Abstract
Despite rigorous preclinical testing, clinical attrition rates in drug development remain high with drug-induced liver injury (DILI) remaining one of the most frequent causes of project failures. To understand DILI mechanisms, major efforts are put into the development of physiologically relevant cell models and culture paradigms with the aim to enhance preclinical to clinical result translation. While the majority of toxicogenomic studies have been based on cell lines, there are emerging trends toward the predominant use of stem cell-derived organoids and primary human hepatocytes in complex 3D cell models. Such studies have been successful in disentangling diverse toxicity mechanisms, including genotoxicity, mitochondrial injury, steatogenesis and cholestasis and can aid in distinguishing hepatotoxic from nontoxic structural analogs. Furthermore, by leveraging inter-individual differences of cells from different donors, these approaches can emulate the complexity of polygenic risk scores, which facilitates personalized drug-specific DILI risk analyses. In summary, toxicogenomic studies into drug-induced hepatotoxicity have majorly contributed to our mechanistic understanding of DILI and the incorporation of organotypic human 3D liver models into the preclinical testing arsenal promises to enhance biological insights during drug discovery, increase confidence in preclinical safety and minimize the translational gap.
Introduction
Drug-induced liver injury (DILI) constitutes an important phenomenon in drug development and clinical practice. DILI is idiosyncratic and rare with DILI risk estimates for individual medications ranging from 1 in 10,000 to 1 in 100,000 individuals upon exposure (Larrey Citation2002; de Abajo et al. Citation2004; Navarro and Senior Citation2006; Bell and Chalasani Citation2009; Björnsson Citation2010). Similar results were obtained for liver injury due to herbal or dietary supplements (Navarro et al. Citation2017). Overall, DILI accounts for 52% of acute liver failure cases of which around 75% are due to intentional and unintentional acetaminophen overdoses (Ostapowicz et al. Citation2002). Excluding acetaminophen, overall DILI incidence at the population level is considerable with crude annual incidence rates in the general population of 1 in 5,000 to 1 in 10,000 (Sgro et al. Citation2002; Bjornsson et al. Citation2013). In addition to its clinical relevance, hepatotoxicity constitutes a major hurdle in drug development and remains a leading cause of drug failure during both preclinical and clinical stages (Watkins Citation2011; Cook et al. Citation2014). Furthermore, hepatotoxicity constitutes the most common reason of drug withdrawals in post-marketing stages accounting for 81 medicinal products between 1953 and 2014 out of a total of 462 withdrawals (18%) (Onakpoya et al. Citation2016).
Importantly, prediction of DILI propensity for a new compound is difficult using animal models due to extensive species-specific differences in expression patterns, isoform composition, and activities of drug metabolizing enzymes and drug transporters (Martignoni et al. Citation2006; Chu et al. Citation2013). Consequently, in vitro models using human liver cells are integral, well-established tools for a multitude of toxicological and pharmacological applications in drug development, including the identification of hepatotoxic liabilities and the delineation of the underlying toxicity mechanisms ( and Lauschke et al. Citation2016; Lin and Khetani Citation2016; Kuna et al. Citation2018; Lauschke et al. Citation2019; Zhou et al. Citation2019). In the past decade, there has been a growing body of literature utilizing comprehensive expression profiling of human liver cell systems to investigate hepatotoxicity mechanisms. While most studies are based on the hepatoma cell lines HepG2 and HepaRG, there is an increasing number of studies evaluating toxicity in stem cell-derived hepatocyte-like cells (HLC) and primary human hepatocytes (PHH) in conventional 2D monolayers and, more recently, in 3D cultures ().
Figure 1. 3D culture models of primary human liver cells constitute versatile tools for a multitude of applications in toxicology and pharmacology. Different 3D culture paradigms have been presented that are compatible with the culture of human hepatocytes or hepatocyte-like cells in mono-culture or in co-culture with non-parenchymal liver cells (NPCs). Figure modified with permission from Lauschke et al. (Citation2019).
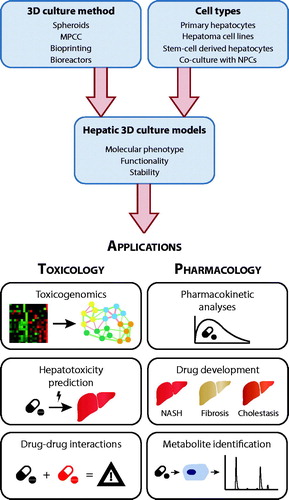
Table 1. Advantages and limitations of commonly used human hepatic cell models for toxicological studies.
