Abstract
We investigated dissipation and sorption of atrazine, terbuthylazine, bromacil, diazinon, hexazinone and procymidone in two contrasting New Zealand soils (0–10 cm and 40–50 cm) under controlled laboratory conditions. The six pesticides showed marked differences in their degradation rates in both top- and subsoils, and the estimated DT50 values for the compounds were: 19–120 (atrazine), 10–36 (terbuthylazine), 12–46 (bromacil), 7–25 (diazinon), 8–92 (hexazinone) and 13–60 days for procymidone. Diazinon had the lowest range for DT50 values, while bromacil and hexazinone gave the highest DT50 values under any given condition on any soil type. Batch derived effective distribution coefficient (K d eff) values for the pesticides varied markedly with bromacil and hexazinone exhibiting low sorption affinity for the soils at either depth, while diazinon gave high sorption values. Comparison of pesticide degradation in sterile and non-sterile soils suggests that microbial degradation was the major dissipation pathway for all six compounds, although little influence of abiotic degradation was noticeable for diazinon and procymidone.
Introduction
Modern agriculture relies increasingly on the use of pesticides to meet the ever-growing need for food and fiber. While pesticides are indispensable to increase the quantity and quality of food commodities and to safe guard society through better health and higher living standards, their off-site migration and detrimental effects on surface water and groundwater quality are of environmental concern.
Once released to the soil, sorption and degradation are two governing processes that determine the distribution and persistence of pesticides. Pesticides can undergo degradation either through biological and or abiotic pathways, including photolysis. Microbial transformation can take place directly through metabolic processes like mineralization, co-metabolism, conjugation and accumulation.[ Citation 1 ] Apart from the chemical properties (structure, solubility, concentration, etc.) of the pesticide in question, a plethora of other soil and environmental factors such as pH, clay and organic matter content, moisture content and temperature also affect the rate of degradation for pesticides in soil. Microbial activity in soil varies according to profile depth, as do the quantity of organic matter, clay composition, temperature and moisture content within that soil profile.[ Citation 2 ] In addition, these factors often vary from site to site and from year to year (e.g. temperature and moisture). Therefore results obtained from any study of pesticide persistence in the field are specific to the particular location and season.[ Citation 3 ] For these reasons degradation results obtained from overseas studies for a given pesticide can not be accurately extrapolated for a different soil type in another country. Therefore, site- and soil type-specific information for a particular compound should be obtained by performing controlled laboratory or field degradation experiments. Similarly sorption affinity for soils for a range of pesticides in different locations within a country could also vary markedly due to soils' physical and chemical properties as well as mineralogical characteristics and this can indirectly affect rate of dissipation of a given pesticides in the soils.
From the New Zealand farmers' perspective, pesticides have been of considerable financial benefit, and no other method of weed and pest management has been as cost-effective.[ Citation 2 ] However, financial benefits from the use of pesticides need to be balanced against negative environmental and health effects.[ Citation 4 ] According to a report released in 2002, by the New Zealand Ministry for the Environment, herbicides continue to dominate pesticide use, accounting for nearly 68%, followed by fungicides (24%) and insecticides (8%). Although the majority of groundwaters in New Zealand appear free from pesticide contamination,[ Citation 4 ] recent surveys have found residues of a number of pesticides in 21–35% of the wells sampled, albeit at low concentrations.[ Citation 5 ] Furthermore, earlier surveys by the local government authorities also revealed that shallow groundwaters underlying free draining soils contained a number of pesticides.[ Citation 6 ] These surveys thus indicate a need for better data collection on the fate of a number of commonly used pesticides detected in the groundwaters. Currently, there is no information on important environmental parameters such as sorption coefficients, and half-lives or DT50 values for a number of pesticides under varied climatic and soil conditions in New Zealand. Both sorption coefficient and dissipation half-life are important input parameters in many pesticide-fate modeling studies and for risk assessment purposes, and must be measured accurately under controlled laboratory or field experiments. The term “dissipation” has been preferred in place of “degradation” in this work as we did not attempt to identify metabolites formed during the course of this investigation.
Atrazine (2-chloro-4-ethylamino-6-isopropylamino-1,3,5-s-triazine), bromacil (5-bromo-3-sec butyl -6-methyluracil), hexazinone (3-cyclohexyl-6-dimethylamino-1-methyl-1,3,5-triazine-2,4(1H,3H)-dione), terbuthylazine (2-tert-butylamino-4-chloro-6-ethylamino-1,2,5-triazine) are some of the herbicides commonly used for weed control in horticulture and arable farming in New Zealand. Procymidone N-(3,5-dichlorophenyl)-1,2-dimethylcyclopropane-1,2-dicarboximide) is a fungicide currently registered for use in variety of fruits and vegetables in New Zealand to control various pathogens and diseases, while diazinon (O,O-diethyl O-2-isopropyl-6-methylpyrimidin-4-yl phosphorothioate), a broad spectrum organophosphate insecticide is commonly used on New Zealand apple orchards specifically for the control of apple leaf-curling midge (ALM) (dasineura mali Diptera: Cecidomyiidae).[ Citation 7 , Citation 8 ]
While degradation half-lives for these pesticides are available in the literature, given the varied soil and climatic conditions of New Zealand, extrapolation of degradation data obtained from overseas studies to New Zealand conditions is not desirable. The purpose of this study was to conduct laboratory incubation experiments for the six pesticides on two soils (top and subsoil) collected from the South Island of New Zealand at two temperatures and specified water content and estimate the half-lives, and to compare the values obtained in this study with those obtained under similar conditions in overseas studies. Another intention was the use of the half-life values in simulation modelling exercise as input parameters and for regulatory decision-making purposes for those New Zealand regional councils concerned.
