Abstract
This study was designed to demonstrate a differential tumour response to radiation given as a large single dose or fractionated, and to investigate the postulated role of the tumour vasculature as a target for this difference. A C3H mammary carcinoma grown in the right rear foot of female CDF1 mice was used when at 200 mm3. Radiation (240 kV x-rays) was given locally to the tumour bearing foot either as 1×20 Gy or 10×2 Gy (2 fractions/day). Tumour response was assessed by calculating tumour growth time (TGT; time to grow to 3 times treatment volume). Vascular effects were monitored by performing dynamic contrast enhanced-magnetic resonance imaging using a 3-Tesla magnet and the contrast agent gadolinium (Gd)-DTPA. The endpoint was the initial area under the concentration curve (IAUC) following Gd-DTPA. The mean (±1 S.E.) TGT for control mice was 4.0 days (±0.2). This was significantly (Student's t-test; p < 0.05) increased to 12.9 (±0.6) and 19.8 (±0.7) days, by 10×2 and 1×20 Gy, respectively. The mean (±1 S.E.) of the median IAUC values for control tumours was 8.4 mMs (±0.5). This was non-significantly increased to 9.4 mMs (±0.4) 6-hours after 1×20 Gy, but then significantly decreased to a nadir of 6.8 mMs (±0.5) after 48-hours. IAUC recovered at longer time intervals. With 10×2 Gy, IAUC significantly decreased during irradiation, reaching 6.7 (±0.3) and 5.6 (±0.3) mMs at the mid-point and end of the irradiation period, respectively. IAUC recovered 24-hours after irradiating before significantly decreasing to 4.4 mMs (±0.3) at 48-hours. Our results confirm that radiation given in a large single dose is superior to the same dose given in a more conventional fractionated schedule, but vascular-mediated effects did not account for this difference in radiation sensitivity.
There is a valid biological rationale why the use of conventional fractionated schedules has been the dominant factor in the curative use of radiation therapy for many decades Citation[1]. Although schedules involving large doses per fraction are likely to be more effective against tumours, they have never been popular because of the concerns of late normal tissue toxicity resulting in a lower therapeutic ratio Citation[2]. However, improved physical targeting should improve this therapeutic ratio and as such larger radiation dose schedules are being evaluated in a variety of lesions Citation[3].
Although the target for radiation has always been considered to be the tumour clonogens Citation[4], Citation[5], recent pre-clinical evidence suggests that the tumour vasculature may play a major role in determining sensitivity, through the induction of apoptosis in the tumour endothelial cells Citation[6]. This response was radiation-dose dependent, with larger doses having the greatest effect Citation[6]. There is also clinical data supporting a vascular component with such radiation schedules. Using low-grade astrocytomas it was shown that the more angiogenic a tumour the higher the risk for early local recurrence after stereotactic radiation therapy Citation[7]. Additional studies with angiographically occult vascular malformations indicate that the use of stereotactic approaches may reduce the risk of symptomatic bleeding post treatment, presumably due to radiation induced vascular obliteration Citation[8].
The aim of this study was to use our well established C3H mouse mammary carcinoma model, to demonstrate that radiation given as a large single dose was superior to the same dose given in a fractionated schedule. DCE-MRI was then used to investigate the possible role that the tumour vasculature plays in influencing this difference.
Materials and methods
Animal and tumour model
A C3H mammary carcinoma grown in the right rear foot of 10–14 week-old female CDF1 mice was used in all experiments. Details of the derivation and maintenance of this tumour has been previously described Citation[9]. New tumour material was taken from frozen stock every three months and transplanted on the flanks of host animals. To produce experimental tumours large flank tumours were excised, finely minced with scissors and 5–10 µl subcutaneously injected in the foot. Treatments were performed when tumours had reached about 200 mm3 in size, which generally occurred within 12–24 days after implantation. Tumour volume was estimated from the formula: D1×D2×D3×π/6, where the D values represent the three orthogonal diameters. All experiments were performed under National and European approved guidelines for animal welfare.
