Abstract
Background: Gene electrotrotransfer describes the use of electric pulses to transfer DNA to cells. Particularly skeletal muscle has potential for systemic secretion of therapeutic proteins. Gene electrotransfer to muscle using the integrin inhibitor plasmid AMEP (Antiangiogenic MEtargidin Peptide) was investigated in a phase I dose escalation study. Primary objective was safety.
Material and methods: Patients with metastatic or locally advanced solid tumors, without further standard treatments available, were treated with once-only gene electrotransfer of plasmid AMEP to the femoral muscle. Safety was monitored by adverse events registration, visual analog scale (VAS) after procedure and magnetic resonance imaging (MRI) of treated muscles. Pharmacokinetics of plasmid AMEP in plasma and urine was determined by quantitative polymerase chain reaction. Response was evaluated by positron emission tomography–computed tomography (PET–CT) scans.
Results: Seven patients were enrolled and treated at dose levels from 50 to 250 μg of plasmid AMEP, the study was terminated early due to cessation of plasmid production. Minimal systemic toxicity was observed and only transient mild pain was associated with the delivery of the electric pulses. MRI of the treated muscles revealed discrete intramuscular edema 24 h after treatment. The changes in the muscle tissue resolved within 2 weeks after treatment. Peak concentrations of plasmid AMEP was detected only in plasma within the first 24 hours after injection. Protein AMEP could not be detected, which could be due to the limit of detection. No objective responses were seen.
Conclusions: Gene electrotransfer of plasmid AMEP was found to be safe and tolerable. No objective responses were observed but other DNA drugs may be tested in the future using this procedure.
Background
Gene therapy offers promise of entirely new treatment strategies in a number of diseases, including cancer. Therapeutic genes can be delivered to cells and tissues, rendering the transfected cells capable of producing proteins of therapeutic benefit [Citation1,Citation2]. Gene electrotransfer describes the use of electric pulses to facilitate transport of plasmid DNA into cells and tissues [Citation3]. This method can be applied to any accessible tissue, and numerous in vivo studies have shown that the technique significantly enhances gene transfer efficiency [Citation4]. An important feature of gene electrotransfer is that transfection only takes place in the area encompassed by the electrodes which allows control of what tissue areas are transfected [Citation5].
Skeletal muscle is an attractive target tissue for gene electrotransfer as muscle fibers have a high intrinsic capacity to produce and secrete proteins into the circulation leading to a systemic effect [Citation6–8]. Furthermore, gene electrotransfer to skeletal muscle is highly efficient, showing a 200-fold increase in gene expression compared to DNA injection alone [Citation9] as well as persistent expression for several months [Citation9]. Several studies in large animal models have demonstrated that DNA electrotransfer of a therapeutic gene to a limited muscle mass can lead to expression levels within therapeutic range of the transferred gene and moreover therapeutic benefits in the animals [Citation10,Citation11]. Thus, from a clinical perspective gene electrotransfer to muscle tissue may provide an opportunity for the patient to become his own medicine producer, as schematically shown in .
Figure 1. The patient as the medicine producer. The plasmid DNA is injected into the muscle tissue followed by application of electric pulses (illustrated as the red lightning). After electrotransfer, the plasmid DNA migrates to the cell nucleus and gene expression takes place with the formation of the transgenic protein (illustrated as small blue molecules). The transgenic protein is secreted into the systemic circulation and may act elsewhere in the body.
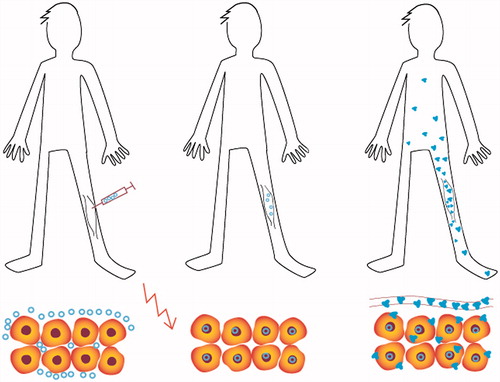
The contribution of angiogenesis in tumor progression is well-established. Integrins play a key role in the regulation of angiogenesis as integrins control endothelial cell adhesion, migration and survival [Citation12]. Both αvβ3 and α5β1 integrins are upregulated on endothelial cells during tumor angiogenesis [Citation13], and antagonists to these two integrins have been shown to block angiogenesis in vitro and in vivo [Citation13,Citation14]. Thus, integrin antagonists targeting αvβ3 and α5β1 integrins may inhibit tumor angiogenesis, and be beneficial in the treatment of patients with advanced solid tumors.
