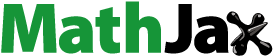
Abstract
Airborne transmission of infectious (viable) SARS-CoV-2 is increasingly accepted as the primary manner by which the virus is spread from person to person. Risk of exposure to airborne virus is higher in enclosed and poorly ventilated spaces. We present a study focused on air sampling within residences occupied by individuals with COVID-19. Air samplers (BioSpot-VIVAS, VIVAS, and BC-251) were positioned in primary- and secondary-occupancy regions in seven homes. Swab samples were collected from high-touch surfaces. Isolation of SARS-CoV-2 was attempted for samples with virus detectable by RT-qPCR. Viable virus was quantified by plaque assay, and complete virus genome sequences were obtained for selected samples from each sampling day. SARS-CoV-2 was detected in 24 of 125 samples (19.2%) by RT-qPCR and isolated from 14 (11.2%) in cell cultures. It was detected in 80.9% (17/21) and cultured from 61.9% (13/21) of air samples collected using water condensation samplers, compared to swab samples which had a RT-qPCR detection rate of 10.5% (4/38) and virus isolation rate of 2.63% (1/38). No statistically significant differences existed in the likelihood of virus detection by RT-qPCR or amount of infectious virus in the air between areas of primary and secondary occupancy within residences. Our work provides information about the presence of SARS-CoV-2 in the air within homes of individuals with COVID-19. Information herein can help individuals make informed decisions about personal exposure risks when sharing indoor spaces with infected individuals isolating at home and further inform health departments and the public about SARS-CoV-2 exposure risks within residences.
Graphical Abstract
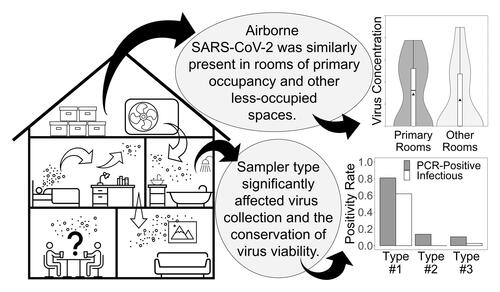
Editor:
1. Introduction
Epidemiological and clinical data during the Coronavirus Disease 2019 (COVID-19) pandemic indicate significant SARS-CoV-2 infection risk in indoor spaces shared with an infected individual, leading to outbreaks within households (Qian et al. Citation2021). Apart from medical facilities, most indoor spaces are not designed for infection control and have much lower air exchange rates (Allen and Ibrahim Citation2021). With infected individuals isolating themselves in their residences following diagnosis of COVID-19 and clinical data showing some individuals can shed infectious SARS-CoV-2 for more than 5 days after symptom onset or an initial positive test (Boucau et al. Citation2022), co-inhabitants of a household with an infected person are subject to prolonged periods of high exposure risks (Wang et al. Citation2020).
Two dominant analytical approaches are used to monitor SARS-CoV-2 in environmental air samples: (1) real-time quantitative polymerase chain reaction (RT-qPCR) analyses performed to detect the presence of viral RNA and determine the virus concentration in a sample, and (2) attempting to isolate the virus in cell cultures to determine if the virus detected by RT-qPCR is infectious (‘viable’). While it has been a common approach to detect and quantify SARS-CoV-2 genomes in environmental samples by conducting RT-qPCR analysis (Linde et al. Citation2023; Lind et al. Citation2022; Nannu Shankar et al. Citation2022; Vass et al. Citation2022; Lednicky et al. Citation2020b, Citation2021; Huang et al. Citation2020), fewer attempts have been made toward the cell culture isolation of the virus from environmental air samples, especially as biosafety level 3 laboratories were not available for that type of work in a majority of the studies (Fortin et al. Citation2023; Kitagawa et al. Citation2023; Santarpia et al. Citation2022; Vass et al. Citation2022; Lednicky et al. Citation2020a). Importantly, the concentration of virus determined by RT-qPCR differs from that determined by infectivity assays, since the virus genomes detected by RT-qPCR may stem from inactivated or defective virions. The air we breathe is full of viruses, but only those that are viable and able to infect humans are able to sicken us. Though it is critical to determine the infectivity of pathogens in the environment to determine the real risk associated with exposure to viruses, isolating viruses from air samples is challenging since there is no standard air sampler or protocol for air sampling for airborne viruses (Pan, Lednicky, and Wu Citation2019). Viruses differ according to their biophysical and biochemical characteristics; thus, a universal air sampler or standard collection method is not feasible for all classes of viruses. In addition, many factors including ventilation practices, relative humidity, temperature, and sample collection mechanisms influence the viability of viruses in the air.
To assess the presence of airborne infectious virus within residences, it is important to collect air both from spaces frequented by sick persons as well as from living spaces not often used. In this study, air samples were collected from the homes of individuals within 5 days of testing positive for SARS-CoV-2, a time period selected because the shedding of infectious virus occurs most often within the first week following onset of symptoms (Hakki et al. Citation2022). We selected residences as a general type of indoor space for investigation due to characteristically low air exchange rates as compared to communal or medical buildings and due to evidence from past studies on associated exposure risks (Myers et al. Citation2022; Vass et al. Citation2022), which demonstrated potential for the presence of viable virus in air samples from similar environments. Multiple air samplers were employed in this study, positioned in: (1) the room occupied most frequently by the infected individual, and (2), shared spaces, such as a living room, or other room less frequently visited by the infected individual. We then determined SARS-CoV-2 concentrations in collected air samples and on surface swab samples by RT-qPCR and by viable virus counts using plaque assays.