Materials and methods
Soils and pesticides
Fresh top (0–20 cm) and subsoils (20–40 cm) from a Waikiwi silt loam near Invercargill (Typic firm brown silty loam), and a Motupiko silt loam near Wakefield, Nelson (recent fluvial silt loam over gravely alluvium), were sieved (2 mm), and water contents were measured immediately. Soils collected from the two sites had no history of previous pesticide use at all and are representative of the farm soils in the region. The physical and chemical properties of the soils are presented in . Organic carbon (OC) was determined using an improved chromic acid digestion and spectrophotometric procedure,[ Citation 9 ] and particle size distribution was determined by the pipette method.[ Citation 10 ] Soil microbial biomass carbon was measured by fumigation-extraction technique.[ Citation 11 ] Soils were fumigated in CHCl3 for 24 h and extracted in 0.5 M K2SO4. Organic C in the extracts was measured by Shimadzu 5000 TOC analyzer and the flush of C (difference between fumigated and non-fumigated soils) converted to microbial biomass using a KEC (extractable part of microbial biomass carbon after fumigation) factor of 0.41.[ Citation 12 ]
Table 1 Selected soil properties used in the degradation study.
Analytical grade atrazine (98%), bromacil (99.5%), hexazinone (99%), diazinon (97.5%), procymidone (98%) and terbuthylazine (99.5%) were purchased from Dr Ehrenstorfer, Germany. The structure and chemical properties of these compounds are given in .
Table 2 Properties of pesticides (Wauchope et al. [ Citation 28 ]; FOOTPRINT 2006).
Laboratory batch sorption studies
Batch sorption studies were performed for each pesticide in the topsoil and subsoils collected from each site. Briefly, 25 g of each air-dried soil were equilibrated with 50 mL of 0.005 M CaCl2 solution containing the single pesticide at 7 initial concentrations (0.01, 0.05, 0.1, 0.5, 1, 5 and 10 mg L–1) in 50 mL glass centrifuge tubes with Teflon-lined screw caps. Samples were shaken in the dark at 23 (± 2)°C, in an end-over-end shaker for 20 h, centrifuged, and an aliquot of the supernatant analyzed using gas chromatography (GC) as described later. Control solutions containing pesticides without soil (controls), and control blanks containing soil were included in all experiments to determine container sorption losses and check for peaks that may interfere during analysis. Sorption coefficients were determined using the Freundlich model: C s = K f C w N , where C s (μ g g− 1) is the amount of pesticide sorbed to soil, C w (μ g mL− 1) is the aqueous equilibrium concentration, K f (μ g1 − N mL N g− 1) is the Freundlich sorption coefficient and N (dimensionless) is a measure of sorption non-linearity (N = 1 for linear isotherm).
Laboratory dissipation studies
Appropriate duplicate amounts of air-dried soil from each depth from the two sites were placed on an aluminium foil sheet, and a known amount of pesticide (atrazine, bromacil, diazinon, hexazinone, terbuthylazine, and procymidone) solutions was individually spiked and thoroughly mixed with a spatula to give nominal soil concentrations of 3.3 mg/kg (atrazine and terbuthylazine) and 0.55 mg kg− 1 (hexazinone, diazinon, bromacil, procymidone), respectively. The concentrations were chosen on the basis of recommended application rates for these compounds in New Zealand. Water was added to adjust the soil water content to 60% maximum water-holding capacity (MWHC) measured at −10 kPa and incubated in the dark (20°C and 7.5°C) to simulate the average soil temperatures of the two sites from where samples were collected. Water was added once a week to maintain the soil water content (weight basis) during the experiment. One set of soil from each site was autoclaved on 3 consecutive days (at 1 kg cm− 2, 120°C for 60 min) to remove microbial activity and was used as sterile control treatments. Duplicate sub-samples (40 g) from each fortified bulk sample for each depth and site were periodically removed on day 0, 7, 14, 45, 70 and 120, and analyzed for residues by gas chromatography equipped with an electron capture detector (GC-ECD; diazinon, bromacil, procymidone) and thermionic specific detection (TSD; atrazine, hexazinone, terbuthylazine).
Extraction and analysis
Duplicate samples (25 g) from dissipation studies were extracted with 100 mL ethyl acetate, followed by 10 minutes sonication, and mechanically shaken for 2 hours. The extracted sample was filtered, and cleaned with gel permeation chromatography (GPC). GPC cleanup was achieved using a Shimadzu liquid chromatograph (LC-10) equipped with a UV detector and a fraction collector, coupled to a Biobeads SX-3 column (45 cm × 1 cm diameter) using ethylacetate/cyclohexane (1:1 v/v) as mobile phase. An aliquot of 1 mL of the concentrated sample extract was injected onto the GPC column and the fraction containing the pesticide analytes was collected in a glass vial. The clean sample extracts were concentrated and transferred to an autosampler vial for analysis. Analysis was performed by gas chromatography with electron capture (procymidone and bromacil) and thermionic specific detection (atrazine, hexazinone, diazinon and terbuthylazine) using a Varian 3500 Gas Chromatogram and 8100 temperature programmable auto-injector. The limit of detection was 0.01 mg kg− 1 for all 6 pesticides studied. The percent recoveries of pesticides from the fortified soils were > 80% at the experimental concentration levels.
