu, and F. Yip. 2016. Respiratory health effects of ultrafine particles in children: A literature review. Water. Air. Soil Pollut. 227(1). doi:10.1007/s11270-015-2726-6.
- Hu, S., Y. Gao, G. Feng, F. Hu, C. Liu, and J. Li. 2021. Experimental study of the dust-removal performance of a wet scrubber. Int. J. Coal Sci. Technol. 8:228–239. doi:10.1007/s40789-021-00410-y.
- Huang, C.-H., C.-J. Tsai, and Y.-M. Wang. 2007. Control efficiency of submicron particles by an efficient venturi scrubber system. J. Environ. Eng. 133 (4):454–461. doi:10.1061/(ASCE)0733-9372(2007)133:4(454).
- Huang, S. H., J. L. Kang, D. S. H. Wong, S. S. Jang, and C. A. Lin. 2020. Particle-Scavenging prediction in sieve plate scrubber via dimension reduction in computational fluid dynamics. Chem. Eng. Res. Des. 160:540–550. doi:10.1016/j.cherd.2020.06.024.
- Huang, Y., C. Zheng, Q. Li, J. Zhang, Y. Guo, Y. Zhang, and X. Gao. 2020. Numerical simulation of the simultaneous removal of particulate matter in a wet flue gas desulfurization system. Environ. Sci. Pollut. Res. Int. 27 (2):1598–1607. doi:10.1007/s11356-019-06773-9.
- Johnstone, H. F., and M. N. Roberts. 1949. Deposition of aerosol particles from moving gas streams. Ind. Eng. Chem. 41 (11):2417–2423. doi:10.1021/ie50479a019.
- Jung, C. H., and K. W. Lee. 1998. Filtration of fine particles by multiple liquid droplet and gas bubble systems. Aerosol Sci. Technol. 29 (5):389–401. doi:10.1080/02786829808965578.
- Kabir, E., V. Kumar, K. H. Kim, A. C. K. Yip, and J. R. Sohn. 2018. Environmental impacts of nanomaterials. J. Environ. Manage. 225:261–271. doi:10.1016/j.jenvman.2018.07.087.
- Kang, G. S., P. A. Gillespie, A. Gunnison, A. L. Moreira, K.-M. Tchou-Wong, and L.-C. Chen. 2011. Long-term inhalation exposure to nickel nanoparticles exacerbated atherosclerosis in a susceptible mouse model. Environ. Health Perspect. 119 (2):176–181. doi:10.1289/ehp.1002508.
- Keshavarz, P., Y. Bozorgi, J. Fathikalajahi, and M. Taheri. 2008. Prediction of the spray scrubbers’ performance in the gaseous and particulate scrubbing processes. Chem. Eng. J. 140 (1–3):22–31. doi:10.1016/j.cej.2007.08.034.
- Khan, I., K. Saeed, and I. Khan. 2019. Nanoparticles: Properties, applications and toxicities. Arab. J. Chem. 12(7):908–931. doi:10.1016/j.arabjc.2017.05.011.
- Kim, H. T., C. H. Jung, S. N. Oh, and K. W. Lee. 2001. Particle removal efficiency of gravitational wet scrubber considering diffusion, interception, and impaction. Environ. Eng. Sci. 18 (2):125–136. doi:10.1089/10928750151132357.
- Kim, J. S., and J. W. Park. 2020. A method of estimating aerosol particle removal rates using one-dimensional two-fluid equations for venturi scrubbers in filtered containment venting. Ann. Nucl. Energy. 145:107543. doi:10.1016/j.anucene.2020.107543.
- Krames, J., and H. Büttner. 1994. The cyclone scrubber – a high efficiency wet separator. Chem. Eng. Technol. 17 (2):73–80. doi:10.1002/ceat.270170202.
- Lai, K.-Y., N. Dayan, M. Kerker, K.-Y. Lai, N. Dayan, and M. Kerker. 1978. Scavenging of aerosol particles by a falling water drop. J. Atmosph. Sci. 35 (4):674–82. doi:10.1175/1520-0469(1978)035 < 0674:SOAPBA>2.0.CO;2.