2. Materials and methods
This environmental sampling work was IRB-exempt as it did not involve research with human subjects, and information that may reveal the identity of the volunteers was not collected. After the volunteers’ verbal consent, air samplers were positioned in their residences, and air and surface samples collected.
2.1. Sampling sites and sampler location
Environmental sampling was conducted at the residences of seven volunteers, of which one resided in a single-family home and the others in multi-story complexes (Table S1). Five of the residences had four bedrooms and two had two bedrooms. All residences were located in North Central Florida, USA. The sampling locations were categorized as “primary” and “secondary,” corresponding to the location where the infected individual spent the most and the least time, respectively. The categorization was based on the volunteers’ information and the movements of the occupants were not monitored. Air samplers were set at fixed points in the rooms, with the sampling inlets ∼1.5-1.8 m from the ground. Within primary rooms, samplers were ∼1.5–1.8 m away from where infected individuals were expected to be, at locations that did not interfere with normal activities of the occupants. The condensation and non-condensation samplers discussed in section 2.2.2 were positioned so that both sampler types were in primary and secondary locations in each residence. The samplers were generally placed away from overhead fans and heating, ventilation, and air conditioning (HVAC) air supply features whenever possible.
2.2. Collection of samples
2.2.1. Collection medium
Aqueous virus transport medium (VTM) used in prior studies for the collection of airborne SARS-CoV-2 (Nannu Shankar et al. Citation2022; Vass et al. Citation2022) was used during its collection, and for the maintenance of its viability, in this study.
2.2.2. Air sampling
Viable Virus Aerosol Sampler (VIVAS, Aerosol Dynamics Inc, USA), BioSpot-VIVAS (Model 300, Aerosol Devices Inc, USA), and NIOSH bioaerosol samplers (Model BC-251) were used to collect air samples, depending on the availability of the samplers and the layout of the sampling site. The principles of operation of the BioSpot-VIVAS, VIVAS and the BC-251 were previously described (Nannu Shankar et al. Citation2022; Lednicky et al. Citation2020b; Cao et al. Citation2010; Lindsley, Schmechel, and Chen Citation2006). Briefly, the BioSpot-VIVAS is the commercialized version of VIVAS that has been fitted with external hardware to make it more durable during use outside of the laboratory. Functionally, both systems operate by applying the same water-based condensational growth technology, which deposits water molecules onto the surfaces of airborne particles, enlarging their outer diameters as the particles move through the sampler. The growing layers of water molecules that form around the particles make them heavier, and these size-amplified droplets are collected by gentle impaction into VTM in a 35 × 10 mm Petri dish (ThermoFisher Scientific, USA). Both VIVAS and Bio-Spot VIVAS collected air samples at a flow rate of 8 LPM for 3 hr. A flow rate of 8 LPM was used as it corresponds to the average amount of air that is breathed in per minute by a resting adult human in an upright sitting posture (Landers et al. Citation2003). The BC-251 consists of three stages: a first-stage cyclone with 15 mL centrifuge tube (Fisher Scientific, USA), a second-stage cyclone with 1.5 mL Eppendorf tube (Fisher scientific, USA), and a third stage polytetrafluoroethylene (PTFE) filter (37 mm, 2 μm pore, SKC Inc., USA). The BC-251 was connected to an Airchek pump (Model 224-PCXR4, SKC Inc., USA) and operated for 3 hr. At a flow rate of 3.5 LPM, the three stages of the BC-251 collected particles of aerodynamic diameters >4 μm, 1–4, μm and <1 μm, respectively. Indoor temperature and relative humidity (RH) were recorded using a digital temperature and humidity meter (Model CECOMINOD002312, Mengshen, China) co-located with air samplers at both the start and end of sampling, and average values were used for data analysis.
2.2.3. Surface sampling
Areas that were self-reported by the volunteers as frequently touched were swabbed with sterile nylon swabs (Nasal Flocked Swab, part no. 96000, Miraclean Technology Co, Ltd., China) pre-wetted with VTM. A precut template (15.2 cm × 15.2 cm) or a LASER measure (GLM165-40, Robert Bosch GmbH, Gerlingen, Germany) was used to outline the area to be swabbed. The surfaces sampled included mobile phones, desks, tabletops, doorknobs, refrigerator handles, and television remote controls. After sampling, the swabs were immersed in 2 mL of VTM in a screw cap tube, and the tubes kept in wet ice for transport to the laboratory, where they were subsequently stored at −80 °C until further analyses could be performed.
2.3. Analyses of samples and data
Air and surface samples were processed as detailed in our previous reports (Nannu Shankar et al. Citation2022; Vass et al. Citation2022). RT-qPCR, isolation of SARS-CoV-2 in Vero E6 cells and LLC-MK2 cells, plaque assay and Sanger sequencing for the complete SARS-CoV-2 genome were conducted by methods established previously (Nannu Shankar et al. Citation2022; Vass et al. Citation2022; Lednicky et al. Citation2020a, Citation2020b). Data were tabulated, and unit conversions made using Microsoft Excel. Data sorting was performed according to “Sampler Type” which pertains to the particle collection (condensation, non-condensation & swab), “Instrument” which specifies the device being used (BC-251, VIVAS, BioSpot-1 & BioSpot-2), and “Location” that pertains to primary or secondary occupancy areas. Sorted data were then exported to a .csv file and imported into R for statistical analyses. Mixed effects hurdle models were applied to air sample results to determine whether target explanatory variables exerted significant impact on virus detectability and viability while considering variation due to changes between volunteer residences. Variables with significant impact according to the hurdle models or otherwise of further interest were then assessed by Wilcoxon rank sum tests for pairwise impact. Wilcoxon rank sum tests were used for any variables that could not be examined with the hurdle models due to collinearity. Overall positivity rates of both RT-qPCR and cell culture analyses were completed by sampler type using Fisher’s exact test. Results were considered significantly different if the p-value was ≤0.05. Further elaboration on the analytical methods is included in the online supplemental information.