During the gas chromatography mass spectrometry (GCMS) analysis the injector temperature was held at 80 °C for 0.3 minute then increased at 160 °C min− 1 to a final temperature of 250 °C for the complete run. Injection was done in split/splitless mode using a Hewlett Packard Ultra-2 capillary GC column (30 m × 0.25 mm i.d. × 0.33 μ m) connected to a column effluent splitter to split and transfer the column effluent to the ECD and TSD. Helium was used as carrier gas at a flow rate of 1.1 mL min− 1. The temperature of the column was held constant at 70 °C for 2 minutes, increased to 135 °C (1 minute hold) at 15 °C min− 1, then to 180 °C at 1.4 °C min− 1 (no hold), followed by 2.5 °C min− 1 to 200 °C (no hold), then increased at 50 °C min− 1 to 300 °C, where it was held for 7 minutes. The compound retention times were: atrazine (26.625 min), tebuthylazine (28.258 min), diazinon (29.966), bromacil (39.235 min), procymidone (45.093 min) and hexazinone (47.848 min).
Multi-factorial statistical analysis
Three-way analysis of variance (ANOVA) was performed to examine the effects of soil type, soil depth and temperature on the DT50 values of each pesticide. Soil type and soil depth were treated as fixed effects (for depth we were interested only in differences between topsoil and subsoil, rather than the general relationship between depth and pesticide DT50 values), while temperature was treated as a random effect. Significance testing was performed using permutations, following the guidelines of Anderson and ter Braak.[ Citation 13 ] There were not enough treatment levels to obtain adequate power for an exact mixed model test (where treatment combinations rather than individual observations are permuted). Consequently, an approximate test was performed where, in testing the significance of each factor, the effect of all other factors was removed by analyzing the residuals from these factors rather than raw data. This test provides appropriate type-I errors and good power for most types of error distribution in mixed models, providing there is no interaction between fixed and random effects. In the presence of interactions there may be a slight inflation of type-I error in some instances, [ Citation 13 ] though this is only relevant where effects are marginally significant. For each test, 10, 000 permutations were used to obtain p-values, where p is the proportion of permutations giving an F-statistic greater than or equal to that observed. The significance of all possible interactions between factors was also examined using permutation of residuals. In this case, residuals were taken from all factors involved in the interaction in question. Statistical analysis was carried out only for dissipation data and not for sorption results.
Results and discussion
Batch sorption studies
Freundlich model derived sorption parameters for the pesticides in topsoil and subsoil from both sites are presented in along with their respective N and r 2 values. In Motupiko soil, values for N ranged between 0.87–1.34 (topsoil) and 0.92–1.08 (subsoil), however, they were consistently < 1 (N = 0.76–0.83) in Waikiwi topsoil and varied from 0.87–1.24 in the subsoil for the pesticides studied. While there is no universally defined threshold value for N to address the sorption non-linearity, Pignatello et al.,[ Citation 14 ] however, suggest that from practical perspective, any isotherm with 1.05 > N > 0.95 could be considered linear with caution required in interpreting the data as N is sensitive to the concentration range used in the study. Due to observed inconsistency in isotherm linearity for majority of soil-solute combination in our study, it was difficult to compare K d or K oc values between solutes or soils as they were concentration-dependent. Therefore, in order to compare sorption across soils, a concentration-dependent effective distribution coefficient (K d eff = K f C w N − 1) was calculated with the lowest equilibrium concentration of 0.01 mg L− 1 (). Like wise, concentration-dependent organic carbon-normalized partition coefficient values (K oc , L kg− 1 OC) were calculated for each soil and pesticide with equation K oc = K f C w N − 1 f oc − 1, where f oc is the fraction of soil organic carbon (% OC × 100− 1). The K oc defined through this approach is equivalent to a single-point OC-normalized distribution coefficient determined at a particular concentration (C e ); however, is not the same as slope of the linearized isotherm, as it is commonly defined in the literature.[ Citation 14 ]
Table 3 Summary of batch isotherm parameters for the pesticides in Motupiko and Waikiwi soils.
There was marked difference in the effective distribution coefficients (K d eff ) for the pesticides within each soil type. By far, diazinon sorption onto soils from both depths was much higher than the other compounds (). Overall, bromacil and hexazinone gave consistently lower values for sorption, while diazinon had highest K oc values in topsoils from Waikiwi and Motupiko sites. An observation to note is the higher K oc values in Motupiko subsoil for the pesticides, which could be attributed to higher clay content of Motupiko subsoil. The soils in this site originated from alluvial material consisting of clay minerals such as kaolinites and have high oxide surfaces which can give rise to high sorption values for some pesticides (Malcolm McLeod, personal communication). Overall the sorption affinities for both diazinon and terbuthylazine to Motupiko and Waikiwi topsoils were greater as compared with other pesticides. Bromacil and hexazinone exhibited much lower sorption to these soils at both depths. Low K oc values for hexazinone (12–69) and bromacil (40–75) in the topsoils also suggested that the compounds have the potential for greater mobility under conditions conducive to leaching and this was consistent with field observations for these compounds in a recent study at these sites.[ Citation 15 ]
Dissipation studies
Results of dissipation studies for six pesticides in top and subsoils at various sampling times are plotted in for Waikiwi soil and in for Motupiko soil. A visual examination of degradation pattern for the herbicides at both depths suggest significant deviation from first-order kinetic and this was further proved by our initial attempt to fit the data using first-order kinetic, which was less satisfactory (r 2 range of 0.58–0.9 for most compounds). Therefore, we present the data as percent of each compound dissipated from the initial application on soil, and the corresponding values for DT50 (50% dissipation time) are graphically estimated from and . In the companion paper, however, we discuss in more detail about the application of non-linear degradation models to describe the observed two-phase kinetic and the model derived DT50 and DT90 values for each compound.