- Lang, I.-M., M. Hauser, W. Baumann, H. Mätzing, H.-R. Paur, and H. Seifert. 2015. Untersuchungen zur Freisetzung von synthetischen Nanopartikeln bei der Abfallverbrennung, vivis.de.
- Lee, B.-K., B. R. Mohan, S.-H. Byeon, K.-S. Lim, and E.-P. Hong. 2013. Evaluating the performance of a turbulent wet scrubber for scrubbing particulate matter. J. Air Waste Manag. Assoc. 63 (5):499–506. doi:10.1080/10962247.2012.738626.
- Lee, J. 2008. Black carbon and elemental carbon concentrations of spark-generated carbon particles. ETH-Conference on combustion generated nanoparticles, Zurich, Switzerland, vol. 25 (2013001650004), 1–37.
- Licht, W. 1988. Air pollution control engineering: Basic calculations for particulate collection. Cambridge: CRC Press.
- Lim, K. S., S. H. Lee, and H. S. Park. 2006. Prediction for particle removal efficiency of a reverse jet scrubber. J. Aerosol Sci. 37 (12):1826–1839. doi:10.1016/j.jaerosci.2006.06.010.
- Maricq, M. M., N. Xu, and R. E. Chase. 2007. Measuring particulate mass emissions with the electrical low pressure impactor. Aeros. Sci. Technol. 40 (1):68–79. doi:10.1080/02786820500466591.
- Massari, A., M. Beggio, S. Hreglich, R. Marin, and S. Zuin. 2014. Behavior of TiO2 nanoparticles during incineration of solid paint waste: A lab-scale test. Waste Manag. 34 (10):1897–1907. doi:10.1016/j.wasman.2014.05.015.
- Mazari, S. A., E. Ali, R. Abro, F. S. A. Khan, I. Ahmed, M. Ahmed, S. Nizamuddin, T. H. Siddiqui, N. Hossain, N. M. Mubarak, et al. 2021. Nanomaterials: Applications, waste-handling, environmental toxicities, and future challenges – A review. J. Environ. Chem. Eng. 9 (2):105028. doi:10.1016/j.jece.2021.105028.
- Mi, T., and X. M. Yu. 2012. Dust removal and desulphurization in a novel venturi scrubber. Chem. Eng. Process. Process Intensif. 62:159–167. doi:10.1016/j.cep.2012.07.010.
- Mishra, A. K., R. Arya, and D. Panchal. 2020. Environmental nanotechnology: Global framework and integrative strategies of nanowaste management. Handbook Environ Mat Manag. 1–31. doi:10.1007/978-3-319-58538-3_188-1
- Mohan, B. R., S. Biswas, and B. C. Meikap. 2008. Performance characteristics of the particulates scrubbing in a counter-current spray-column. Sep. Purif. Technol. 61 (1):96–102. doi:10.1016/j.seppur.2007.09.018.
- Mueller, N., B. Nowack, J. Wang, A. Ulrich, and J. Buha. 2012. Nanomaterials in waste incineration and landfills. Intern. Empa-report. http://www.empa.ch/plugin/template/empa/*/124595.
- Mueller, N., J. Buha, J. Wang, A. Ulrich, and B. Nowack. 2013. Modeling the flows of engineered nanomaterials during waste handling. pubs.rsc.org.
- Muller, N., B. Benadda, and M. Otterbein. 2001. Mass transfer in a “droplets column” in presence of solid particles. Chem. Eng. Process. 40 (2):167–174. doi:10.1016/S0255-2701(00)00136-7.
- Nakao, S., P. Tang, X. Tang, C. H. Clark, L. Qi, E. Seo, A. Asa-Awuku, and D. Cocker. 2013. Density and elemental ratios of secondary organic aerosol: Application of a density prediction method. Atmos. Environ. 68:273–277. doi:10.1016/j.atmosenv.2012.11.006.
- Neuwahl, F., G. Cusano, J. G. Benavides, S. Holbrook, and R. Serge. 2019. Best available techniques (BAT) reference document for waste treatment industries. Industrial Emissions Directive 2010/75/EU (Integrated Pollution Prevention and Control) Frederik.