3. Results
125 samples were collected, 49 from primary and 76 from secondary occupancy spaces. These consisted of 38 swab samples (30.4%) taken from high-touch surfaces, and 87 air samples (69.6%). Of those 87 air samples, 66 were from the three-stage BC-251. After combining individual stage samples from the same sampler to provide a fairer comparison with condensation samplers, 22 BC-251 samples and 43 total air samples remained for data analysis. Indoor ambient temperatures across all sample locations ranged from 20.2 °C to 26.1 °C, and relative humidity ranged from 48.8% to 74.8% (Table S2).
Of the 43 air samples, SARS-CoV-2 RNA was detectable by RT-qPCR in 20 (46.5%), and infectious SARS-CoV-2 in 14 (32.6%) (). Among 38 surface samples, SARS-CoV-2 was detected in four (10.5%) and infectious virus was isolated from one (2.63%). The virus detection rate by sampler type was 80.9%, 13.6%, and 10.5% for water condensation devices, non-condensation samplers, and surface swabs, respectively. Likewise, the viable virus isolation rate by sampler type was 61.9%, 0%, and 2.63%. All three samples from non-condensation samplers with PCR-detectable virus came from the second BC-251 cyclone stage (1.1-4.4 μm particles). Fisher’s exact tests on RT-qPCR (p = 1.04E-07) and infectious virus (p = 1.84 E-07) positivity rates indicated significant differences between air sampler types ().
Figure 1. A comparison of results from field samples in three categories: Total - (dark gray), PCR-positive - (light gray), and infectious samples (white). Swab samples are from high-touch surfaces, and all other samples are from air samplers. (a) Shows sample counts by Instrument. The BC-251 contains two cyclone and one filter sample collection stages. 66 individual BC-251 samples are shown here as 22 since all three stages from a single sampler were combined. Only the second BC-251 cyclone stage (1-4 μm particles) collected detectable virus. BioSpot-1 and BioSpot-2 refer to two separate instruments of the same model which, along with VIVAS, employ water-based condensation particle growth technology (b) Depicts sample positivity rates by Sampler Type. BioSpot-1, BioSpot-2, and VIVAS comprise the “Condensation” group based on their common particle collection mechanism. BC-251 samples fully comprise the “Non-Condensation” group. By Fisher’s exact tests, significant difference exists between these rates based on type of air sampler (p ≪ 0.05).
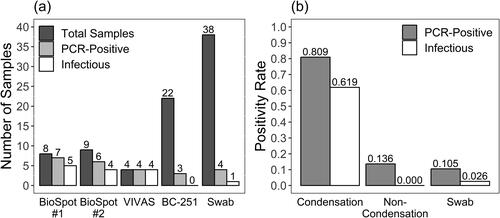
SARS-CoV-2 isolated from all residences was determined by Sanger sequencing to belong to the omicron-lineage subvariants. Three separate subvariants were identified: Pango Lineages, BA.1.1, BA.2, and BA.2.12.1 (Table S3), and associated sequences were deposited in GenBank. The hurdle model () applied to estimates of the total virus counts/L of air (“total virus”), expressed as SARS-CoV-2 genome equivalents (GE)/L of air, indicated significantly lower odds of failing to detect total virus in air samples collected with condensation samplers versus non-condensation samplers (). The model estimated no difference in the average concentration of total virus collected due to effects from sampler type, location, temperature, or relative humidity within this dataset. Sampler type could not be included as a variable in the viable virus model () because only condensation samplers collected viable virus.
Figure 2. SARS-CoV-2 detected by RT-qPCR according to the Sampler Type used to collect air samples, depicted with (a) all data included and samples with non-detectable virus shown as zero-values, and (b) only samples where virus was detected. Significant p-values according to Wilcoxon rank sum tests are shown with asterisks, and “ns” indicates no statistically significant difference.
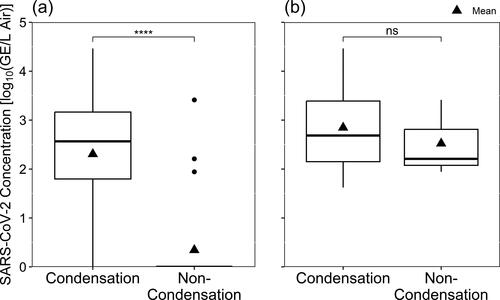
Table 1. Results from mixed effects hurdle models.
Viable virus model estimates, reported for plaque-forming units (PFU)/100 L of air, suggested only one significant effect on the average concentration of viable virus stemming from temperature. The effect, while marginally-significant according to the model, was sensitive to a single, highly-leveraged outlier. The removal of that one impactful outlier from the 13-sample subset made the impact of temperature no longer significant (p = 0.492).
Wilcoxon rank sum tests () confirmed that the median concentration of virus detected in samples collected by condensation devices was significantly different from what was detected in air samples from non-condensation samplers.
Table 2. Wilcoxon rank sum tests results following pairwise comparisons within categorical explanatory variables.
Those tests also indicated that no significant differences existed between the different condensation samplers. The median concentration of virus detected in samples collected by condensation samplers was larger than the median concentration from non-condensation samplers, however the difference was not statistically significant ().
Sampling location within residences did not create significant differences in either total virus collected () or amount of infectious virus cultured ().