Fig. 1 Plots of dissipation for the six pesticides in non-sterile (bar) Waikiwi topsoil and subsoil, at constant temperature of 20°C (A) and 7.5°C (B) and water content of 60% water-holding capacity (MWHC) (−10 kPa). Sterile control treatments are shown in dashed lines and symbols. Error bars show the range of duplicate results.

Fig. 2 Plots of dissipation for the six pesticides in non-sterile (bar) Motupiko topsoil and subsoil, at constant temperature of 20°C (A) and 7.5°C (B) and maximum water content of 60% water-holding capacity (MWHC) (−10 kPa). Sterile control treatments are shown in dashed lines and symbols. Error bars show the range of duplicate results.
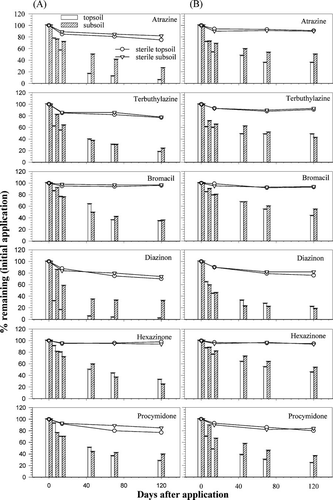
Effect of temperature and soil depth on dissipation
Atrazine
The six pesticides showed wide differences in their dissipation rates in both topsoil and subsoils at a constant temperature of either 20°C or 7.5°C and a specified water content of 60% MWHC (−10kPa). It can be observed that > 90% and > 70% of applied atrazine degraded respectively in the topsoil and subsoil (). However, atrazine degradation was slow at 7.5°C with nearly 40% (topsoil) and 60% (subsoil) of the applied amount still remaining at the end of day 120 (). The three-way analysis of variance (ANOVA) results show that there was highly significant difference in the DT50 values for atrazine between the top and subsoils and the two temperatures (p value < 0.001), however, the soil type did not show any significant effect. Interaction between the soil type, depth and temperature on the DT50 values for atrazine was found to be significant at p < 0.05. This indicates that the effects of soil depth and temperature on DT50 were partially dependent on each other and on soil type.
Increases in temperature and decreasing soil depth have been found to affect degradation rate for many pesticides and has been demonstrated by number of researchers.[ Citation 16 , Citation 17 , Citation 18 ] With increasing soil depth, there is reduced microbial activity and organic carbon content, which reduces the sorption capacity of soils for organic compounds; thus degradation can be slowed. Graphically determined DT50 values for atrazine ranged from 19 and 46 days in the Waikiwi topsoil at 20°C and 7.5°C respectively. However, they were ∼ two-fold higher in the subsoil, emphasizing the micro-organisms' role in the upper layer of the soil profile. Motupiko soil also gave similar DT50 values (). Dousset et al.[ Citation 19 ] reported atrazine half-life (T1/2) to vary from 66 to 105 days in 3 agricultural soils near Orleans in France at 20°C but at a slightly higher water content (80%) than the present study. In Spain, Obrador et al.[ Citation 20 ] calculated T1/2 values of 55 days for atrazine in a loamy soil with only 0.5% organic carbon, but at a temperature of 25°C, which perhaps explains slightly lower half-life value than the one obtained in our study. In contrast to the above findings, Muller et al.[ Citation 21 ] found T1/2 value for atrazine to be only 12 and 13 days in a New Zealand allophanic soil, when incubated at 20°C and 7.5°C respectively at 60% MWHC. It is possible that the significantly higher level of organic carbon (∼ 8%) may have contributed to higher degradation for the compound in that soil. In both soil types, some abiotic hydrolysis seemed to influence the overall atrazine degradation with 5 to 15% of the applied amount under sterile treatment degraded by day 120. It is important to note a direct comparison of T1/2 values reported by various authors are difficult to compare with our DT50 values as the approaches were different and our data did not follow first-order kinetic.
Terbuthylazine
Of the applied amount, 20 to 30% of terbuthylazine still remained at the end of 4 months at 20°C in the Motupiko soil, however, during the same period, > 40% of the applied terbuthylazine could still be detected at 7.5°C. The incubation in reduced temperature slowed the rate of dissipation of terbuthylazine in Motupiko soil irrespective of depths, with only about 2–10% dissipating within day 7 to day 120. However, degradation was relatively faster in Waikiwi soil and this was evident in the derived DT50 values for the compounds (). In Motupiko soil, terbuthylazine persisted in higher amounts and DT50 values were > two-fold higher than in Waikiwi soil (, ) despite lower OC (0.26–2.4) and reduced microbial biomass in the Motupiko soil (). This could be attributed to higher clay content (26%) in Motupiko soil resulting higher sorption () and thus reduction in degradation of terbuthylazine. There was significant difference (p = 0.001) in the DT50 values for terbuthylazine between the two soil types and between topsoil and subsoil, however no significant temperature effect was observed. T1/2 values for terbuthylazine in the soil have been reported to vary between 5 and 116 days depending on the soil characteristics and temperature.[ Citation 19 , Citation 22 , Citation 23 ] The T1/2 values for terbuthylazine in one soil increased to 144 days at 10°C from 68 days at 20°C. [ Citation 24 ] Similarly, this study also demonstrated a tendency (though non-significant) for an increase in terbuthylazine DT50 with decreasing temperature, with > two-fold increases in DT50 value in the Motupiko soil ().