HepG2 cells
HepG2 cells have been the predominant cell model for toxicogenomic analyses. While phenotypes and sensitivity to toxic insults differ drastically between HepG2 and primary human liver cells (Wilkening et al. Citation2003; Hart et al. Citation2010; Gerets et al. Citation2012; Sison-Young et al. Citation2017), these cell models have nevertheless proved useful for transcriptomics-based hepatotoxicity testing. By comparing gene expression patterns in HepG2 cells exposed to 13 Ames test-positive genotoxic compounds with 21 controls, transcriptomic signatures were identified that were highly predictive for genotoxicity with 89% accuracy and 91% specificity using an additional independent test set of 28 chemicals (Magkoufopoulou et al. Citation2012). Gene expression profiling moreover allowed to distinguish between carcinogens that activated the oxidative stress response and those that acted via different mechanisms (Deferme et al. Citation2015). Transcriptomics were similarly successful to identify a gene expression signature of 36 genes that was sufficient to accurately (91% accuracy) flag molecules with hepatotoxic liability on a small validation panel of 11 compounds (8 hepatotoxins and 3 nontoxic controls; ) and to sub-classify compounds into cholestatic and non-cholestatic mechanisms () (Van den Hof et al. Citation2014). Interestingly, hepatotoxins that synergized with pro-inflammatory cytokine signaling, such as diclofenac and carbamazepine, caused dysregulation of endoplasmic reticulum (ER) stress and Nrf2-mediated antioxidant signaling in HepG2 cells, which aligned well with data from 80 drugs in PHH (Fredriksson et al. Citation2014). In contrast, compounds whose toxicity was inflammation-independent, including ketoconazole, nefazodone, and methotrexate, did not perturb these pathways.
Figure 2. Toxicogenomic studies in human hepatic cells can identify hepatotoxic compounds and delineate underlying toxicity mechanisms. (a) Heatmap showing a gene expression signature of 36 genes in HepG2 cells that can distinguish hepatotoxic from non-hepatotoxic compounds. The signature was identified in the training set and replicated reasonably well in a separate set of validation compounds. (b) Furthermore, using a separate signature of 12 genes, HepG2 cells could distinguish between cholestatic and non-hepatotoxic compounds. Panels a and b were adapted with permission from Van den Hof et al. (Citation2014). Copyright 2014 American Chemical Society. (c) Heatmap visualization of a gene expression signature in patient-derived HLCs from three patients sensitive (Sens1-3) and two patients tolerant (Tol1-2) to pazopanib. Transcripts highlighted in red TFRC and SPINK1 are related to iron metabolism. (d) Gene set enrichment analysis (GSEA) for genes related to oxidative stress (top panel) or iron metabolism (bottom panel) in cells for which pazopanib was toxic (HT PZ) compared to nontoxic (NHT PZ). ES = enrichment score. Panels c and d were modified with permission from Choudhury et al. (Citation2017). (e) Venn diagram showing the number of differentially expressed genes (FDR < 0.05) in primary human hepatocyte spheroids exposed to hepatotoxins with different toxicity mechanisms compared to vehicle controls. GSEA indicates compound-specific and overlapping toxicity responses. Panel e modified with permission from Bell et al. (Citation2017).
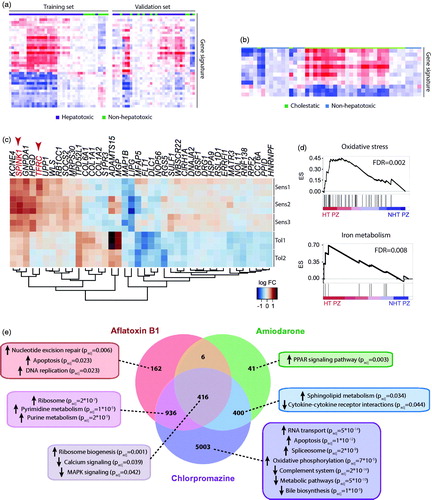
Besides overall patterns of toxicity, HepG2 cells have proven useful for the elucidation of the toxic mechanisms for candidate drugs of interest. Analyses of the gene expression changes caused by the genotoxic agent cisplatin revealed regulatory networks controlled by HNF4A, SP1, MYC and p53 that were conserved across species and cell models (human HepG2 cells, mouse primary hepatocytes and embryonic stem cells) (Rieswijk et al. Citation2014). Similarly, HepG2 cells revealed upregulation of SOS1 and oncogenic RAS as an important mechanism underlying toxicity of the non-genotoxic carcinogen 2,3,7,8-tetrachlorodibenzo-p-dioxin (TCDD) (Jennen et al. Citation2011).