Radiation treatments
The tumour-bearing feet of mice were locally irradiated using a conventional therapeutic x-ray machine (240 kV, 15 mA, 2-mm Al filter, 1.1 mm Cu half-value layer, dose-rate of 2.3 Gy/min). Animals were non-anaesthetised and restrained in a Lucite jig during the treatment. Their tumour-bearing feet were exposed and loosely attached to the jig with tape so as not to impair the blood supply to the foot, and immersed in a water bath at 25°C to ensure homogeneity of the radiation dose. The remainder of each animal's body was shielded by 1-cm thick lead. Radiation treatments were either 1×20 Gy or 10×2 Gy; the latter given as 2 fractions/day, separated by a 6-hour interval, for 5 consecutive days. Tumour response to irradiation was assessed using a tumour growth delay assay. This involved measuring tumour volume daily as described previously, and then calculating the tumour growth time (TGT; the time to regrow to 3 times the treatment volume).
Magnetic resonance imaging
Dynamic contrast enhanced imaging (DCE-MRI) was performed using a 3.0 T MR scanner (Signa Excite HD, General Electric, Milwaukee, WI). The mice were restrained in lucite jigs and their tumour-bearing legs exposed, as already described. A cannula attached to a 0.38 mm inner diameter line of 80 cm in length was connected to a syringe primed with contrast agent solution, and then inserted intravenously (i.v.) into the tail vein of the mice and held in position using tape. Two mice were simultaneously positioned in an upper extremity quadrature coil (Mayo Clinic BC-10 3.0 T). Images for T1-mapping were acquired using a spiral readout inversion recovery sequence with FOV = 10×10 or 8×8 cm, slice thickness = 2 mm, matrix size = 128×128, number of interleaves = 32, TR = 4000 ms, TI = 50; 250; 450; 650; 850; 1050;1250;1450 ms or 25; 450; 850; 1250;1650; 2050; 2450; 2850 ms, and TE = 13 ms. The dynamic images were acquired using a fast spoiled gradient echo sequence with FOV = 4×4 or 5×5 cm, slice thickness = 2 mm, matrix size = 128×128, or 128×120 reconstructed to 128×128, number of averages = 4 or 8, flip angle = 15, 30, or 35°, TR = 6.4, 6.5, 9.3, or 13.1 ms, and TE = 2.6, 4.1, or 4.3 ms. Each used parameter combination gave a time resolution of 4.8 to 5.2 s for the dynamic images. Gd-DTPA (Magnevist, Schering, Berlin, Germany) was diluted in heparinised saline to a concentration of 0.02 mmol/ml, and then during the initial 4 s of the 6th or 7th image acquisition it was i.v. injected at a dose of 0.1 mmol/kg. For each tumour, a region of interest (ROI) containing the whole tumour was drawn by hand for the data analysis. The inversion recovery sequence images were co-registered to the dynamic images and used for calculation of a T1 map. A linear relationship between Gd-DTPA concentration and ΔR1 (R1=1/T1) with a relaxivity r1=3300 l/(s mol) was assumed Citation[10]. It was further assumed that Gd-DTPA affected the relaxation rate of all tissue water molecules (so-called fast water exchange: water moves freely over vascular and cellular boundaries during the timescale of the image acquisition). Signal intensity was related to R1 by the signal equation for the T1-weighted fast spoiled gradient echo sequence for pixel-by-pixel calculation of Gd-DTPA concentration at each time point. Pixel concentration-time curves were used for calculation of the semiquantitative parameter initial area under the curve (IAUC) by trapezoidal integration of the first 90 s post administration of Gd-DTPA.
Data analysis
For the TGT data mean values (±1 S.E.) were calculated, while for the IAUC median values were obtained and means (±1 S.E.) were determined. With both endpoints statistical analysis was performed using a Student's t-test, with the significance level being p = 0.05.
Results
The effect of the different treatments on the growth of this C3H mouse mammary carcinoma, are illustrated in . Untreated control tumours showed exponential growth with a TGT of 4.0 days (3.8–4.2). Irradiating tumours with 1×20 Gy significantly delayed the growth of this tumour model resulting in a TGT of 19.8 days (19.1–20.5). A significant growth inhibition was also obtained with the 10×2 Gy radiation treatment, but the effect was significantly less than that seen with 1×20 Gy; the TGT being 12.9 days (12.3–13.5).
Figure 1. The effect of radiation on the growth of a C3H mouse mammary carcinoma. Tumour volume (left panel) was measured on a daily basis from the start of treatment in mice given either no treatment (○), 1×20 Gy (Δ), or 10×2 Gy (•). The TGT calculated from these growth curves are summarized (right panel). All results show means (±1 S.E.) from 8–10 mice.