4. Discussion
The omicron variant of concern spread rapidly (Callaway Citation2022) beginning at the end of 2021 and quickly overtook the previously-dominant delta variant (Lambrou et al. Citation2022) despite inconclusive evidence about differences in the amount of virus shed in exhaled breath in a controlled, clinical environment (Lai et al. Citation2022). Epidemiological data and assessment of the omicron variant spike protein structure suggested that the new variant was more transmissible than earlier variants (Ren et al. Citation2022). Here, we report that viable SARS-CoV-2 was collected from the air in a variety of residences over five months. The viruses we collected were identified as three subvariants of omicron-clade SARS-CoV-2. The detection and isolation of the subvariants from the air of residential spaces occurred during the time periods when they were the dominant clade in clinical sample data reported by the US CDC (Centers for Disease Control Citation2022) and of nearly identical SARS-CoV-2 sequences deposited in GenBank during the corresponding periods (NCBI Citation2022). Therefore, this work indicates that environmental monitoring by the collection of samples from ambient air might be useful as a pathogen surveillance tool to detect viruses circulating in a community if clinical test results are insufficient for public health needs or are altogether unavailable.
Studies similar to ours were previously conducted in residential spaces, with varying levels of successful virus recovery. For example, Döhla et al. (Citation2022) collected 15 air samples among 200 total environmental samples at the beginning of the pandemic in March 2020 using a wetted cyclone but were unable to detect SARS-CoV-2. Myers et al. (Citation2022) conducted residential air sampling when alpha, iota, gamma, and delta variants were dominant in the United States and reported detecting virus in 14 of 34 samples collected on membrane filters in primary and secondary spaces of 17 different homes. Their sampling periods were much longer than those used here, and that study did not have within its objectives the isolation of viable virus or quantification of total virus. This research group previously reported the detection and isolation of delta variant SARS-CoV-2 collected from air in a residence (Vass et al. Citation2022) with total virus concentrations similar to those reported in this study. It is interesting that the airborne viable virus concentrations reported in our previous work (132 and 292 PFU/L) were higher than the distribution among samples with culturable virus reported here (median = 3.85 PFU/L, maximum = 65.2 PFU/L). Such a finding of low viable virus concentrations in residential air relative to the delta variant could support the claim that omicron subvariants are more transmissible than previous variants (Allen et al. Citation2023) when considered in light of epidemiological reports showing greater transmissibility of omicron subvariants (Vogel Citation2023; WHO Citation2022; Torjesen Citation2021). However, we hold that a claim about omicron subvariant transmission at lower airborne concentrations would require a more controlled study design and larger sample size. We suggest that the most likely explanation for lower viable concentrations in this work is linked to the free movement of volunteers within their residences versus self-isolation in a dedicated space in Vass et al. (Citation2022). Regardless, the continued presence of viable virus in ambient air samples supports the notion that omicron subvariants retain characteristic potential for transmission in aerosols as has been posited for other variants of SARS-CoV-2.
Previous work from the periods when alpha and delta variants of SARS-CoV-2 predominated revealed that it is possible to detect virus in air samples within and beyond residential spaces designated as true isolation rooms (Vass et al. Citation2022), selected as primary occupancy regions (Myers et al. Citation2022), and within congregant housing facilities (Ramuta et al. Citation2022). Like those studies, this study was not designed to determine how virus might move from the source to other spaces within a residence. Participants were not required to remain in a single room of their homes, but, like Myers et al. (Citation2022), the placement of samplers was recorded as being either in a ‘primary’ location, where volunteers said they would spend most of their time, or ‘secondary’ locations, where volunteers would go less frequently. We did not identify a statistically significant difference in the likelihood of detecting virus by RT-qPCR () or isolating virus in cell cultures () based on sampling location within the home, nor did we see any significant difference in the average concentrations of virus based on location ( and ).
This consistent statistical similarity indicates that SARS-CoV-2 was recovered from the air throughout our sampled residential environments. These results provide evidence that persons living with symptomatic COVID-19 cases are at risk of exposure to SARS-CoV-2 in the air and should consider using personal protective measures, even when spatially separated from sick individuals. Examples of potential protective measures include wearing a facemask (de Man et al. Citation2022; Lindsley et al. Citation2021) or modifying the environment to increase the air exchange rate. The latter could be accomplished simply by opening windows or by employing a device like a Corsi-Rosenthal box or portable air cleaner (Myers et al. Citation2022; Lindsley et al. Citation2021). Further, when the presence of infectious virus in the air throughout these sampled residences is considered with known characteristics of COVID-19, namely that some infectious COVID-19 cases either remain asymptomatic or become infectious before exhibiting symptoms (Huang Citation2022; Huang et al. Citation2020), the wearing of well-fitted facemasks (Rahman et al. Citation2022) by co-occupants of a residence where someone is sick with COVID-19 may help mitigate the risk of SARS-CoV-2 transmission.
The selection of the right air sampler for a given sampling environment and target bioaerosol is critical to improving the likelihood of collecting data that are helpful toward characterizing health risks within the environment. Here, we employed two types of low-flow air samplers, the condensation BioSpot-VIVAS/VIVAS, and non-condensation BC-251. We assessed their relative success at both collecting detectable quantities of virus and conserving virus viability throughout the sampling process. Condensation devices all performed comparably () and were much more likely to collect detectable quantities of virus in air samples (). Though samplers collected air from the same environments at the same time, this higher likelihood of detection could be due to the lower airflow rate of the BC-251 as compared to BioSpot-VIVAS and VIVAS. This seems to be an incomplete answer, however, when considering samples with detectable concentrations of virus (). The median airflow-adjusted concentrations from condensation and non-condensation samplers were not statistically different. This signifies that when the BC-251 was able to collect total virus, it did so with an efficiency similar to the condensation samplers. A complication compounding the low airflow rate of the BC-251 was its size-fractionating of particles. Particles collected by the BC-251 would have been distributed across two cyclone stages and one filter stage. That division could have reduced collected virus below detectable limits by molecular tests and thus increased the likelihood of false-negatives. When enough virus was present to allow detection, however, the measurable performance of the BC-251 pertaining to total virus collection was statistically similar to condensation devices. Still, different flow rates likely provide part of the reason condensation samplers more successfully collected total virus than non-condensation samplers since increased flowrate improves the likelihood of collecting virus particles often dilute in ambient air.