AMEP (Antiangiogenic MEtargidin Peptide) is the disintegrin domain of human metargidin (also known as ADAM-15). It is an anti-cancer agent with proven anti-angiogenic properties by binding of αvβ3 and α5β1 integrins on endothelial cells [Citation15–17]. Furthermore, intramuscular gene electrotransfer of plasmid AMEP has been shown to inhibit tumor growth in murine models [Citation17]. Recently, we reported the results from a first-in-man study investigating intratumoural gene electrotransfer of plasmid AMEP into cutaneous melanoma. Plasmid AMEP was well tolerated and measurements of treated lesions suggested local anti-tumor efficacy [Citation18]. In this study, AMEP mRNA was found in treated lesions; however, AMEP protein could not be detected with available methodology [Citation18].
Here, we report the results from a phase I study investigating gene electrotransfer to muscle tissue using plasmid AMEP in patients with advanced solid tumors. The primary objective of the study was evaluation of safety. Secondary objectives included investigation of the pharmacokinetic profile of plasmid AMEP, tolerability of the treatment procedure and clinical response.
Material and methods
Study design
The study was an investigator-initiated phase I dose escalation study. All patients were recruited and treated at Copenhagen University Hospital Herlev, Denmark. Regulatory approvals from the Danish Board of Health, the Regional Ethics Committee and the Danish Data Protection Agency were obtained. All patients signed written informed consent. The primary objective of the study was evaluation of the safety of intramuscular gene electrotransfer of plasmid AMEP. Secondary objectives included assessment of the anti-tumor efficacy of the investigational treatment, evaluation of pharmacokinetics for plasmid AMEP and protein AMEP, and patient experience of the treatment procedure.
Patients
Patients with histologically confirmed advanced solid tumors, without further standard treatment options available, were eligible for the study. Other inclusion criteria included: written informed consent, age ≥18 years, Eastern Cooperative Oncology Group (ECOG) performance status ≤1, life expectancy ≥3 months, measurable disease according to RECIST 1.1. Patients had to have adequate bone marrow, liver and renal function and patients of reproductive capacity had to use safe anti-contraceptives. Exclusion criteria included: pregnancy or lactation, anticancer treatment within the last 28 days, treatment with anticoagulant drugs, active infection, any implanted electronic device, acute myocardial infarction, stroke or acute ischemic event within the last 6 months, severe hypertension, severe atherosclerosis or significant arrhythmias.
Study plan
Four-dose levels were planned with three patients in each cohort. The dose levels were 50, 100, 250 and 500 μg of plasmid AMEP administered as a once-only treatment. Patients were treated once with intramuscular electrotransfer of plasmid AMEP at Day 1. As this was a first-in-man study using gene electrotransfer to muscle of this DNA drug, an independent safety committee reviewed the study data before each patient inclusion as well as before dose escalation. Dose-limiting toxicity was defined as any Grade 4 event according to the National Cancer Institute Common Terminology Criteria for Adverse Events (NCI CTC-AE) version 4.0. All patients were hospitalized for 24 h after treatment with the purpose of safety monitoring and pharmacokinetic sampling. Patients were monitored weekly until Day 29, and at Day 57. A combined fluoro-18-deoxyglucose (18F-FDG) positron emission tomography (PET) and contrast-enhanced computed tomography (CT) was performed at baseline, at Day 29, and Day 57.
Plasmid AMEP
Plasmid AMEP encodes the disintegrin domain of human metargidin (ADAM-15) under the control of the human cytomegalovirus immediate early enhancer/promoter CMV-intron A. The plasmid backbone was devoid of any antibiotic resistance gene and produced under Good Manufacturing Practice (GMP) standards by Eurogentec S.A. (Liege, Belgium). Before use, the plasmid was diluted in sterile water to the prescribed concentration and dispensed in an insulin syringe (29 G, length of needle 12.7 mm), prepared by the hospital pharmacy unit. Plasmid injection volume was 200 μl.