- Nurkiewicz, T. R., D. W. Porter, A. F. Hubbs, J. L. Cumpston, B. T. Chen, D. G. Frazer, and V. Castranova. 2008. Nanoparticle inhalation augments particle-dependent systemic microvascular dysfunction. Part. Fibre Toxicol. 5 (1):1–12. doi:10.1186/1743-8977-5-1.
- Oberdörster, G., E. Oberdörster, and J. Oberdörster. 2005. Nanotoxicology: An emerging discipline evolving from studies of ultrafine particles. Environ. Health Perspect. 113(7):823–839. doi:10.1289/ehp.7339
- Oischinger, J., M. Meiller, R. Daschner, A. Hornung, and R. Warnecke. 2019. Fate of nano titanium dioxide during combustion of engineered nanomaterial-containing waste in a municipal solid waste incineration plant. Waste Manag. Res. 37 (10):1033–1042. doi:10.1177/0734242X19862603.
- Ounoughene, G., C. Chivas-Joly, C. Longuet, O. Le Bihan, J. M. Lopez-Cuesta, and L. Le Coq. 2019. Evaluation of nanosilica emission in polydimethylsiloxane composite during incineration. J. Hazard. Mater. 371:415–422. doi:10.1016/j.jhazmat.2019.03.026.
- Ounoughene, G., O. Le Bihan, C. Chivas-Joly, C. Motzkus, C. Longuet, B. Debray, A. Joubert, L. Le Coq, and J. M. Lopez-Cuesta. 2015. Behavior and fate of halloysite nanotubes (HNTs) when incinerating pa6/HNTs nanocomposite. Environ. Sci. Technol. 49 (9):5450–5457. doi:10.1021/es505674j.
- Park, K., F. Cao, D. B. Kittelson, and P. H. McMurry. 2003. Relationship between particle mass and mobility for diesel exhaust particles. Environ. Sci. Technol. 37 (3):577–583. doi:10.1021/es025960v.
- Part, F., N. Berge, P. Baran, A. Stringfellow, W. Sun, S. Bartelt-Hunt, D. Mitrano, L. Li, P. Hennebert, P. Quicker, et al. 2018. A review of the fate of engineered nanomaterials in municipal solid waste streams. Waste Manag. 75:427–449. doi:10.1016/j.wasman.2018.02.012
- Perry, R. H., D. W. Green, and J. O. Maloney. 1997. Perry’s chemical engineers’ handbook, 7th ed. Blacklick, OH: McGraw-Hill.
- Pilat, M. J., and A. Prem. 1976. Calculated particle collection efficiencies of single droplets including inertial impaction, Brownian diffusion, diffusiophoresis and thermophoresis. Atmos. Environ 10 (1):13–19. doi:10.1016/0004-6981(76)90253-5.
- Pilat, M. J., S. A. Jaasund, and L. E. Sparks. 1974. Collection of aerosol particles by electrostatic droplet spray scrubbers. Environ. Sci. Technol. 8 (4):360–362. doi:10.1021/es60089a006.
- Pourchez, J., C. Chivas-Joly, C. Longuet, L. Leclerc, G. Sarry, and J. M. Lopez-Cuesta. 2018. End-of-life incineration of nanocomposites: new insights into nanofiller partitioning into by-products and biological outcomes of airborne emission and residual ash. Environ. Sci: Nano. 5 (8):1951–1964. doi:10.1039/C8EN00420J.
- Pranesha, T. S., and A. K. Kamra. 1996. Scavenging of aerosol particles by large water drops 1. Neutral case. J. Geophys. Res. 101 (D18):23373–23380. doi:10.1029/96JD01311.
- Rafidi, N., F. Brogaard, L. Chen, R. Håkansson, and A. Tabikh. 2018. CFD and experimental studies on capture of fine particles by liquid droplets in open spray towers. Sustain. Environ. Res. 28 (6):382–388. doi:10.1016/j.serj.2018.08.003.
- Raj Mohan, B., R. K. Jain, and B. C. Meikap. 2008. Comprehensive analysis for prediction of dust removal efficiency using twin-fluid atomization in a spray scrubber. Sep. Purif. Technol. 63 (2):269–277. doi:10.1016/j.seppur.2008.05.006.