If considering airflow rate differences, better performance of higher-flow air samplers when detecting virus by RT-qPCR would not be surprising as such samplers have been used to successfully collect virus for similar molecular tests. Yet such high-flow devices often create conditions that are detrimental to the conservation of viable virus relative to low-flow samplers (Raynor et al. Citation2021). Similarly, particle collection mechanisms like filtration and impaction onto dry surfaces have been shown to inactivate viable virus (Pan, Lednicky, and Wu Citation2019). Our results show that, even at low flowrates, water condensation devices were capable of consistent conservation of viable virus at significantly higher rates as compared to the non-condensation cyclone and filter stages of the BC-251 operating in the same environment (). Enhancement of the knowledge related to the presence of infectious virus in the air is critical for reducing the spread of respiratory viruses. As researchers seek to build that understanding and better inform the public about exposure risks and helpful protective measures, they ought to consider the use of samplers that have demonstrated the ability to conserve infectious virus (Pan et al. Citation2017).
Only four samples with virus RNA detectable by RT-qPCR and one sample with viable virus were obtained from swab samplings of high-touch surfaces (). Though Wong et al. (Citation2022) were able to detect SARS-CoV-2 RNA in 21 of 39 swabs from a single room when the omicron variant was first appearing, other samplings during that same study and environmental swab sampling before omicron appeared generally have reported detection rates of virus from surface swab samples similar to ours (Vass et al. Citation2022; Wong et al. Citation2022; Liu et al. Citation2021; Cheng et al. Citation2020). Here, samples that contained detectable virus all came from either a mobile phone or computer (), and those surfaces were shown to have a higher rate of successful detection of SARS-CoV-2 in past studies (Wong et al. Citation2022; Liu et al. Citation2021). Similarly, virus viability was conserved only in a mobile phone sample, a circumstance also found in previous work (Vass et al. Citation2022). Our results indicate that the likelihood of recovering virus with swabs, even from many high-touch surfaces, is low and may be maximized by sampling surfaces on objects that are frequently used near the breathing zones of sick individuals. This frequent usage of a handheld device within one meter may allow the deposition of large droplets containing greater quantities of virus (Wang et al. Citation2021) and may explain why SARS-CoV-2 has been better detected on handheld devices and surfaces close to patients.
Figure 5. Resultant concentration of SARS-CoV-2 detected by RT-qPCR in surface swab samples, shown with all sample data included and undetectable virus concentrations plotted as zero-values (All Samples) and with only samples containing detectable virus concentrations (Positives).
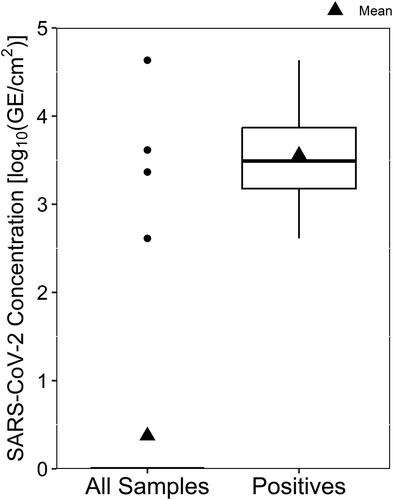
Table 3. Detection of SARS-CoV-2 and culture of viable virus from surface swabs.
We acknowledge that our work had several limitations, namely, environmental and study design factors. First, environmental factors varied widely between buildings and individuals. Though most residences can be grouped together as apartments, the design of apartments varies widely by construction, HVAC system design, and HVAC operation. Behavioral patterns, disease progression, and virus subvariants specific to individuals all could have introduced confounding errors into our dataset. Such factors likely contributed to the variance between volunteers indicated by both hurdle models. Second, the small sample size and the lack of scripted actions by participants further added potential bias, reduced statistical power, and introduced uncertainty due to the natural movement of volunteers out of primary spaces within their homes. Third, due to the opportunistic nature of the samplings included in this work, samples were not collected from individuals; hence, the relationship between individual and environmental SARS-CoV-2 sequences could not be determined. Fourth, while the BC-251 uses two different non-condensation particle collection mechanisms, its particle size-fractionating design leaves open the question about how well other cyclones or filters without size fractionation could have collected virus from these environments. The limitations evident in this work indicate a need for further controlled studies that are able to account for environmental variation, employ an array of sampler types, and involve enough participants to overcome statistical power hurdles.
5. Conclusions
SARS-CoV-2 was detected in 80.9% (17/21) of air samples collected from residential settings with condensation-based air samplers, compared to only 13.6% (3/22) from non-condensation-based air samplers and 10.5% (4/38) from surface swabs. Infectious SARS-CoV-2 was isolated successfully from 65.0% (13/20) of air samples and 25.0% (1/4) of surface samples that tested positive by RT-qPCR. Infectious virus isolated from air samples collected within and beyond areas of primary occupancy in homes corresponded to three omicron subvariants. Airborne virus concentrations were similar when compared by resident-reported room use frequency. Our work builds knowledge about the existence of infectious SARS-CoV-2 in the air. The demonstrated presence of virus beyond primary-occupancy spaces provides health agencies with information to apply to recommendations regarding airborne exposure risks in homes of sick individuals, such as enhancing air exchange rates, operating air purifiers, and using personal respiratory protection devices.