Treatment procedure
Treament was delivered to the femoral muscle according to the procedure illustrated and described in .
Figure 2. Treatment procedure. (A) A 6-cm long incision was marked on the thigh ∼10 cm above the patella. Local anesthesia (lidocaine 20 mg/ml with epinephrine 5 μg/ml) was injected subcutaneously. (B) Skin and subcutaneous tissue were incised to m. rectus femoris. Local anesthesia was then injected beneath the intact muscle fascia in the periphery of the exposed area. (C) A needle electrode array was placed directly on the exposed muscle fascia parallel to muscle fiber orientation. Plasmid AMEP was injected intramuscularly between the two rows in the needle array. Immediately after injection of plasmid AMEP the electrode was inserted into the muscle and a combination of one short high voltage pulse followed by one long low voltage pulse was applied. Electric pulses were delivered in less than a second. (D) After injection of plasmid DNA and delivery of the electric pulses, the subcutaneous tissue was closed with absorbable sutures and the skin closed with non-absorbable continuous suture. A compression bandage was applied for 24 h. The non-absorbable skin suture was removed 14 days after treatment. (E) Scar on the thigh 14 days after treatment.
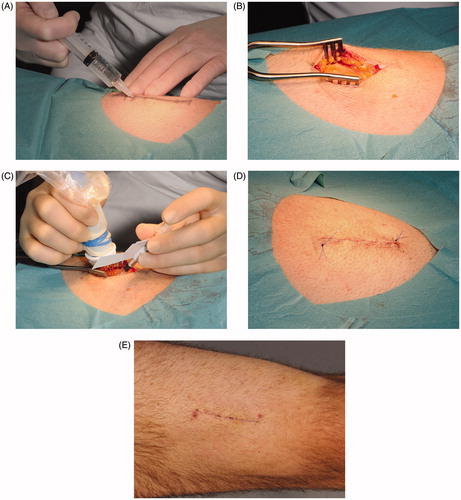
Based on our preclinical study, it was decided to use an open procedure in order to ensure exact co-localization of plasmid DNA and the electric field, and to use equipment approved for clinical use [Citation5]. Electric pulses were delivered using a square wave pulse generator (Cliniporator, IGEA, Carpi, Italy) and a needle electrode array with eight needles, four needles in each row with a 4-mm gap between the two rows (IGEA, Carpi, Italy). Pulse parameters consisted of one high voltage (applied voltage to electrode distance ratio 700 V/cm, 100 μs) followed by one long low voltage (80 V/cm, 400 ms) pulse with a lag of 1 s between the pulses, based on previous preclinical data [Citation19]. Patients were not prescribed any pre-medication for the procedure.
Assessment of safety and efficacy
Adverse events were recorded according to the NCI CTC-AE version 4.0. Full-blood count, clotting, and serum biochemistry including biochemical markers of muscle damage, creatinine phosphokinase (CPK) and lactate dehydrogenase (LDH), was measured over time. Electrocardiogram (ECG) was performed before and after treatment. Twenty-four hours (Day 2) and 2 weeks (Day 15) after treatment magnetic resonance imaging (MRI) of the treated thigh and the opposite untreated (control) extremity was performed using a 3T MRI system (Achieva, Philips Medical, Eindhoven, the Netherlands), see protocol details in Supplementary material. MRI scans were evaluated by a skilled radiologist. To evaluate patient experience of the study procedure, a visual analog scale (VAS) was used. The scale consists of a horizontal line, 10 cm in length, anchored by 0 and 10 (0 being ‘no pain’ and 10 being ‘worst pain imaginable’).
Combined 18F-FDG PET 60-min post-tracer injection and contrast-enhanced CT scan of the neck, thorax, abdomen and pelvis was performed at baseline, at Day 29 and Day 57. All examinations were performed with a Gemini PET/CT system (Philips Medical) according to standard procedure. Efficacy of the treatment was solely based on the CT scans using the Response Evaluation Criteria in Solid Tumours (RECIST) version 1.1. The PET images were evaluated using the PERCIST criteria [Citation20] and provided additional information on changes in target lesions for overall response. Specialists in respectively radiology and nuclear medicine performed analyses of CT and PET images.