Table 4 DT50 values for the six pesticides in Waikiwi and Motupiko soils at two temperatures and water content of 60% MWHC (−10 kPa).
Bromacil
All three factors had a significant effect on DT50 values for bromacil (p values < 0.001 to 0.004), and the three-way interactions between soil type, depth and temperature was also significant (). Bromacil degraded faster in Waikiwi soil than Motupiko soil when incubated at 20°C, with graphical DT50 values of 12 and 40 days, for top and subsoil respectively. However, values were 3 times higher when incubated at 7.5°C (). There was no evidence of abiotic hydrolysis as the sterile treatment essentially remained the same throughout the sampling period. In Waikiwi subsoil, degradation of bromacil was very slow with only about 25% of the compound degrading by day 40, however, after that no loss of bromacil was observed and DT50 value could not be determined (). An attempt to monitor the viability of microbial biomass during the study period for each sampling time was not made in this study. However, we speculate that the apparent slow degradation of bromacil could be attributed to the loss of microbial activity or the fraction that is irreversibly bound to soil organic domain and could not be extracted with organic solvent. Furthermore organic-carbon normalized sorption coefficient (K oc ) value for bromacil was > 17000 (), which lends support to the observed slow degradation rate for the compound in the subsoil.
Table 5 Results from three-way analysis of variance for each pesticide. Fobs is the observed F-statistic, Fexp is the mean F-statistic taken across the permutations while ‘p’ values indicate the proportion of simulations yielding a p-value greater than or equal to that observed.
The DT50 values of bromacil in topsoil found in this study were lower than those reported in the literature,[ Citation 25 , Citation 26 ]although a direct comparison was difficult due to difference in the environmental conditions used in the respective studies. Overall, bromacil degradation was slowest among all the compounds studied under any given condition, with > 40% of the applied amount still remaining in the soils from both depths and sites, with the exception in Waikiwi topsoil at 20°C, where only about 12% of the applied bromacil remained in the soil. ()
Diazinon
Depth was the only factor to have a significant effect on DT50 for this compound. There was essentially no difference in degradation rate for diazinon at either depths at 20°C in Waikiwi soils, and corresponding DT50 values were 7 and 8 days respectively in top and subsoils. However, DT50 values in the Waikiwi subsoil increased when incubated at lower temperature (). Motupiko subsoil gave slightly higher DT50 values for diazinon at 20°C, however, values were same for both depths under 7.5°C and reason for this is not clear. Corresponding DT50 values found in the topsoils from both Waikiwi and Motupiko were lower than the reported half-lives for the compound elsewhere. Sattar[ Citation 27 ] reported mean T1/2 for diazinon as 28 days and 36 days, in a silty clay (OC = 0.6%) and a sandy clay (OC = 0.5%) soil respectively. The author incubated at a much higher temperature (25°C) than the present study, and the soils had much lower organic carbon content, which may explain higher T1/2 values for the compound in those soils. The T1/2 of diazinon under aerobic conditions has been reported in the range of 3 to 40 days in soils.[ Citation 28 , Citation 29 ] Sterile control treatment for diazinon indicates some contribution of abiotic process in the overall degradation. For example, some loss through chemical hydrolysis for diazinon was apparent in both Waikiwi and Motupiko soils with the former accounting for nearly 30% loss in the sterile samples, while in Motupiko soil, the loss was ∼ 20%. Therefore, rapid disappearance of diazinon in these soils was a combined effect of biotic and abiotic hydrolysis. Hydrolysis is an important factor for diazinon degradation especially under acidic organic soil[ Citation 30 ] and the soil pH in our study ranged between 5.1–6.1, and therefore overall rapid dissipation of diazinon in our study as compared to what has been reported in the literature was not surprising.
Hexazinone
All three factors had a significant effect on DT50 values for bromacil, and the three-way interaction between soil type, soil depth and temperature was significant (). After 120 days of incubation at 20°C, 98% and 45% of the applied hexazinone degraded with DT50 of 8 and 92 days, in the Waikiwi topsoil and subsoil respectively. Motupiko topsoil gave a DT50 value of 45 days at 20°C, consistent with the lower level of OC present in the soil and low sorptive affinity of hexazinone for the Motupiko soil (). Sterile control treatment had little influence in overall degradation process with abiotic hydrolysis accounting for < 8% of compound loss (Waikiwi), while no abiotic degradation took place in Motupiko soil ( and ). Calderón et al.[ Citation 31 ] reported hexazinone half-life of 47 and 91 days for two Spanish agricultural soils at 20°C; however, water content values were not reported. Other soil studies have determined DT50 for hexazinone to vary as low as 10 to as high as 175 days.[ Citation 32 , Citation 33 , Citation 34 ] Holland et al.[ Citation 35 ] reported T1/2 of 72 days for hexazinone in 2 soils from New Zealand that had similar pH values and OC content. Available literature data thus correspond well with the values obtained in the current study for hexazinone; however, caution is needed when comparing them as not all experiments were conducted using the same experimental protocol, and soils vary significantly in their physico-chemical properties as well as in the microbial populations responsible for degrading the particular pesticide in question.