Supplemental Material
Download MS Word (377.3 KB)Acknowledgements
Tuition for William B. Vass was paid through the U.S. Army Medical Service Corps Long-Term Health Education and Training fellowship, and for Sripriya Nannu Shankar through NIH-NCATS under UF and FSU CTSI awards (TL1TR001428 & UL1TR001427). The authors thank Dr. William G. Lindsley of the National Institute for Occupational Safety and Health for the free loan of NIOSH BC-251 samplers; Dr. David Kaplan, University of Florida, for providing access to the official State of Florida vehicle; and Aerosol Devices, Inc., for maintenance support for BioSpot-VIVAS. The authors extend their gratitude to Holly Ryan, Tsion Shimola, and Moiz Usmani for their assistance in conducting the study.
Disclosure statement
Aerosol Dynamics Inc. holds the rights to the patent underlying the laminar flow, water-based condensational particle growth for concentrated collection of airborne particles (US-20140060155).
Additional information
Funding
References
- Allen, J. G., and A. M. Ibrahim. 2021. Indoor air changes and potential implications for SARS-CoV-2 transmission. Jama 325 (20):2112–2113. doi: 10.1001/jama.2021.5053.
- Allen, H., E. Tessier, C. Turner, C. Anderson, P. Blomquist, D. Simons, A. Løchen, C. I. Jarvis, N. Groves, F. Capelastegui, et al. 2023. Comparative transmission of SARS-CoV-2 Omicron (B.1.1.529) and Delta (B.1.617.2) variants and the impact of vaccination: National cohort study, England. Epidemiol. Infect. 151:e58. doi: 10.1017/S0950268823000420.
- Boucau, J., C. Marino, J. Regan, R. Uddin, M. C. Choudhary, J. P. Flynn, G. Chen, A. M. Stuckwisch, J. Mathews, M. Y. Liew, et al. 2022. Duration of shedding of culturable virus in SARS-CoV-2 Omicron (BA.1) infection. N Engl. J. Med. 387 (3):275–277. doi: 10.1056/nejmc2202092.
- Callaway, E. 2022. Omicron sub-variant: What scientists know so far. Nature. 602 (7898):556–557. doi: 10.1038/d41586-022-00471-2.
- Cao, G., F. M. Blachere, W. G. Lindsley, J. D. Noti, and D. H. Beezhold. 2010. Development of a methodology to detect viable airborne virus using personal aerosol samplers. EPA/600/R-10/127, U.S. Environmental Protection Agency, Washington, DC.
- Centers for Disease Control. 2022. COVID data tracker variant proportions. Accessed 5 November 2022. https://covid.cdc.gov/covid-data-tracker/#variant-proportions.
- Cheng, V. C.-C., S.-C. Wong, V. W. M. Chan, S. Y. C. So, J. H. K. Chen, C. C. Y. Yip, K. H. Chan, H. Chu, T. W. H. Chung, S. Sridhar, et al. 2020. Air and environmental sampling for SARS-CoV-2 around hospitalized patients with coronavirus disease 2019 (COVID-19). Infect. Control Hosp. Epidemiol. 41 (11):1258–1265. doi: 10.1017/ice.2020.282.
- de Man, P., M. A. Ortiz, P. M. Bluyssen, S. J. de Man, M. J. Rentmeester, M. van der Vliet, E. J. Wils, and D. S. Y. Ong. 2022. Airborne SARS-CoV-2 in home and hospital environments investigated with a high-powered air sampler. J. Hosp. Infect. 119:126–131. doi: 10.1016/j.jhin.2021.10.018.
- Döhla, M., B. Schulte, G. Wilbring, B. M. Kümmerer, C. Döhla, E. Sib, E. Richter, P. F. Ottensmeyer, A. Haag, S. Engelhart, et al. 2022. SARS-CoV-2 in environmental samples of quarantined households. Viruses. 14 (5):1075. doi: 10.3390/v14051075.
- Fortin, A., M. Veillette, A. Larrotta, Y. Longtin, C. Duchaine, and N. Grandvaux. 2023. Detection of viable SARS-CoV-2 in retrospective analysis of aerosol samples collected from hospital rooms of patients with COVID-19. Clin. Microbiol. Infect. 29 (6):805–807. doi: 10.1016/j.cmi.2023.03.019.
- Hakki, S., J. Zhou, J. Jonnerby, A. Singanayagam, J. L. Barnett, K. J. Madon, A. Koycheva, C. Kelly, H. Houston, S. Nevin, et al. 2022. Onset and window of SARS-CoV-2 infectiousness and temporal correlation with symptom onset: A prospective, longitudinal, community cohort study. Lancet. Respir. Med. 10 (11):1061–1073. doi: 10.1016/S2213-2600(22)00226-0.
- Huang, L. 2022. Adjusted control rate closely associated with the epidemiologic evolution of the recent COVID-19 wave in Shanghai, with 94.3% of all new cases being asymptomatic on first diagnosis. J. Infect. 85 (4):e89–e91. doi: 10.1016/j.jinf.2022.07.010.
- Huang, L., X. Zhang, X. Zhang, Z. Wei, L. Zhang, J. Xu, P. Liang, Y. Xu, C. Zhang, and A. Xu. 2020. Rapid asymptomatic transmission of COVID-19 during the incubation period demonstrating strong infectivity in a cluster of youngsters aged 16-23 years outside Wuhan and characteristics of young patients with COVID-19: A prospective contact-tracing study. J. Infect. 80 (6):e1–e13. doi: 10.1016/j.jinf.2020.03.006.