- Ristimäki, J., A. Virtanen, M. Marjamäki, A. Rostedt, and J. Keskinen. 2002. On-line measurement of size distribution and effective density of submicron aerosol particles. J. Aerosol Sci. 33 (11):1541–1557. doi:10.1016/S0021-8502(02)00106-4.
- Ru, Y., L. Zhao, L. J. S. Hadlocon, H. Zhu, and S. K. Ramdon. 2017. Laboratory evaluation of electrostatic spray wet scrubber to control particulate matter emissions from poultry facilities. Environ. Technol. 38 (1):23–33. doi:10.1080/09593330.2016.1184319.
- Schifftner, K. C. 2013. Air pollution control equipment selection guide. Boca Raton, FL: CRC Press.
- Schnelle, K. B., and C. A. Brown. 2002. Air pollution control technology handbook. Boca Raton, FL: CRC Press.
- Schraufnagel, D. E. 2020. The health effects of ultrafine particles. Exp. Mol. Med. 52 (3):311–317. doi:10.1038/s12276-020-0403-3.
- Seinfeld, J. H., and S. N. Pandis. 2006. Atmospheric chemistry and physics: From air pollution to climate change. New York: J. Wiley.
- Sidiropoulou, E., K. Feidantsis, S. Kalogiannis, G. P. Gallios, G. Kastrinaki, E. Papaioannou, M. Václavíková, and M. Kaloyianni. 2018. Insights into the toxicity of iron oxides nanoparticles in land snails. Comp Biochem Physiol C Toxicol Pharmacol. 206–207:1–10. doi:10.1016/j.cbpc.2018.02.001.
- Smita, S., S. K. Gupta, A. Bartonova, M. Dusinska, A. C. Gutleb, and Q. Rahman. 2012. Nanoparticles in the environment: Assessment using the causal diagram approach. Environ. Heal. A Glob. Access Sci. Source. 11:S13. doi:10.1186/1476-069X-11-S1-S13
- Su, L., Y. Zhang, Q. Du, X. Dai, J. Gao, P. Dong, and H. Wang. 2020. An experimental study on the removal of submicron fly ash and black carbon in a gravitational wet scrubber with electrostatic enhancement. RSC Adv. 10 (10):5905–5912. doi:10.1039/C9RA10046F.
- Teleanu, D., C. Chircov, A. Grumezescu, A. Volceanov, and R. Teleanu. 2018. Impact of nanoparticles on brain health: An up to date overview. J. Clin. Med 7 (12):490. doi:10.3390/jcm7120490.
- Thomas, S., E. Al Mutairi, and S. De. 2013. Impact of nanomaterials on health and environment. Arab. J. Sci. Eng. 38 (3):457–477. doi:10.1007/s13369-012-0324-0.
- Tomb, T. F., J. E. Emmerling, and R. H. Kellner. 1972. Collection of airborne coal dust by water spray in a horizontal duct. Am. Ind. Hyg. Assoc. J. 33 (11):715–721. doi:10.1080/0002889728506736.
- Tsai, C.-J., C.-H. Lin, Y.-M. Wang, C.-H. Hunag, S.-N. Li, Z.-X. Wu, and F.-C. Wang. 2005. An efficient venturi scrubber system to remove submicron particles in exhaust gas. J. Air Waste Manag. Assoc. 55 (3):319–325. doi:10.1080/10473289.2005.10464622.
- Vallero, D. A. 2019. Air pollution control technologies (Chapter 13, D.A. Vallero, ed.). Winston, NC: Elsevier, 377–428.
- Vasudevan, T. V., A. J. Gokhale, and R. Mahalingam. 1985. Phoretic phenomena in tar vapor-particulate mixture separation from fuel gas streams. Can. J. Chem. Eng. 63 (6):903–910. doi:10.1002/cjce.5450630606.
- Vejerano, E. P., A. L. Holder, and L. C. Marr. 2013. Emissions of polycyclic aromatic hydrocarbons, polychlorinated dibenzo-p-dioxins, and dibenzofurans from incineration of nanomaterials. Environ. Sci. Technol. 47 (9):4866–4874. doi:10.1021/es304895z.