Procymidone
Depth was the only factor to have a significant effect on DT50 for this compound, though the three-way interaction was also significant (). Dissipation of procymidone was relatively faster in the Waikiwi soil as compared with the Motupiko soil with estimated DT50 values of 9 and 24 respectively, in top and subsoil. Decrease in the DT50 value at 7.5°C, was however, an exception from the general assumption that with decreasing temperature pesticides undergo slower degradation. Change in DT50 values for procymidone was also not observed in the Waikiwi soil between the two temperatures and earlier diazinon also behaved in a similar pattern (). Overall, for Motupiko topsoil and subsoil, T1/2 for procymidone ranged from 13 to 60 days at the incubated temperatures of 7.5–20°C, while in the Waikiwi soil, it ranged from 9 to 120 days. Degradation half-life values for this compound are not readily available, and the only comparison that we could make with our data (), was to the range of degradation half-life reported for procymidone (7–120 days) at: www.arsusda.gv/acsl/ppdb.html. This comparison shows that present data set obtained in our experiment fall within range of T1/2 values for procymidone available in the literature.
Comparison of DT50 values between the treatments
In an attempt to draw comparison of pesticide dissipation under different soil types, depths and temperatures, plots between the estimated DT50 values against the pesticides were shown in . Diazinon gave by far the lowest DT50 values (5–24) among all pesticides under any given soil type, depth and temperature () and except soil depth, neither soil type nor temperature influenced the degradation significantly. For both atrazine and diazinon, no interactions between the treatments were observed, however, atrazine DT50 values were significantly affected by depth and temperature (p < 0.001), as well as significant interactions between the treatments were also observed. In general, soil depth had positive effects on the DT50 values for the pesticides with p values ranging between < 0.001 to 0.007 (). In most cases there was no two-way interaction between either of the fixed effects and temperature, though there were some instances of three-way interactions (). For example, atrazine, bromacil, hexazinone and procymidone, three-way interactions were found with atrazine also showing depth and temperature interactions and both highly significant at p < 0.001 (). For bromacil, a DT50 value could not be obtained in the Waikiwi soil, because of slow rate of dissipation which could have been caused by loss of soil microbial activity with > 80% of the applied bromacil could still be detected on day 120 in the Waikiwi topsoil (7.5°C). Estimated DT50 values for bromacil and hexazinone were found to be > 140 days, which were higher than any pesticide for any given condition. There was a marked difference in the DT50 values between the compounds under any given condition with DT50 ranging from as low as 5 days (diazinon) to as high as > 140 days for bromacil and hexazinone (). Temperature had a clear effect on the rate of dissipation for some pesticides (atrazine, bromacil and hexazinone at p < 0.05), with increase in the DT50 values by two-folds and sometimes higher with the decrease in temperature (20 to 7.5°C) in either soil type (). Similarly DT50 values for majority of the pesticides in the subsoil increased, which was consistent with assumption that reduced organic carbon and lower microbiological activity at greater depths hinders degradation.
Fig. 3 Plots showing graphically determined DT50 values for each pesticide in Waikiwi silt loam and Motupiko silt loam soils at two soil depths and temperatures. Vertical bars represent standard errors of means (n = 2). DT50 for bromacil in Waikiwi subsoil (7.5°C) could not be determined, while DT50 values for bromacil and hexazinone in Motupiko subsoil were found to be > 140 days.
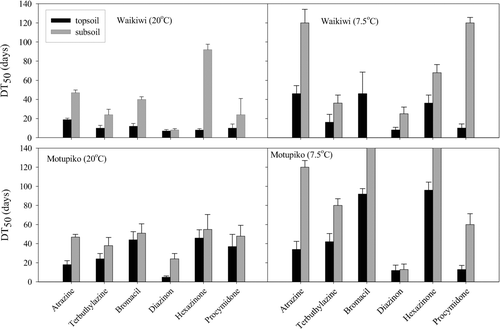
It would appear from data obtained that interplay of several factors may have been responsible for the dissipation of the compounds. The link between sorption and dissipation is not always straightforward and often there are difficulties associated with determining the effect of single soil property on the dissipation of pesticide, especially when the experiment is performed using only a few soil types.[ Citation 36 ] For instance, often a single property such as organic carbon can have strong influence on sorption; however, this effect can be masked by the dominant clay minerals or the microbial biomass, both the degree of biological activity and the presence of specific degraders, as well as the pH level in the soil type which also influence the overall dissipation process. In this study, a close examination of sorption and degradation data revealed a general trend of faster degradation associated with stronger sorption and this was evident in both soil types. Kah et al.[ Citation 36 ] also found a similar relationship between sorption and degradation data for dicamba, metsulfuron-methyl and pirimicarb in arable southern England soils. We postulate that the dissipation and estimated DT50 values for the compounds in our study were dependent on the type and numbers of specific degraders present in these soils and differed in their ability to degrade one specific compound. An attempt to isolate the types of micro-organisms present in these soils was beyond the scope of the present study, and perhaps this can be seen as a possible future research area to shed some insights into the degradation mechanisms of these compounds in the soils investigated.
The two soils used in this study were from two contrasting sites, one with much wetter climate, greater percentage of organic carbon and microbial biomass (Waikiwi soil), while Motupiko soil came from a site much drier and significantly lower level of organic carbon and corresponding microbial biomass (). In some instances, the rates of dissipation of some pesticides may have been due to combination of both biotic and abiotic degradation; however a comparison between non-sterile and sterile data revealed that microbial degradation was the major dissipation pathway for these compounds. The rates of degradation for terbuthylazine, bromacil and procymidone were much slower in Waikiwi soils at both depths, except for diazinon, which degraded in subsoil at a rate similar to topsoil. The reduced rate of degradation for all the pesticides in Motupiko soil can be expected, given lower OC content and corresponding microbial biomass in Motupiko soil. However, the degradation pattern was similar to what we observed in Waikiwi soil. Under both soil types, all pesticides showed rapid degradation during the first few sampling days, but with time since exposure, the rate of degradation slowed markedly and an apparent bi-phasic kinetic seemed to dominate the degradation of most compounds.