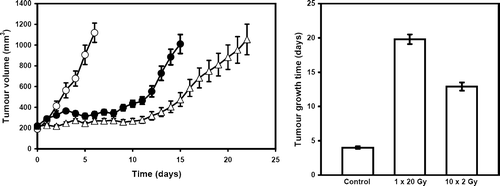
shows representative examples of the IAUC maps obtained at one particular time point. In these examples measurements were made 48-hours after either giving 1×20 Gy, or the final radiation treatment in the 10×2 Gy schedule. The actual IAUC values are summarized in , along with other time intervals. Following treatment with 1×20 Gy time-dependent changes in IAUC were observed. During the first 6-hours after irradiation there was a non-significant increase in IAUC, but after this time period IAUC decreased with a maximal reduction at 48-hours. The values at 48-hours was significantly lower than that measured at 6-hours, but did not reach significance when compared to pre-treatment values (p = 0.065). At longer time intervals after irradiating IAUC recovered to the pre-treatment levels. With 10×2 Gy, IAUC continually decreased during the irradiation period, reaching significance after 5 fractions and was even lower at 9 fractions. Within 24-hours after completing the fractionated radiation schedule IAUC had recovered to a level not significantly different from that seen at pre-treatment. However, by 48-hours IAUC had again significantly decreased to the lowest value measured. In , the IAUC values obtained 48-hours after irradiating with 1×20 Gy and 10×2 Gy are compared, and demonstrate that at this time the IAUC measured after 10×2 Gy was significantly lower than that found with 1×20 Gy.
Figure 2. Representative IAUC maps from differently treated C3H mouse mammary carcinomas. Maps are for either untreated tumours or 48-hours following local irradiation with 1×20 Gy or 10×2 Gy. Each pixel in the figure represents the IAUC measured over the first 90 s post injection of Gd-DTPA.
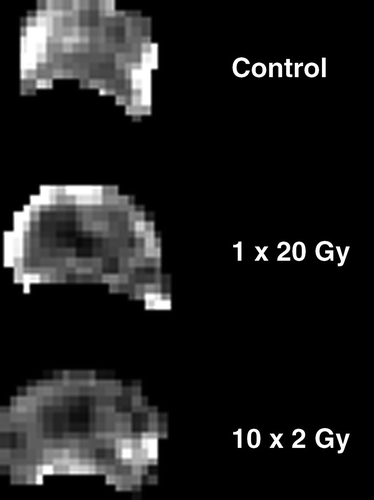
Figure 3. The effect of radiation on the uptake of Gd-DTPA. Tumours were irradiated with 1×20 Gy or 10×2 Gy and the IAUC for Gd-DTPA uptake measured either before, during, or 48–120 hours after irradiating (left panel). Arrows indicate the time of irradiation with 1×20 Gy (open arrow) or 10×2 Gy (closed arrows). Different animals were used for each time point, except the 3 + 6 hour values. The IAUC values 48-hours after giving either 1×20 Gy or 10×2 Gy are also compared (right panel). All results are means (±1 S.E.) of the median values from 5–10 mice.
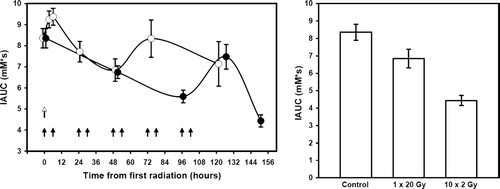
Discussion
Irradiating C3H mouse mammary carcinomas with a large single dose of 1×20 Gy significantly inhibited tumour growth. A significant growth delay was also seen when the same total dose was given, but in a fractionated schedule of 10×2 Gy. However, fractionating the radiation treatment was less effective than the large single dose. Historically, the target for radiation was always considered to be the tumour clonogens Citation[4], Citation[5], and the differences in sensitivity between the two radiation schedules primarily attributed to recovery from the radiation damage induced in these clonogenic tumour cells Citation[11]. However, recent studies have suggested that the tumour vasculature may play an important role in influencing response to radiation through the induction of endothelial cell apoptosis Citation[6]. It has been well established that the successful growth and development of any solid tumour require the development of a functional vascular supply Citation[12], Citation[13], and it has also been demonstrated that damaging this tumour vascular supply using various vascular disrupting agents (VDAs) can inhibit tumour growth Citation[14]. Additional evidence that radiation can induce a vascular mediated effect comes from the well known tumour bed effect phenomenum, in which tumour growth can be delayed by implanting tumours into an area that had previously been irradiated Citation[15], Citation[16]. But, this tumour bed effect is achieved with large doses. Moreover, the induction of endothelial cell apoptosis was only seen at relatively large single doses Citation[6], which has lead to criticism about the role of the vasculature as a target for radiation damage when using a more conventional fractionated schedule Citation[17], Citation[18].