In addition to carcinogens, toxicogenomic studies have contributed to the mechanistic understanding of acetaminophen (APAP) toxicity. By exploiting the fact that HepG2 cells do not express CYP2E1, the enzyme responsible for formation of the reactive APAP metabolite N-acetyl-p-benzoquinone imine (NAPQI), these cells provided a paradigm that allowed the investigation of NAPQI-independent mechanisms of APAP toxicity. Specifically, dysregulated expression of genes encoding the subunits of electron transport chain complexes and downregulation of the mitochondrial ROS scavenger SOD2 were identified as mechanisms underlying APAP-induced oxidative stress (Jiang et al. Citation2015).
In recent years, comprehensive mechanistic evaluations of toxicity in HepG2 cells have extended beyond the use of transcriptomics. Using Maldi-TOF proteomics, van Summeren and colleagues identified ER stress and perturbed ER-Golgi transport as a potential mechanism underlying the hepatotoxicity of cyclosporine A (Van Summeren et al. Citation2011). Similarly, epigenomic and metabolomic analyses revealed persistent patterns of altered DNA methylation upon aflatoxin B1 exposure (Rieswijk et al. Citation2016) and dose-dependent metabolomic alterations as indicators of hepatotoxicity using a panel of 35 test substances (Ramirez et al. Citation2018).
HepaRG cells
HepaRG cells are bipotent hepatic progenitor cells that can be differentiated into cells with hepatocyte-like and biliary-like epithelial morphology and phenotypes (Parent et al. Citation2004). Upon differentiation, they are generally considered to better reflect human hepatocyte phenotypes and function compared to HepG2 cells (Hart et al. Citation2010; Jennen et al. Citation2010; Gerets et al. Citation2012; Sison-Young et al. Citation2017). Specifically, HepaRG cell differentiation results in proliferative quiescence and the expression of key drug metabolizing enzymes and transporters at much higher levels than in HepG2 cells (Jennen et al. Citation2010). Nevertheless, much fewer studies have used HepaRG cells for toxicogenomic evaluations. Using a cost-effective transcriptomic assay with reduced complexity (profiling of 2839 genes) in HepaRG cells exposed to six different drugs, it was possible to discriminate dose-dependent and compound-specific hepatotoxicity signatures (Limonciel et al. Citation2018). Specifically, these experiments confirmed mitochondrial injury and ER perturbations upon cyclosporine A exposure mediated by ATF4 gene activation. Similarly, valproic acid activated the NRF2 pathway in alignment with its mechanism of liver injury that involves elevated ROS levels due to mitochondrial dysfunction. Furthermore, by combining gene expression profiling of 47 genes with proteomics, candidate markers for injury of 30 different pesticides were identified already at subtoxic concentrations (Braeuning et al. Citation2020). However, whether those can systematically optimize liver safety assessments remains to be determined.
HepaRG cells have also proven useful for the mechanistic evaluation of compound-specific liver toxicity. Using integrative transcriptomic, proteomic, and metabolomic profiling, bosentan was shown to inhibit the bile salt export pump (BSEP), activate FXR signaling, and induce oxidative stress and inflammation (Rodrigues et al. Citation2018). Similar integrative approaches revealed that inhibition of fatty acid metabolism and activation of AhR signaling underlie the toxicity pathway of the chemical pollutant PCB126 at toxicologically relevant picomolar concentrations (Mesnage et al. Citation2018).
Stem cell-derived hepatocyte-like cells
Human induced pluripotent stem cells (iPSCs) constitute a promising cell source that upon differentiation could provide an unlimited supply of liver cells. Particularly promising is the possibility to generate HLCs from individuals with specific genetic predispositions, such as genetic diseases or major histocompatibility complex (MHC) variants. Importantly however, while differentiation protocols have been substantially improved in recent years, current stem cell-derived liver systems still do not allow to closely recapitulate mature hepatic phenotypes and functions (Schwartz et al. Citation2014; Messina et al. Citation2020). Consequently, despite increasing use as cell models for toxicity testing (Wills and Rajagopalan Citation2020), only few toxicogenomic studies have been published that use HLCs for mechanistic evaluations.