Pharmacokinetics and protein AMEP measurements
Blood samples using EDTA tubes (9 ml/sampling) were collected before treatment, then 2, 6, 24 and 48 h after plasmid administration and at each follow-up visit up to Day 57 post-treatment. Samples were immediately centrifuged at 800 rpm, for 10 min at 4 °C, supernatants were collected and centrifuged at 1600 rpm, for 10 min at 4 °C. Plasma samples were stored in a temperature-logged freezer at −80 °C. Urine samples were collected at baseline, 2 and 24 h after plasmid administration and at each follow-up visit. Urine samples were centrifuged within 2 h at 1600 rpm, for 10 min at 4 °C and stored at −80 °C. Quantitative assessment of plasmid AMEP levels in plasma and urine was determined by quantitative polymerase chain reaction (QPCR) and quantification of protein AMEP in plasma samples by mass spectrometry. Protocols for bio-analyses are described in Supplemental material.
Statistical methods
All statistical analyses were descriptive.
Results
Patient characteristics
Patient enrollment began July 2012. Seven out of a total of 12 patients planned for the study were treated before the study terminated early 1 March 2014. The batch of plasmid AMEP expired at the end of February 2014 and production was discontinued by Onxeo (formerly BioAlliance, Paris, France). Patient characteristics are described in . Due to disease progression three patients went off study following evaluation at Day 29. All were referred to further experimental treatment (see ). One patient decided to opt for further experimental chemotherapy at Day 19, but agreed to continue evaluation of adverse events and pharmacokinetic sampling. Thus, all patients were evaluable for toxicity at Day 29. Two patients declined the MRI scan at Day 15.
Table 1. Patient characteristics at baseline.
Safety and tolerability of the treatment procedure
All patients received one intramuscular injection of plasmid AMEP followed by electric pulses as described in . No dose-limiting toxicity was observed. One related serious adverse event occurred (hospitalization for one day due to Grade 1 fever). Treatment-site toxicity was modest with one patient reporting a minor subcutaneous hematoma. No wound infections or signs of impaired healing occurred. Modest systemic toxicity was observed with only Grade 1 adverse events reported. No major changes in laboratory parameters were detected apart from transient increase in C-reactive protein (4/7 patients). All treatment-emergent adverse events are shown in . No allergic reactions, bleeding episodes or hypertension were seen. No ECG changes were observed.
Table 2. All treatment-emergent adverse events.
As expected, a brief contraction of the thigh muscle was observed during application of the electric pulses. The treatment procedure was well tolerated with a median VAS score of ≤2 at delivery of the electric pulses (range 0–7.0). Thirty minutes post treatment VAS had diminished to ≤1 (7/7 patients). None of the patients needed additional analgesics following treatment.
MRI
shows representative MR images from one patient. MR scans performed at Day 2 showed minimal intramuscular edema (7/7 patients) and discrete intramuscular bleeding (7/7 patients) in the area corresponding to the insertion of the needle electrode. The observed changes were no longer present on MR scans performed at Day 15 except for one patient where discrete edema was still visible.
Figure 3. MR images of untreated and treated muscles. Representative MR images of the untreated and treated muscle (patient no. 2) acquired Day 2 and Day 15 after treatment. Different MR sequences were used: T2-weighted (T2W) sequence to assess edema; T1-weighted (T1W) gradient-echo (GRE) isotropic sequence to detect acute bleeding and a T2*-weighted (T2*W) sequence to detect coagulated blood i.e. detection of remnants of previous bleeding. Minimal intramuscular edema and discrete intramuscular bleeding in the area corresponding to the insertion of the needle electrode is seen at Day 2. The changes are no longer present at Day 15.