The superiority of the 1×20 Gy in influencing tumour response could be due to this radiation treatment inducing vascular damage that is not apparent with the less effective 10×2 Gy. In this study, we used DCE-MRI to monitor possible vascular changes induced by radiation, the endpoint being the IAUC following injection of Gd-DTPA. This approach was utilized because a recent publication suggested this as the optimal method for monitoring the effects of VDAs Citation[19]. Following a single radiation treatment of 1×20 Gy there was a transient increase in IAUC within the first 6-hours, which could be indicative of an increase in radiation-induced vessel permeability. However, at longer time intervals IAUC decreased, reaching a nadir 48-hours after giving the radiation. Studies with VDAs also demonstrate that as a result of an effect on tumour endothelium there is an increase in vessel permeability followed by vascular collapse presumably due to the resulting increase in interstitial fluid pressure Citation[20], Citation[21]. Thus, the data is consistent with some form of vascular effect. However, what was surprising was that the IAUC also decreased during the fractionated radiation schedule, and this effect was even larger than that seen with 1×20 Gy. Moreover, 24-hours after completion of the fractionated irradiation there was complete recovery, followed at 48-hours by an even greater decrease in IAUC. If the tumour response to radiation was mediated through the vasculature then one would have expected the 10×2 Gy to have a larger tumour growth inhibition than 1×20 Gy, and this is clearly not the case. This suggests that our IAUC results do not entirely reflect vascular mediated effects. Besides the semi-quantitative IAUC, quantitative model parameters (e.g., Ktrans) could be performed, and additional studies using contrast agents of different sizes (e.g., USPIO agents) might help shed light on this issue. Both approaches have been used to show vascular changes following treatment with vascular modifiers Citation[22–24]. Another factor that argues against the vasculature being the major target for radiation comes from the observation that VDAs which are very effective at inducing vascular damage actually have very little effect on tumour growth and significant inhibition is only seen when such drugs are combined with treatments that target the tumour cells Citation[25].
Overall, our study shows some clear time-dependent changes in IAUC during and after irradiation, but the degree of change is totally inconsistent with the anti-tumour efficacy of the radiation dose given. This suggests that our data do not appear to support the concept of the vasculature being a major target for radiation damage. The most likely explanation for the difference in response of this C3H mammary carcinoma to 1×20 Gy and 10×2 Gy is an effect mediated through the tumour clonogenic cell population.
The authors would like to thank Ms. Inger Marie Horsman, Ms. Pia Schjerbeck and Ms. Dorthe Grand for excellent technical assistance. Financial support was obtained from the Danish Cancer Society. Presented at the Third Acta Oncologica Symposium: “Stereotactic Body Radiotherapy” June 15–17, 2006, Copenhagen, Denmark.
References
- Fletcher GH. Regaud Lecture: Perspectives on the history of radiotherapy. Radiother Oncol 1988; 12: 253–71
- Baumann M, Saunders MI, Joiner MC. Modified fractionation. In: Steel GG, editor. Basic Clinical Radiobiology. 3rd ed. London: Arnold; p 147–57.