A comparative study using HLCs, HepaRG, HepG2 cells, as well as PHH investigated expression signature alterations upon exposure to acetaminophen across cell models (Rodrigues et al. Citation2016). The authors use an acute toxicity setting with sub-toxic concentrations, i.e. acetaminophen concentrations that cause 10% cell death after 24 h of exposure (IC10). Notably, IC10 values differed drastically between models with HLCs being least sensitive (IC10, HLC of 18 mM, which is approximately 18-fold higher than concentrations resulting in fulminant liver failure in vivo); however, all 2D models aligned overall poorly with transcriptomic signatures of in vivo acute liver failure samples.
In an interesting study into the hepatotoxicity mechanisms of the tyrosine kinase inhibitor pazopanib, Choudhury and colleagues established iPSC lines from two and three patients treated with identical doses that tolerated pazopanib or experienced pazopanib-induced hepatotoxicity, respectively (Choudhury et al. Citation2017). Upon differentiation, HLCs from pazopanib-sensitive individuals showed higher pazopanib toxicity, whereas no differences were observed for acetaminophen as reference compound. Transcriptomic and functional analyses revealed that pazopanib depleted glutathione and increased levels of reactive oxygen species in all samples while further causing specific alterations of genes related to iron homeostasis and iron metabolism in susceptible-HLCs (), thus providing a nice demonstration of the power of stem cell derived liver models in linking clinical phenotypes to patient-specific molecular alterations.
Primary human hepatocytes
Primary hepatocytes are considered the gold standard for in vitro hepatotoxicity testing and their use is becoming more and more common. A multitude of studies have evaluated toxicogenomic responses in conventional 2D PHH cultures. One prominent example of the utility of this system is the identification of expression changes of genes involved in mitochondrial damage, RNA processing, transcription, and inflammation that clearly distinguish the idiosyncratic hepatotoxin trovafloxacin from structurally related nontoxic fluoroquinolones (Liguori et al. Citation2005; Citation2008). Similarly, PHH monolayer cultures have provided insights into the toxicity mechanisms of cyclosporine A (Wolters et al. Citation2016), diclofenac (Sarkar et al. Citation2017), valproic acid (Wolters et al. Citation2018) and triazole antifungals (Goetz and Dix Citation2009). Furthermore, by leveraging transcriptomic data from the Open TG-GATES repository, a toxicogenomic database containing data from PHH exposed to 158 compounds, NRF2 activation was identified as a good indicator of the intrinsic biochemical reactivity of a compound which remains an important component for the comprehensive evaluation of compound systems toxicology (Copple et al. Citation2019).
Notably however, in conventional 2D monolayer cultures, PHH very rapidly lose their mature characteristics with first gene expression changes being detectable as early as 30 min after the start of culture (Lauschke et al. Citation2016), which likely impacts toxicogenomic responses. Consequently, organotypic 3D cell culture methods that prevent or ameliorate this dedifferentiation have become increasingly prevalent. Comprehensive toxicity screens in spheroids and micropatterned co-cultures (MPCCs) using hundreds of compounds have demonstrated that PHH in 3D culture have high predictive accuracy (Khetani et al. Citation2013; Proctor et al. Citation2017; Vorrink et al. Citation2018). Furthermore, due to the extended viability and phenotypic stability that allows for chronic exposure studies for multiple weeks, the sensitivity of such 3D systems exceeds those of cells from the same donors cultured in 2D monolayers or 2D sandwich cultures (Bell et al. Citation2018).
In a toxicogenomic analysis of PHH in MPCC with stabilizing fibroblasts, differential gene expression analysis could distinguish hepatotoxic drugs troglitazone, nefazodone, ibufenac and tolcapone from their nontoxic structural analogues rosiglitazone, buspirone, ibuprofen and entacapone (Ware et al. Citation2017). Similarly, transcriptomic analyses of 3D spheroid cultures accurately identified the diverse toxicity mechanisms of aflatoxin B1 (genotoxicity), amiodarone (inhibition of mitochondrial respiration and steatogenesis) and chlorpromazine (cholestasis) based on gene expression and pathway activity differences () (Bell et al. Citation2017). Furthermore, the same study showed that the expression of biomarkers correlated well with the available in vivo data.