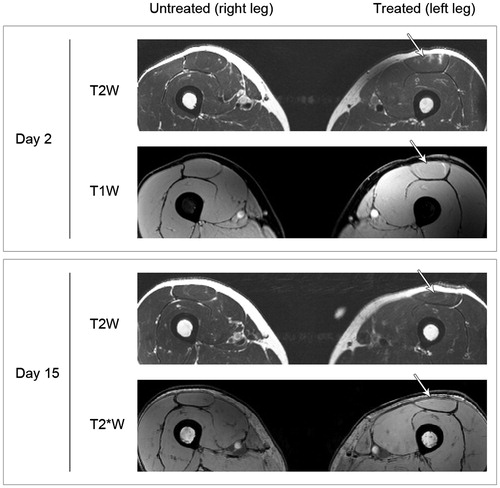
CPK and LDH measurements
An increase of CPK plasma levels of 2–8-fold was observed 24 h after treatment (5/7 patients). The increase in CPK was not above the upper limit of normal and had returned to baseline levels at Day 8. In contrast, the increase in CPK was above upper limit of normal (Grade 1) at Day 8 for patient no. 3 and returned to baseline levels at Day 15. No changes in plasma LDH levels were seen when comparing baseline values to samples collected post treatment.
Efficacy
Response evaluated according to RECIST 1.1 and PERCIST is shown in . No objective responses were seen. One patient had stable disease and stable metabolic disease at Day 57 but clinical progression.
Table 3. Response (RECIST 1.1 and PERCIST).
Pharmacokinetics and measurements of protein AMEP
shows the plasma levels of plasmid AMEP (copy numbers/10 μl plasma) for the seven patients. Mean peak concentration of plasmid AMEP was 7.3 × 103 copies/10 μl (range 4.0 × 102–1.4 × 105 copies/10 μl) corresponding to 0.005% (range 0.0005–0.09%) of injected plasmid AMEP copy numbers. Plasmid AMEP was not detected in urine at any time points in any of the seven patients (data not shown). There was no detectable protein AMEP in plasma samples (limit of quantification 25 ng/ml by mass spectrometry).
Figure 4. Plasma levels of plasmid AMEP (copy numbers) following treatment. Quantitative PCR was used to determine plasma levels of plasmid AMEP. Samples were collected before treatment, 2, 6 and 24 after plasmid administration and at each follow-up visit. Results from for the seven patients are shown. h: hours
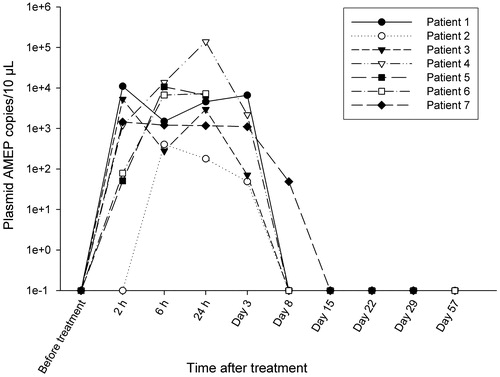
Discussion
DNA drugs offer a new way of delivering cancer therapy, where the patient actually becomes the medicines producer. As many new targets are discovered for cancer therapy, this approach may offer possibilities for production of a variety of DNA drugs, further enabling personalized medicine.
Successful intramuscular electrotransfer of therapeutic genes with systemic effects have been reported in many preclinical studies using large animal models [Citation10,Citation21,Citation22].
Safety and tolerability of gene electrotransfer to muscle
In this study, intramuscular electrotransfer of plasmid AMEP was well-tolerated. Patients reported transient mild pain at delivery of the electric pulses. MRI of treated muscles revealed minimal impact from the treatment procedure. Transient permeabilization of the cell membrane will lead to uptake of sodium, leading to transient edema [Citation23,Citation24]. Only transient increase in plasma CPK levels was seen. Indeed the combination of one high-voltage pulse followed by one low-voltage pulse has been shown to cause minimal perturbation of cell physiology, which is important for efficient transgene expression [Citation19,Citation25].
Pharmacokinetics
Following intramuscular electrotransfer of plasmid AMEP, the plasmid seemed to enter the circulation at early time points with peak concentration of plasmid AMEP being detected at the first or second sampling time (2 and 6 h, respectively) in 5/7 patients. This is in line with published data demonstrating that plasmid DNA is rapidly cleared and degraded following intramuscular electrotransfer [Citation26]. Also, the pharmacokinetic data suggest some inter-patient variability in plasmid AMEP concentrations after intramuscular injection similar to the results found in our recent study on intratumoural gene electrotransfer of plasmid AMEP into cutaneous melanoma. Dose dependency regarding plasmid AMEP found in plasma was not observed which is an interesting observation although the small size of the study population must be kept in mind.