Among the compounds studied, diazinon had the lowest DT50 values for any given condition on any soil type except in Motupiko subsoil at 20°C, where rate of degradation of diazinon was slow. Overall, faster degradation of diazinon observed in this study also corresponded well with the reported low T1/2 values for the compound. Although there was a wide variation (0.7 to 5.7 fold) in DT50 values for the compounds with temperature, most (79%) showed an increase in DT50 with decreasing temperature. The mean difference between 20° and 7.5°C was a factor of 2.0 for both top and subsoils.
Conclusion
A marked difference was observed between the soils in their ability to degrade the pesticides. It is conceivable that within one soil type, different layers may contain different microbial populations that are preferential degraders of different pesticides.[ Citation 12 ] In addition, the combined effect of sorption and degradation are also influenced by the organic carbon content and other mineral constituents present within the soil depth, which can have an interactive effect on overall degradation process. Given the complex interaction between the sorption and degradation, the lack of consistent protocol often renders a global approach to obtain DT50 values for different pesticides unrealistic. Therefore site specific data must be obtained for a given pesticide so that appropriate decision making can be made for the environmental safety.
Acknowledgments
This study was funded by contracts CO9X0017 (Landcare Research) and CO3X0303 (ESR) from the Foundation for Science, Research and Technology (New Zealand). We thank Janine Ryburn of Landcare Research for technical assistance and HortResearch, Ruakura for analyzing the pesticide samples. Frank Scherr is thanked for comments on the manuscript and help in some graphics.
Notes
∗Concentration-dependent OC-normalized sorption coefficient calculated using K oc = K f C w N − 1f oc − 1 at C w = 0.01 μ g mL− 1, and at an assumed environmental concentration of 0.04 μ g L− 1 for the pesticides.
References
- Bollag , J. M. and Liu , S. Y. 1990 . “ Biological transformation processes of pesticides ” . In Pesticides in the soil environment: Processes, impacts, and modelling , Edited by: Cheng , H. H. 169 – 211 . Madison, WI : SSSA .
- Sarmah , A. K. , Muller , K. and Ahmad , R. 2004 . Fate and behaviour of pesticides in the agro-ecosystem—a review with a New Zealand perspective . Aust. J. Soil. Res. , 42 : 125 – 257 .
- Hurle , K. and Walker , A. 1988 . “ Persistence and its prediction ” . In Interactions between herbicides and the soil , Edited by: Hance , R. J. 83 – 122 . New York : Academic Press Inc .
- Ministry for the Environment (MfE) . 2002 . Towards a pesticide risk reduction policy for New Zealand. A public discussion paper , 1 – 67 . Wellington, NZ : MfE .
- Close , M. E. and Flintoft , M. J. 2004 . National survey of pesticides in groundwater in New Zealand –2001 . NZ J. Marine. Freshwater. Res. , 38 : 289 – 299 .
- Hadfield , J. and Smith , D. 1997 . Pesticide contamination of groundwater: An investigation in the Waikato Region, New Zealand . Proceedings of the 24th Water & Hydrology Symposium . Nov. 24–28 1997 , Engineers Australia. pp. 246 – 251 . Auckland : NZ Hydrological Society .
- Smith , J. T. and Chapman , R. B. 1997 . Apple leafcurling midge egg laying on different apple cultivars and orchard properties on the Waimea Plains . Nelson. Proc. 50th N. Z. Plant Prot. Conf . Aug. 18–21 1997 . pp. 247 – 251 . Lincoln, NZ : NZ Plant Protection Society .
- Tomkins , A. R. , Wilson , D. J. , Hutchings , S. O. and June , S. 1994 . A survey of apple leafcurling midge (Dasyneura mali) management in Waikato orchards . Proc. 47th N. Z. Plant Prot. Conf . Aug. 9–11 1994 . pp. 346 – 349 . Waitangi, NZ : NZ Plant Protection Society .
- Heanes , D. L. 1984 . Determination of total organic-C by an improved chromic acid digestion and spectrophotometric procedure . Commun. Soil Sci. Plant. Anal. , 10 : 1191 – 1213 .
- Day , P. R. 1965 . “ Particle fractionation and particle-size analysis ” . In Methods of Soil Analysis, Part I , Edited by: Black , C. A. 545 – 567 . Madison, WI : American Society of Agronomy .
- Jenkinson , D. S. and Powlson , D. S. 1976 . The effects of biocidal treatments on metabolism in soil. V. A method for measuring soil biomass . Soil. Biol. Biochem. , 8 : 209 – 213 .
- Sparling , G. , Dragten , R. and Aislabie , J. 1998 . Atrazine mineralisation in New Zealand topsoils and subsoils: influence of edaphic factors and numbers of atrazine-degrading microbes . Aust. J. Soil Res. , 36 : 557 – 570 .
- Anderson , M. J. and ter Braak , C. J. F. 2003 . Permutation tests for multi-factorial analysis of variance . J. Statist. Comput Simul. , 73 : 85 – 113 .