Conclusions and future perspectives
Driven in part by changes in legislation and perception of animal research, the multi-faceted advances in human cell culture methods and platforms have opened up new possibilities for the improved prediction and mechanistic analysis of DILI. However, important considerations remain. For instance, current culture models rarely embrace the inter-individual variability of human livers, as cell lines are derived from a single individual and studies using primary cells rarely use cells from more than five donors per study. While most clinically apparent cases of DILI are dose dependent and are primarily attributed to accidental or voluntary acetaminophen overdoses, the hepatotoxicity of most drugs is idiosyncratic and very rare, occurring only in 1 per 10,000 or fewer individuals. Consequently, only those toxic liabilities can be detected by toxicogenomic analyses where altered gene expression modules manifest in all or most individuals, though few would have experienced clinically manifest liver injury.
Along these lines, an interesting recent study developed a polygenic risk score for the susceptibility to DILI based on previous large-scale genome-wide association studies (Koido et al. Citation2020). Using primary hepatocytes and stem cell-derived organoids from multiple donors treated with over ten different drugs, this approach could flag individuals at risk of DILI, which can inform preclinical studies as well as patient selection in clinical trials. In conclusion, organotypic culture methods coupled with comprehensive multi-dimensional molecular profiling and an increasing understanding and appreciation of the polygenic architecture of DILI predisposition promises to improve early hepatotoxic risk prediction and reduce safety events in drug development.
Acknowledgments
The laboratory acknowledges support by Merck KGaA and Eli Lilly and Company.
Disclosure statement
V.M.L. is CEO and shareholder of HepaPredict AB, as well as co-founder and chairman of the board of PersoMedix AB. Furthermore, V.M.L. discloses consultancy work for Enginzyme AB.
Additional information
Funding
References
- Bell CC, Dankers ACA, Lauschke VM, Sison-Young R, Jenkins R, Rowe C, Goldring CE, Park K, Regan SL, Walker T, et al. 2018. Comparison of hepatic 2D sandwich cultures and 3D spheroids for long-term toxicity applications: a multicenter study. Toxicol Sci. 162(2):655–666.
- Bell CC, Lauschke VM, Vorrink SU, Palmgren H, Duffin R, Andersson TB, Ingelman-Sundberg M. 2017. Transcriptional, functional, and mechanistic comparisons of stem cell-derived hepatocytes, HepaRG cells, and three-dimensional human hepatocyte spheroids as predictive in vitro systems for drug-induced liver injury. Drug Metab Dispos. 45(4):419–429.
- Bell L, Chalasani N. 2009. Epidemiology of idiosyncratic drug-induced liver injury. Semin Liver Dis. 29(4):337–347.
- Björnsson E. 2010. Review article: drug-induced liver injury in clinical practice. Aliment Pharmacol Ther. 32(1):3–13.
- Bjornsson ES, Bergmann OM, Björnsson HK, Kvaran RB, Olafsson S. 2013. Incidence, presentation, and outcomes in patients with drug-induced liver injury in the general population of iceland. Gastroenterology. 144(7):1419–1425.
- Braeuning A, et al. 2020. RNA-protein correlation of liver toxicity markers in HepaRG cells. EXCLI J. 19:135–153.
- Choudhury Y, Toh YC, Xing J, Qu Y, Poh J, Li H, Tan HS, Kanesvaran R, Yu H, Tan M-H, et al. 2017. Patient-specific hepatocyte-like cells derived from induced pluripotent stem cells model pazopanib-mediated hepatotoxicity. Sci Rep. 7:46391.
- Chu X, Bleasby K, Evers R. 2013. Species differences in drug transporters and implications for translating preclinical findings to humans. Expert Opin Drug Metab Toxicol. 9(3):237–252.
- Cook D, Brown D, Alexander R, March R, Morgan P, Satterthwaite G, Pangalos MN. 2014. Lessons learned from the fate of AstraZeneca’s drug pipeline: a five-dimensional framework. Nat Rev Drug Discov. 13(6):419–431.
- Copple IM, den Hollander W, Callegaro G, Mutter FE, Maggs JL, Schofield AL, Rainbow L, Fang Y, Sutherland JJ, Ellis EC, et al. 2019. Characterisation of the NRF2 transcriptional network and its response to chemical insult in primary human hepatocytes: implications for prediction of drug-induced liver injury. Arch Toxicol. 93(2):385–399.
- de Abajo FJ, Montero D, Madurga M, García Rodríguez LA. 2004. Acute and clinically relevant drug-induced liver injury: a population based case-control study. Br J Clin Pharmacol. 58(1):71–80.
- Deferme L, Wolters J, Claessen S, Briedé J, Kleinjans J. 2015. Oxidative stress mechanisms do not discriminate between genotoxic and nongenotoxic liver carcinogens. Chem Res Toxicol. 28(8):1636–1646.