Response
No objective responses were observed in the present study. This may be due to no or inadequate expression of plasmid AMEP in the muscle as no protein AMEP was detected in plasma using mass spectrometry (limit of quantification 25 ng/ml). It is of course possible that the muscle cells were damaged by the electric pulses. However, the pulsing technique and parameters used have previously been successfully tested in a large animal model [Citation5]. The available assay for protein AMEP may very well have had a detection limit too high to pick up secreted protein AMEP—indeed this was also a problem in a previous study [Citation18]. In the previous study using plasmid AMEP [Citation18], it was possible to investigate presence of mRNA, which was not done in this study as the target for the gene therapy was the femoral muscle. Finally, protein AMEP may have been produced and released from treated muscles but the targeted integrins may not have been present in the tumors. αvβ3 and α5β1 integrin expression was not tested in patient’s tumors as no validated test exists.
An optimal situation would be working with proteins for which validated and sensitive tests exist, as well as targeting molecules where presence in tumors may be confirmed by a validated assay. However, it must also be acknowledged that when using novel DNA drugs this ideal situation may not be present.
Successful gene electrotransfer depends both on the nature of the plasmid DNA and the dose. Plasmid AMEP contains the cytomegalovirus immediate-early promoter, which can drive high-level long-term expression in skeletal muscle [Citation9]. In the present study, low doses of plasmid DNA was tested. Increasing the amount of DNA may not necessarily lead to higher expression levels [Citation5,Citation8].
The present study indicates that gene electrotransfer to muscle using a surgical approach can be safely performed in patients. This encourages the development of novel plasmid DNA drugs for human use. Novel DNA drugs should have a validated method for detection of drug target as well as a method for detecting the target of the transgenic protein.
Conclusions
This study describes the results from a phase I trial evaluating intramuscular gene electrotransfer of plasmid AMEP. Modest adverse events have been reported including brief mild pain associated with the delivery of electric pulses. MRI of treated muscles 24 h post-treatment showed discrete tissue changes. No objective responses were observed.
Taken together, the results indicate that the gene electrotransfer procedure is safe and tolerable. Further studies using different DNA drugs are encouraged.
IONC_A_1315171-Supplementary_Information.docx
Download MS Word (13.8 KB)Acknowledgments
The authors particularly wish to thank Dr. Ulrik Lassen, Rigshospitalet, Dr. Ole Steen Nielsen, University of Aarhus, and Dr. Anders Jakobsen, Vejle Hospital all from Denmark for voluntarily serving on the safety committee for the study. The help of research nurses Eva Rønnengart, Karen Clement Petersen, Helle Jürs and technicians Vibeke Hintze Holm and Charlotte Falk from the Department of Oncology, is greatly appreciated. The authors acknowledge Hanne Michelsen and Knud Nelausen from the Clinical Research Unit at the Department of Oncology for setting up the clinical database. Thank you to photographer Anders Jaegenoe, Department of Plastic Surgery, for documentation of the treatment procedure.
Disclosure statement
Pierre Attali and Céline Bouquet are former employees of Onxeo and current Onxeo’s stockholders. All other authors report no conflicts of interest. The authors alone are responsible for the content and writing of this article.
Additional information
Funding
References
- Yin H, Kanasty RL, Eltoukhy AA, et al. Non-viral vectors for gene-based therapy. Nat Rev Genet. 2014;15:541–555.
- Daud AI, DeConti RC, Andrews S, et al. Phase I trial of interleukin-12 plasmid electroporation in patients with metastatic melanoma. J Clin Oncol. 2008;26:5896–5903.
- Neumann E, Schaefer-Ridder M, Wang Y, et al. Gene transfer into mouse lyoma cells by electroporation in high electric fields. EMBO J. 1982;1:841–845.
- Mir LM, Moller PH, Andre F, et al. Electric pulse-mediated gene delivery to various animal tissues. Adv Genet. 2005;54:83–114.
- Spanggaard I, Corydon T, Hojman P, et al. Spatial distribution of transgenic protein after gene electrotransfer to porcine muscle. Hum Gene Ther Methods. 2012;23:387–392.
- Lu QL, Bou-Gharios G, Partridge TA. Non-viral gene delivery in skeletal muscle: a protein factory. Gene Ther. 2003;10:131–142.