- Pignatello , J. J. , Lu , Y. , LeBoeuf , E. J. , Huang , W. , Song , J. and Xing , B. 2006 . Nonlinear and competitive sorption of apolar compounds in black carbon-free natural organic materials . J. Environ. Qual. , 35 : 1049 – 1059 .
- Close , M. E. , Sarmah , A. K. , Flintoft , M. J. , Thomas , J. and Hughes , B. 2006 . Field and laboratory study of pesticide leaching in a Motupiko silt loam (Nelson) and in a Waikiwi silt loam (Southland) . Aust. J. Soil Res. , 44 : 569 – 580 .
- Di , H. J. , Aylmore , L. A. G. and Kookana , R. S. 1998 . Degradation rates of eight pesticides in surface and subsurface soils under laboratory and field conditions . Soil. Sci. , 163 ( 5 ) : 404 – 411 .
- Sarmah , A. K. , Kookana , R. S. and Alston , A. M. 1999 . Degradation of chlorsulfuron and triasulfuron in alkaline soils under laboratory conditions . Weed. Res. , 39 : 83 – 84 .
- Bromilow , R. H. , Evans , A. A. and Nicholls , P. H. 1999 . Factors affecting degradation rates of five triazole fungicides in two soil types: 1. Laboratory incubations . Pestic. Sci. , 55 : 1129 – 1134 .
- Dousset , S. , Mouvet , C. and Schiavon , M. 1997 . Degradation of 14C terbuthylazine and 14C atrazine in laboratory soil microcosms . Pestic. Sci. , 49 : 9 – 16 .
- Obrador , A. , Lechon , T. and Tadeo , J. K. 1993 . Simulation of atrazine persistence in Spanish soils . Pestic. Sci. , 37 : 301 – 308 .
- Müller , K. , Smith , R. E. , James , T. K. , Holland , P. T. and Rahman , A. 2003 . Spatial variability of atrazine dissipation in an allophanic soil . Pest. Man. Sci. , 59 : 893 – 903 .
- Shahid , I. B. and Teoh , S. S. 1994 . Persistence of terbuthylazine in soils . Bull. Environ. Contamin. Toxicol. , 52 : 226 – 230 .
- Nordmeyer , H. and Pestemer , W. 1995 . Laboratory degradation and sorption experiments of pesticides in soil profiles . Z. Kulturtechnik. Laudentwicklung. , 36 : 6 – 10 .
- Dibbern , H. and Pestemer , W. 1992 . Application of simulation models for the leaching behaviour of pesticides in soils . Machrichtenblatt. des Deutschen. Pflanzenschutzdienstes. , 44 : 134 – 143 .
- Günther , P. , Pestmer , W. , James , T. K. and Rahman , A. 1993 . Testing of the German advisory system HERBASYS under different edaphical climate conditions in New Zealand . Proceedings of the 8th European Weed Research Society Symposium . June 14–16 1993 . pp. 777 – 784 . Braunschweig, , Germany : European Weed Research Society .
- Madhun , Y. A. and Freed , V. H. 1987 . Degradation of the herbicides bromacil, diuron, and chlortoluron in soil . Chemosphere , 16 : 1003 – 1011 .
- Sattar , M. A. 1990 . Fate of organophosphorous pesticides in soils . Chemosphere , 20 : 387 – 396 .
- Wauchope , R. D. , Buttler , T. M. , Hornsby , A. G. , Augustijn-Beckers , P. W. M. and Burt , J. P. 1992 . The SCS/ARS/CES Pesticide properties database for environmental decision making . Rev. Environ. Contamin. Toxicol. , 123 : 1 – 164 .
- McLaughlin , R. A. , Ross , R. H. and Balu , K. 1993 . Diazinon degradation and dissipation in the root zone , 43 – 64 . Madison, WI : SSSA . SSSA Spec. Publ. 32
- Racke , K. D. 1992 . “ Degradation of organophosphorus insecticides in environmental matrices ” . In Organophosphates, Chemistry, Fate, and Effects , Edited by: Chambers , J. E. and Levi , P. E. 47 – 73 . San Diego : Academic Press .
- Calderón , M. J. , Ortega , M. , Hermosin , Garcia-Baudin , J. and Cornejo , J. 2004 . Hexazinone and simazine dissipation in forestry field nurseries . Chemosphere , 54 : 1 – 8 .
- Neary , D. G. , Bush , P. B. and Douglass , J. E. 1983 . Off-site movement of hexazinone in stormflow and baseflow from forest watershed . Weed. Sci. , 31 : 543 – 551 .
- Bouchard , D. C. , Lavy , T. L. and Lawson , E. R. 1985 . Mobility and persistence of hexazinone in a forested watershed . J. Environ. Qual. , 14 ( 2 ) : 229 – 233 .
- Michael , J. L. 1990 . Fate of hexazinone after application for pine planting site preparation , Wilmington, DE : E. I. Du Pont de Nelours and Co. . Department No. AMR 1786-90. unpublished study. DPR # 396–062
- Holland , P. T. , Heiermann , M. , Rahman , A. , James , T. K. and McNaughton , D. E. Prediction of pesticide fate in soil and water , Raukura, NZ : Hart Reasearch . Report to MAF Policy on Operational Research Project FRM/01. 1988, Hort Research Client Report No. 98/146
- Kah , M. , Beulke , S. and Brown , C. D. 2007 . Factors influencing degradation of pesticides in soil . J. Agric. Food. Chem. , 55 : 4487 – 4492 .