- Fredriksson L, Wink S, Herpers B, Benedetti G, Hadi M, de Bont H, Groothuis G, Luijten M, Danen E, de Graauw M, et al. 2014. Drug-induced endoplasmic reticulum and oxidative stress responses independently sensitize toward TNFα-mediated hepatotoxicity. Toxicol Sci. 140(1):144–159.
- Gerets HHJ, Tilmant K, Gerin B, Chanteux H, Depelchin BO, Dhalluin S, Atienzar FA. 2012. Characterization of primary human hepatocytes, HepG2 cells, and HepaRG cells at the mRNA level and CYP activity in response to inducers and their predictivity for the detection of human hepatotoxins. Cell Biol Toxicol. 28(2):69–87.
- Goetz AK, Dix DJ. 2009. Toxicogenomic effects common to triazole antifungals and conserved between rats and humans. Toxicol Appl Pharmacol. 238(1):80–89.
- Hart SN, Li Y, Nakamoto K, Subileau E-a, Steen D, Zhong X-b. 2010. A comparison of whole genome gene expression profiles of HepaRG cells and HepG2 cells to primary human hepatocytes and human liver tissues. Drug Metab Dispos. 38(6):988–994.
- Jennen D, Ruiz-Aracama A, Magkoufopoulou C, Peijnenburg A, Lommen A, van Delft J, Kleinjans J. 2011. Integrating transcriptomics and metabonomics to unravel modes-of-action of 2,3,7,8-tetrachlorodibenzo-p-dioxin (TCDD) in HepG2 cells. BMC Syst Biol. 5:139
- Jennen DGJ, Magkoufopoulou C, Ketelslegers HB, van Herwijnen MHM, Kleinjans JCS, van Delft JHM. 2010. Comparison of HepG2 and HepaRG by whole-genome gene expression analysis for the purpose of chemical hazard identification. Toxicol Sci. 115(1):66–79.
- Jiang J, Briedé JJ, Jennen DGJ, Van Summeren A, Saritas-Brauers K, Schaart G, Kleinjans JCS, de Kok TMCM. 2015. Increased mitochondrial ROS formation by acetaminophen in human hepatic cells is associated with gene expression changes suggesting disruption of the mitochondrial electron transport chain. Toxicol Lett. 234(2):139–150.
- Khetani SR, Kanchagar C, Ukairo O, Krzyzewski S, Moore A, Shi J, Aoyama S, Aleo M, Will Y. 2013. Use of micropatterned cocultures to detect compounds that cause drug-induced liver injury in humans. Toxicol Sci. 132(1):107–117.
- Koido M, Kawakami E, Fukumura J, Noguchi Y, Ohori M, Nio Y, Nicoletti P, Aithal GP, Daly AK, Watkins PB, et al. 2020. Polygenic architecture informs potential vulnerability to drug-induced liver injury. Nat Med. 26(10):1541–1548.
- Kuna L, Bozic I, Kizivat T, Bojanic K, Mrso M, Kralj E, Smolic R, Wu GY, Smolic M. 2018. Models of Drug Induced Liver Injury (DILI) – current issues and future perspectives. Curr Drug Metab. 19(10):830–838.
- Larrey D. 2002. Epidemiology and individual susceptibility to adverse drug reactions affecting the liver. Semin Liver Dis. 22(2):145–155.
- Lauschke VM, Hendriks DFG, Bell CC, Andersson TB, Ingelman-Sundberg M. 2016. Novel 3D culture systems for studies of human liver function and assessments of the hepatotoxicity of drugs and drug candidates. Chem Res Toxicol. 29(12):1936–1955.
- Lauschke VM, Shafagh RZ, Hendriks DFG, Ingelman-Sundberg M. 2019. 3D primary hepatocyte culture systems for analyses of liver diseases, drug metabolism, and toxicity: emerging culture paradigms and applications. Biotechnol J. 14(7):e1800347.
- Lauschke VM, Vorrink SU, Moro SML, Rezayee F, Nordling Å, Hendriks DFG, Bell CC, Sison-Young R, Park BK, Goldring CE, et al. 2016. Massive rearrangements of cellular MicroRNA signatures are key drivers of hepatocyte dedifferentiation. Hepatology. 64(5):1743–1756.