- Kitagawa, H., T. Nomura, Y. Kaiki, M. Kakimoto, T. Nazmul, K. Omori, N. Shigemoto, T. Sakaguchi, and H. Ohge. 2023. Viable SARS-CoV-2 detected in the air of hospital rooms of patients with COVID-19 with an early infection. Int. J. Infect. Dis. 126:73–78. doi: 10.1016/j.ijid.2022.11.003.
- Lai, J., K. K. Coleman, S.-H. Sheldon Tai, J. German, F. Hong, B. Albert, Y. Esparza, A. K. Srikakulapu, M. Schanz, I. S. Maldonado, et al. 2023. Exhaled breath aerosol shedding of highly transmissible versus prior severe acute respiratory syndrome coronavirus 2 variants. Clin. Infect. Dis. 76 (5):786–794. doi: 10.1093/cid/ciac846.
- Lambrou, A. S., P. Shirk, M. K. Steele, P. Paul, C. R. Paden, B. Cadwell, H. E. Reese, Y. Aoki, N. Hassell, J. Caravas, et al. 2022. Genomic surveillance for SARS-CoV-2 variants: Predominance of the Delta (B.1.617.2) and Omicron (B.1.1.529) variants - United States, June 2021 - January 2022. MMWR Morb. Mortal. Wkly. Rep. 71 (6):206–211. doi: 10.15585/mmwr.mm7106a4.
- Landers, M., G. Barker, S. Wallentine, J. W. McWhorter, and C. Peel. 2003. A comparison of tidal volume, breathing frequency, and minute ventilation between two sitting postures in healthy adults. Physiother. Theory Pract. 19 (2):109–119. doi: 10.1080/09593980307958.
- Lednicky, J. A., M. Lauzard, Z. H. Fan, A. Jutla, T. B. Tilly, M. Gangwar, M. Usmani, S. N. Shankar, K. Mohamed, A. Eiguren-Fernandez, et al. 2020a. Viable SARS-CoV-2 in the air of a hospital room with COVID-19 patients. Int. J. Infect. Dis. 100:476–482. doi: 10.1016/j.ijid.2020.09.025.
- Lednicky, J., M. Lauzardo, M. M. Alam, M. Elbadry, C. Stephenson, J. C. Gibson, and J. G. Morris. 2021. Isolation of SARS-CoV-2 from the air in a car driven by a COVID patient with mild illness. Int. J. Infect. Dis. 108:212–216. doi: 10.1016/j.ijid.2021.04.063.
- Lednicky, J. A., S. N. Shankar, M. A. Elbadry, J. C. Gibson, M. M. Alam, C. J. Stephenson, A. Eiguren-Fernandez, J. G. Morris, C. N. Mavian, M. Salemi, et al. 2020b. Collection of SARS-CoV-2 virus from the air of a clinic within a university student health care center and analyses of the viral genomic sequence. Aerosol Air Qual. Res. 20 (6):1167–1171. doi: 10.4209/aaqr.2020.05.0202.
- Lind, M. L., A. J. Robertson, J. Silva, F. Warner, A. C. Coppi, N. Price, C. Duckwall, P. Sosensky, E. C. Di Giuseppe, R. Borg, et al. 2022. Association between primary or booster COVID-19 mRNA vaccination and Omicron lineage BA.1 SARS-CoV-2 infection in people with a prior SARS-CoV-2 infection: A test-negative case-control analysis. PLoS Med. 19 (12):e1004136. doi: 10.1371/journal.pmed.1004136.
- Linde, K. J., I. M. Wouters, J. A. J. W. Kluytmans, M. F. Q. Kluytmans-van den Bergh, S. D. Pas, C. H. GeurtsvanKessel, M. P. G. Koopmans, M. Meier, P. Meijer, C. R. Raben, et al. 2023. Detection of SARS-CoV-2 in air and on surfaces in rooms of infected nursing home residents. Ann. Work Expo. Health. 67 (1):129–140. doi: 10.1093/annweh/wxac056.
- Lindsley, W. G., R. C. Derk, J. P. Coyle, S. B. Martin, K. R. Mead, F. M. Blachere, D. H. Beezhold, J. T. Brooks, T. Boots, and J. D. Noti. 2021. Morbidity and mortality weekly report efficacy of portable air cleaners and masking for reducing indoor exposure to simulated exhaled SARS-CoV-2 aerosols-United States. MMWR Morb. Mortal. Wkly. Rep. 70 (27):972–976. doi: 10.15585/mmwr.mm7027e1.
- Lindsley, W. G., D. Schmechel, and B. T. Chen. 2006. A two-stage cyclone using microcentrifuge tubes for personal bioaerosol sampling. J. Environ. Monit. 8 (11):1136–1142. doi: 10.1039/b609083d.
- Liu, J., J. Liu, Z. He, Z. Yang, J. Yuan, H. Wu, P. Zhu, X. Fu, Y. Lin, Y. Zhang, et al. 2021. Duration of SARS-CoV-2 positive in quarantine room environments: A perspective analysis. Int. J. Infect. Dis. 105:68–74. doi: 10.1016/j.ijid.2021.02.025.
- Myers, N. T., R. J. Laumbach, K. G. Black, P. Ohman-Strickland, S. Alimokhtari, A. Legard, A. De Resende, L. Calderón, F. T. Lu, G. Mainelis, et al. 2022. Portable air cleaners and residential exposure to SARS-CoV-2 aerosols: A real-world study. Indoor Air. 32 (4):e13029. doi: 10.1111/ina.13029.