- Vejerano, E. P., E. C. Leon, A. L. Holder, and L. C. Marr. 2014. Characterization of particle emissions and fate of nanomaterials during incineration. Environ. Sci. Na 1 (2):133–143. doi:10.1039/C3EN00080J.
- Vittori Antisari, L., S. Carbone, S. Bosi, A. Gatti, and G. Dinelli. 2018. Engineered nanoparticles effects in soil-plant system: Basil (Ocimum basilicum L.) study case. Appl. Soil Ecol 123:551–560. doi:10.1016/j.apsoil.2018.01.007.
- Walser, T., L. K. Limbach, R. Brogioli, E. Erismann, L. Flamigni, B. Hattendorf, M. Juchli, F. Krumeich, C. Ludwig, K. Prikopsky, et al. 2012. Persistence of engineered nanoparticles in a municipal solid-waste incineration plant. Nat. Nanotechnol. 7 (8):520–524. doi:10.1038/nnano.2012.64.
- Wang, P. K., and H. R. Pruppacher. 1980. The effect of an external electric field on the scavenging of aerosol particles by cloud drops and small rain drops. J. Colloid Interface Sci 75 (1):286–297. doi:10.1016/0021-9797(80)90370-7.
- WHO. 2006. Regional Office for Europe & Joint WHO/Convention Task Force on the Health Aspects of Air Pollution. Health risks of particulate matter from long-range transboundary air pollution. Copenhagen: WHO Regional Office for Europe. https://apps.who.int/iris/handle/10665/107691.
- Wu, Q., M. Gu, Y. Du, and H. Zeng. 2019. Synergistic removal of dust using the wet flue gas desulfurization systems. R. Soc. Open Sci. 6 (7):181696. doi:10.1098/rsos.181696.
- Xiong, H., and W. Sun. 2017. Investigation of droplet atomization and evaporation in solution precursor plasma spray coating. Coatings 7 (11):207. doi:10.3390/coatings7110:207.
- Yalamov, Y. I., L. Y. Vasiljeva, and E. R. Schukin. 1977. The study of various mechanisms of in-cloud scavenging of large, moderately large, and small aerosol particles. J. Colloid Interface Sci. 62 (3):503–508. doi:10.1016/0021-9797(77)90101-1.
- Yang, L., J. Bao, J. Yan, J. Liu, S. Song, and F. Fan. 2010. Removal of fine particles in wet flue gas desulfurization system by heterogeneous condensation. Chem. Eng. J. 156 (1):25–32. doi:10.1016/j.cej.2009.09.026.
- Yin, Z., X. Ye, S. Jiang, Y. Tao, Y. Shi, X. Yang, and J. Chen. 2015. Size-resolved effective density of urban aerosols in Shanghai. Atmos. Environ. 100:133–140. doi:10.1016/j.atmosenv.2014.10.055.
- Zhang, B., C. Hu, J. He, C. Sui, and W. Chen. 2020. IOP Conference Series: Earth and Environmental Science Performance evaluation of a three-stage composite wet scrubber for removing particulate matter Performance evaluation of a three-stage composite wet scrubber for removing particulate matter. doi:10.1088/1755-1315/615/1/012117.
- Zhang, H., Y. Dong, Y. Lai, H. Zhang, and X. Zhang. 2021. Synergistic removal of particles, SO2, and NO2 in desulfurized flue gas during condensation. Environ. Sci. Pollut. Res. Int. 28 (21):27273–27282. doi:10.1007/s11356-020-12192-y.
- Zhao, H., and C. G. Zheng. 2008. Modeling of Gravitational Wet Scrubbers with Electrostatic Enhancement. Chem. Eng. Technol. 31 (12):1824–1837. doi:10.1002/ceat.200800360.
- Zheng, Z., J. Zhu, S. Xia, and Q. Zeng. 2018. An efficient wet scrubber to remove micron and submicron particles from exhaust gas. J. Chem. Eng. Japan. 51 (10):839–847. doi:10.1252/jcej.17we167.
- Zhou, J., S. Zhou, and Y. Zhu. 2017. Experiment and prediction studies of marine exhaust gas SO2 and particle removal based on NaOH solution with a U-type scrubber. Ind. Eng. Chem. Res. 56 (43):12376–12384. doi:10.1021/acs.iecr.7b02397.