- Liguori MJ, Anderson MG, Bukofzer S, McKim J, Pregenzer JF, Retief J, Spear BB, Waring JF. 2005. Microarray analysis in human hepatocytes suggests a mechanism for hepatotoxicity induced by trovafloxacin. Hepatology. 41(1):177–186.
- Liguori MJ, Blomme EAG, Waring JF. 2008. Trovafloxacin-induced gene expression changes in liver-derived in vitro systems: comparison of primary human hepatocytes to HepG2 cells. Drug Metab Dispos. 36(2):223–233.
- Limonciel A, Ates G, Carta G, Wilmes A, Watzele M, Shepard PJ, VanSteenhouse HC, Seligmann B, Yeakley JM, van de Water B, et al. 2018. Comparison of base-line and chemical-induced transcriptomic responses in HepaRG and RPTEC/TERT1 cells using TempO-Seq. Arch Toxicol. 92(8):2517–2531.
- Lin C, Khetani SR. 2016. Advances in engineered liver models for investigating drug-induced liver injury. BioMed Res Int . 2016:1–20.
- Magkoufopoulou C, Claessen SMH, Tsamou M, Jennen DGJ, Kleinjans JCS, van Delft JHM. 2012. A transcriptomics-based in vitro assay for predicting chemical genotoxicity in vivo. Carcinogenesis. 33(7):1421–1429.
- Martignoni M, Groothuis GMM, de Kanter R. 2006. Species differences between mouse, rat, dog, monkey and human CYP-mediated drug metabolism, inhibition and induction. Expert Opin Drug Metab Toxicol. 2(6):875–894.
- Mesnage R, Biserni M, Balu S, Frainay C, Poupin N, Jourdan F, Wozniak E, Xenakis T, Mein CA, Antoniou MN, et al. 2018. Integrated transcriptomics and metabolomics reveal signatures of lipid metabolism dysregulation in HepaRG liver cells exposed to PCB 126. Arch Toxicol. 92(8):2533–2547.
- Messina A, Luce E, Hussein M, Dubart-Kupperschmitt A. 2020. Pluripotent-stem-cell-derived hepatic cells: hepatocytes and organoids for liver therapy and regeneration. Cells. 9(2):420.
- Navarro VJ, Khan I, Björnsson E, Seeff LB, Serrano J, Hoofnagle JH. 2017. Liver injury from herbal and dietary supplements. Hepatology. 65(1):363–373.
- Navarro VJ, Senior JR. 2006. Drug-related hepatotoxicity. N Engl J Med. 354(7):731–739.
- Onakpoya IJ, Heneghan CJ, Aronson JK. 2016. Post-marketing withdrawal of 462 medicinal products because of adverse drug reactions: a systematic review of the world literature. BMC Med. 14:10–11.
- Ostapowicz G, Fontana RJ, Schiødt FV, Larson A, Davern TJ, Han SHB, McCashland TM, Shakil AO, Hay JE, Hynan L, et al. 2002. Results of a prospective study of acute liver failure at 17 tertiary care centers in the United States. Ann Intern Med. 137(12):947–954.
- Parent R, Marion M-J, Furio L, Trepo C, Petit M-A. 2004. Origin and characterization of a human bipotent liver progenitor cell line. Gastroenterology. 126(4):1147–1156.
- Proctor WR, Foster AJ, Vogt J, Summers C, Middleton B, Pilling MA, Shienson D, Kijanska M, Ströbel S, Kelm JM, et al. 2017. Utility of spherical human liver microtissues for prediction of clinical drug-induced liver injury. Arch Toxicol. 91(8):2849–2863.
- Ramirez T, Strigun A, Verlohner A, Huener H-A, Peter E, Herold M, Bordag N, Mellert W, Walk T, Spitzer M, et al. 2018. Prediction of liver toxicity and mode of action using metabolomics in vitro in HepG2 cells. Arch Toxicol. 92(2):893–906.
- Rieswijk L, Claessen SMH, Bekers O, van Herwijnen M, Theunissen DHJ, Jennen DGJ, de Kok TMCM, Kleinjans JCS, van Breda SGJ. 2016. Aflatoxin B1 induces persistent epigenomic effects in primary human hepatocytes associated with hepatocellular carcinoma. Toxicology. 350-352:31–39.
- Rieswijk L, Lizarraga D, Brauers KJJ, Kleinjans JCS, van Delft JHM. 2014. Characterisation of cisplatin-induced transcriptomics responses in primary mouse hepatocytes, HepG2 cells and mouse embryonic stem cells shows conservation of regulating transcription factor networks. Mutagenesis. 29(1):17–26.