- Solberg TD, Selch MT, Smathers JB, DeSalles AAF. Fractionated stereotactic radiotherapy: Rationale and methods. Med Dos 1998; 23: 209–19
- Trott KR. Tumour stem cells: The biological concept and its application in cancer treatment. Radiother Oncol 1994; 30: 1–5
- Hendry JH, West CML, Moore JV, Potten CS. Tumour stem cells: The relevance of predictive assays for tumour control after radiotherapy. Radiother Oncol 1994; 30: 11–6
- Garcia-Barros M, Paris F, Cordon-Cardo C, Lyden D, Rafii S, Haimovitz-Friedman A, et al. Tumor response to radiotherapy regulated by endothelial cell apoptosis. Science 2003; 300: 1155–9
- Fuss M, Wenz F, Essig M, Muenter M, Debus J, Herman TS, et al. Tumor angiogenesis of low-grade astrocytomas measured by dynamic susceptibility contrast-enhanced MRI (DSC-MRI) is predictive of local tumor control after radiation therapy. Int J Radiat Oncol Biol Phys 2001; 51: 478–82
- Tsien C, Souhami L, Sadikot A, Olivier A, Del Carpio-O'Donovan R, Corns R, et al. Stereotactic radiosurgery in the management of angiographically occult vascular malformations. Int J Radiat Oncol Biol Phys 2001; 50: 133–8
- Overgaard J. Simultaneous and sequential hyperthermia and radiation treatment of an experimental tumor and its surrounding normal tissue in vivo. Int J Radiat Oncol Biol Phys 1980; 6: 1507–17
- Pintaske J, Martirosian P, Graf H, Erb G, Lodemann KP, Claussen CD, et al. Relaxivity of Gadopentetate Dimeglumine (Magnevist), Gadobutrol (Gadovist), and Gadobenate Dimeglumine (MultiHance) in human blood plasma at 0.2, 1.5, and 3 Tesla. Invest Radiol 2006; 41: 213–21
- Steel GG. Cell survival as a determinant of tumour response. In: Steel GG, editor. Basic Clinical Radiobiology. 3rd ed. London: Arnold; p 52–63.
- Folkman J. How is blood vessel growth regulated in normal and neoplastic tissue?. Cancer Res 1986; 46: 467–73
- Hahnfeldt P, Panigrahy D, Folkman J, Hlatky L. Tumor development under angiogenic signaling: A dynamic theory of tumor growth, treatment response, and postvascular dormancy. Cancer Res 1999; 59: 4770–5
- Siemann DW, Bibby MC, Dark GG, Dicker AP, Eskens FALM, Horsman MR, et al. Differentiation and definition of vascular-targeted therapies. Clin Cancer Res 2005; 11: 416–20
- Stenstrom KW, Vermund H, Mosser DG, Marvin JF. Effects of roentgen irradiation on the tumor bed. I. The inhibiting action of local pretransplantation roentgen irradiation (1500 ra) on the growth of mouse mammary carcinoma. Radiat Res 1955; 2: 180–91
- Hewitt HB, Blake ER. The growth of transplanted murine tumours in pre-irradiated sites. Br J Cancer 1968; 22: 808–24
- Suit HD, Willers H. Comment on “tumor response to radiotherapy regulated by endothelial cell apoptosis” (I). Science 2003; 302: 1894
- Brown M. Comment on “tumor response to radiotherapy regulated by endothelial cell apoptosis” (II). Science 2003; 302: 1894
- Leach MO, Brindle KM, Evelhoch JL, Griffiths JR, Horsman MR, Jackson A, et al. The assessment of antiangiogenic and antivascular therapies in early-stage clinical trials using magnetic resonance imaging: Issues and recommendations. Br J Cancer 2005; 92: 1599–610
- Tozer GM, Prise VE, Wilson J, Cemazar M, Shan S, Dewhirst MW, et al. Mechanisms associated with tumor vascular shut-down induced by combretastatin A-4 phosphate: Intravital microscopy and measurement of vascular permeability. Cancer Res 2001; 61: 6413–22
- Baguley BC, Ching L-M. DMXAA: An antivascular agent with multiple host responses. Int J Radiat Oncol Biol Phys 2002; 54: 1503–11
- de Lussanet QG, Backes WH, Griffioen AW, van Engelshoven JMA, Beets-Tan RGH. Gadopentate dimeglumine versus ultrasmall super-paramagnetic iron oxide for dynamic contrast-enhanced MR imaging of tumor angiogenesis in human colon carcinoma in mice. Radiol 2003; 229: 429–38
- Turetschek K, Preda A, Novikov V, Brasch RC, Weinmann HJ, Wunderbaldinger P, et al. Tumor microvascular changes in antiangiogenic treatment:assessment by magnetic resonance contrast media of different molecular weights. J Magn Reson Imaging 2004; 20: 138–44
- Bentzen L, Vestergaard-Poulsen P, Nielsen T, Overgaard J, Bjørnerud A, Briley-Sæbø K, et al. Intravascular contrast agent-enhanced MRI measuring contrast clearance and tumor blood volume and the effects of vascular modifiers in an experimental tumor. Int J Radiat Oncol Biol Phys. 2005; 61: 1208–15
- Horsman MR, Siemann DW. Pathophysiological effects of vascular targeting agents and the implications for combination with conventional therapies. Cancer Res 2006; ( in press).