- Nannu Shankar, S., C. T. Witanachchi, A. F. Morea, J. A. Lednicky, J. C. Loeb, M. M. Alam, Z. H. Fan, A. Eiguren-Fernandez, and C. Y. Wu. 2022. SARS-CoV-2 in residential rooms of two self-isolating persons with COVID-19. J. Aerosol Sci. 159:105870. doi: 10.1016/j.jaerosci.2021.105870.
- NCBI. 2022. NCBI SARS-CoV-2 Data Hub. ncbi.nlm.nih.gov. Accessed 5 November 2022. https://www.ncbi.nlm.nih.gov/labs/virus/vssi/#/virus?SeqType_s=Nucleotide&VirusLineage_ss=Severe%20acute%20respiratory%20syndrome%20coronavirus%202,%20taxid:2697049&utm_source=data-hub&CollectionDate_dr=2022-01-01T00:00:00.00Z%20TO%202022-05-31T23:59:59.00Z&Region_s=North%20America.
- Pan, M., T. S. Bonny, J. Loeb, X. Jiang, J. A. Lednicky, A. Eiguren-Fernandez, S. Hering, Z. H. Fan, and C.-Y. Wu. 2017. Collection of viable aerosolized influenza virus and other respiratory viruses in a student health care center through water-based condensation growth. mSphere. 2 (5):e00251–17. doi: 10.1128/msphere.
- Pan, M., J. A. Lednicky, and C. Y. Wu. 2019. Collection, particle sizing and detection of airborne viruses. J. Appl. Microbiol. 127 (6):1596–1611. doi: 10.1111/jam.14278.
- Qian, H., T. Miao, L. Liu, X. Zheng, D. Luo, and Y. Li. 2021. Indoor transmission of SARS‐CoV‐2. Indoor Air. 31 (3):639–645. doi: 10.1111/ina.12766.
- Rahman, M. Z., M. E. Hoque, M. R. Alam, M. A. Rouf, S. I. Khan, H. Xu, and S. Ramakrishna. 2022. Face masks to combat coronavirus (COVID-19)—Processing, roles, requirements, efficacy, risk and sustainability. Polymers (Basel). 14 (7):1296. doi: 10.3390/polym14071296.
- Ramuta, M. D., C. M. Newman, S. F. Brakefield, M. R. Stauss, R. W. Wiseman, A. Kita-Yarbro, E. J. O'Connor, N. Dahal, A. Lim, K. P. Poulsen, et al. 2022. SARS-CoV-2 and other respiratory pathogens are detected in continuous air samples from congregate settings. Nat. Commun. 13 (1):4717. doi: 10.1038/s41467-022-32406-w.
- Raynor, P. C., A. Adesina, H. A. Aboubakr, M. Yang, M. Torremorell, and S. M. Goyal. 2021. Comparison of samplers collecting airborne influenza viruses: 1. Primarily impingers and cyclones. PLoS One. 16 (1):e0244977. doi: 10.1371/journal.pone.0244977.
- Ren, S.-Y., W.-B. Wang, R.-D. Gao, and A.-M. Zhou. 2022. Omicron variant (B.1.1.529) of SARS-CoV-2: Mutation, infectivity, transmission, and vaccine resistance. World J. Clin. Cases. 10 (1):1–11. doi: 10.12998/wjcc.v10.i1.1.
- Santarpia, J. L., V. L. Herrera, D. N. Rivera, S. Ratnesar-Shumate, S. Reid, D. N. Ackerman, P. W. Denton, J. W. S. Martens, Y. Fang, N. Conoan, et al. 2022. The size and culturability of patient-generated SARS-CoV-2 aerosol. J. Expo. Sci. Environ. Epidemiol. 32 (5):706–711. doi: 10.1038/s41370-021-00376-8.
- Torjesen, I. 2021. Covid-19: Omicron may be more transmissible than other variants and partly resistant to existing vaccines, scientists fear. BMJ. 375:n2943. doi: 10.1136/bmj.n2943.
- Vass, W. B., J. A. Lednicky, S. N. Shankar, Z. H. Fan, A. Eiguren-Fernandez, and C. Y. Wu. 2022. Viable SARS-CoV-2 Delta variant detected in aerosols in a residential setting with a self-isolating college student with COVID-19. J. Aerosol Sci. 165:106038. doi: 10.1016/j.jaerosci.2022.106038.
- Vogel, L. 2023. What to know about Omicron XBB.1.5. CMAJ. 195 (3):E127–E128. doi: 10.1503/cmaj.1096034.
- Wang, Z., W. Ma, X. Zheng, G. Wu, and R. Zhang. 2020. Household transmission of SARS-CoV-2. J. Infect. 81 (1):179–182. doi: 10.1016/j.jinf.2020.03.040.
- Wang, C. C., K. A. Prather, J. Sznitman, J. L. Jimenez, S. S. Lakdawala, Z. Tufekci, and L. C. Marr. 2021. Airborne transmission of respiratory viruses. Science 373 (6558):eabd9149. doi: 10.1126/science.abd9149.
- WHO. 2022. ONE Year Since the Emergence of COVID-19 Virus Variant Omicron. Accessed 8 July 2023. https://www.who.int/news-room/feature-stories/detail/one-year-since-the-emergence-of-omicron.
- Wong, S.-C., A. Ka-Wing Au, H. Chen, L. Lai-Ha Yuen, X. Li, D. Christopher Lung, A. Wing-Ho Chu, J. Daniel Ip, W.-M. Chan, H.-W. Tsoi, et al. 2022. Transmission of omicron (B.1.1.529) – SARS-CoV-2 variant of concern in a designated quarantine hotel for travelers: A challenge of elimination strategy of COVID-19. Lancet Reg. Health West Pac. 18:100360. doi: 10.1016/j.lanwpc.2021.100360.