- Rodrigues RM, Heymans A, De Boe V, Sachinidis A, Chaudhari U, Govaere O, Roskams T, Vanhaecke T, Rogiers V, De Kock J, et al. 2016. Toxicogenomics-based prediction of acetaminophen-induced liver injury using human hepatic cell systems. Toxicol Lett. 240(1):50–59.
- Rodrigues RM, Kollipara L, Chaudhari U, Sachinidis A, Zahedi RP, Sickmann A, Kopp-Schneider A, Jiang X, Keun H, Hengstler J, et al. 2018. Omics-based responses induced by bosentan in human hepatoma HepaRG cell cultures. Arch Toxicol. 92(6):1939–1952.
- Sarkar U, Ravindra KC, Large E, Young CL, Rivera-Burgos D, Yu J, Cirit M, Hughes DJ, Wishnok JS, Lauffenburger DA, et al. 2017. Integrated assessment of diclofenac biotransformation, pharmacokinetics, and omics-based toxicity in a three-dimensional human liver-immunocompetent coculture system. Drug Metab Dispos. 45(7):855–866.
- Schwartz RE, Fleming HE, Khetani SR, Bhatia SN. 2014. Pluripotent stem cell-derived hepatocyte-like cells. Biotechnol Adv. 32(2):504–513.
- Sgro C, Clinard F, Ouazir K, Chanay H, Allard C, Guilleminet C, Lenoir C, Lemoine A, Hillon P. 2002. Incidence of drug-induced hepatic injuries: a French population-based study. Hepatology. 36(2):451–455.
- Sison-Young RL, Lauschke VM, Johann E, Alexandre E, Antherieu S, Aerts H, Gerets HHJ, Labbe G, Hoët D, Dorau M, et al. 2017. A multicenter assessment of single-cell models aligned to standard measures of cell health for prediction of acute hepatotoxicity. Arch Toxicol. 91(3):1385–1400.
- Van den Hof WFPM, Coonen MLJ, van Herwijnen M, Brauers K, Wodzig WKWH, van Delft JHM, Kleinjans JCS. 2014. Classification of hepatotoxicants using HepG2 cells: a proof of principle study. Chem Res Toxicol. 27(3):433–442.
- Van Summeren A, Renes J, Bouwman FG, Noben J-P, van Delft JHM, Kleinjans JCS, Mariman ECM. 2011. Proteomics investigations of drug-induced hepatotoxicity in HepG2 cells. Toxicol Sci. 120(1):109–122.
- Vorrink SU, Zhou Y, Ingelman-Sundberg M, Lauschke VM. 2018. Prediction of drug-induced hepatotoxicity using long-term stable primary hepatic 3D spheroid cultures in chemically defined conditions. Toxicol Sci. 163(2):655–665.
- Ware BR, McVay M, Sunada WY, Khetani SR. 2017. Exploring chronic drug effects on microengineered human liver cultures using global gene expression profiling. Toxicol Sci. 157(2):387–398.
- Watkins PB. 2011. Drug safety sciences and the bottleneck in drug development. Clin Pharmacol Ther. 89(6):788–790.
- Wilkening S, Stahl F, Bader A. 2003. Comparison of primary human hepatocytes and hepatoma cell line Hepg2 with regard to their biotransformation properties. Drug Metab Dispos. 31(8):1035–1042.
- Wills LR, Rajagopalan P. 2020. Advances in human induced pluripotent stem cell-derived hepatocytes for use in toxicity testing. Ann Biomed Eng. 48(3):1045–1057.
- Wolters JEJ, van Breda SGJ, Grossmann J, Fortes C, Caiment F, Kleinjans JCS. 2018. Integrated omics analysis reveals new drug-induced mitochondrial perturbations in human hepatocytes . Toxicol Lett. 289:1–13.
- Wolters JEJ, van Herwijnen MHM, Theunissen DHJ, Jennen DGJ, Van den Hof WFPM, de Kok TMCM, Schaap FG, van Breda SGJ, Kleinjans JCS. 2016. Integrative “-Omics” analysis in primary human hepatocytes unravels persistent mechanisms of cyclosporine A-induced cholestasis. Chem Res Toxicol. 29(12):2164–2174.
- Zhou Y, Shen JX, Lauschke VM. 2019. Comprehensive evaluation of organotypic and microphysiological liver models for prediction of drug-induced liver injury. Front Pharmacol. 10:1093.