- Pedersen BK, Febbraio MA. Muscles, exercise and obesity: skeletal muscle as a secretory organ. Nat Rev Endocrinol. 2012;8:457–465.
- Hojman P, Gissel H, Gehl J. Sensitive and precise regulation of haemoglobin after gene transfer of erythropoietin to muscle tissue using electroporation. Gene Ther. 2007;14:950–959.
- Mir LM, Bureau MF, Gehl J, et al. High-efficiency gene transfer into skeletal muscle mediated by electric pulses. Proc Natl Acad Sci USA. 1999;96:4262–4267.
- Draghia-Akli R, Ellis KM, Hill LA, et al. High-efficiency growth hormone-releasing hormone plasmid vector administration into skeletal muscle mediated by electroporation in pigs. FASEB J. 2003;17:526–528.
- Pavlin D, Tozon N, Sersa G, et al. Efficient electrotransfection into canine muscle. Technol Cancer Res Treat. 2008;7:45–54.
- Cheresh DA, Stupack DG. Regulation of angiogenesis: apoptotic cues from the ECM. Oncogene. 2008;27:6285–6298.
- Kim S, Bell K, Mousa SA, et al. Regulation of angiogenesis in vivo by ligation of integrin alpha5beta1 with the central cell-binding domain of fibronectin. Am J Pathol. 2000;156:1345–1362.
- Brooks PC, Montgomery AM, Rosenfeld M, et al. Integrin alpha v beta 3 antagonists promote tumor regression by inducing apoptosis of angiogenic blood vessels. Cell. 1994;79:1157–1164.
- Bosnjak M, Dolinsek T, Cemazar M, et al. Gene electrotransfer of plasmid AMEP, an integrin-targeted therapy, has antitumor and antiangiogenic action in murine B16 melanoma. Gene Ther. 2015;22:578–590.
- Bosnjak M, Prosen L, Dolinsek T, et al. Biological properties of melanoma and endothelial cells after plasmid AMEP gene electrotransfer depend on integrin quantity on cells. J Membrane Biol. 2013;246:803–819.
- Trochon-Joseph V, Martel-Renoir D, Mir LM, et al. Evidence of antiangiogenic and antimetastatic activities of the recombinant disintegrin domain of metargidin. Cancer Res. 2004;64:2062–2069.
- Spanggaard I, Snoj M, Cavalcanti A, et al. Gene electrotransfer of plasmid antiangiogenic metargidin peptide (AMEP) in disseminated melanoma: safety and efficacy results of a phase I first-in-man study. Hum Gene Ther Clin Dev. 2013;24:99–107.
- Hojman P, Gissel H, Andre FM, et al. Physiological effects of high- and low-voltage pulse combinations for gene electrotransfer in muscle. Hum Gene Ther. 2008;19:1249–1260.
- Wahl RL, Jacene H, Kasamon Y, et al. From RECIST to PERCIST: evolving considerations for PET response criteria in solid tumors. J Nucl Med. 2009;50(Suppl.1):122S–150S.
- Brown PA, Bodles-Brakhop AM, Pope MA, et al. Gene therapy by electroporation for the treatment of chronic renal failure in companion animals. BMC Biotechnol. 2009;9:4.
- Bodles-Brakhop AM, Brown PA, Pope MA, et al. Double-blinded, Placebo-controlled plasmid GHRH trial for cancer-associated anemia in dogs. MolTher. 2008;16:862–870.
- Romeo S, Wu YH, Levine ZA, et al. Water influx and cell swelling after nanosecond electropermeabilization. Biochim Biophys Acta. 2013;1828:1715–1722.
- Mahmood F, Hansen RH, Agerholm-Larsen B, et al. Detection of electroporation-induced membrane permeabilization states in the brain using diffusion-weighted MRI. Acta Oncol. 2015;54:289–297.
- Gissel H. Effects of varying pulse parameters on ion homeostasis, cellular integrity, and force following electroporation of rat muscle in vivo. Am J Physiol Regul Integr Comp Physiol. 2010;298:R918–RR29.
- Bureau MF, Naimi S, Ibad RT, et al. Intramuscular plasmid DNA electrotransfer: biodistribution and degradation. Biochim Biophys Acta. 2004;1676:138–148.