Abstract
Sclerotinia sclerotiorum is a fungal pathogen that causes stem rot in oilseed rape (Brassica napus). Previously, B. napus accessions with partial stem resistance to a Canadian S. sclerotiorum isolate (#321) were identified using a stem test in which flowering plants were inoculated with mycelium plugs. The present study examined the partial stem resistance of four of these accessions, PAK54, PAK93, DC21 and K22, following inoculation with Australian isolates. Mycelial compatibility groups and intergenic spacer (IGS) region haplotypes were identified among 71 isolates from Australian oilseed rape and lupin fields. Eleven genetically diverse isolates showed differences in aggressiveness when inoculated onto nine oilseed rape varieties and one Chinese accession. Isolates CU8.24, CU10.17 and CU11.19 were selected based on genetic diversity, growth rate in vitro and high aggressiveness in the initial screen and subsequently inoculated onto the four B. napus accessions. These accessions developed significantly smaller lesions compared with the susceptible control varieties (‘AV Garnet’ and ‘Westar’), with the average frequency of soft and collapsed lesions being less than 20% in PAK54, DC21 and K22, 29% in PAK93 and greater than 88% in the susceptible controls. Microscopic examination revealed that hyphae were typically confined to the stem cortex in the smallest lesions, but could be found in the stem pith in larger lesions. These results show that B. napus accessions PAK54, PAK93, DC21 and K22 can be used in Australia for development of varieties with partial stem resistance to S. sclerotiorum.
Résumé
Sclerotinia sclerotiorum est un agent pathogène fongique qui cause la pourriture sclérotique chez le colza oléagineux (Brassica napus). Auparavant, les accessions de B. napus possédant une résistance partielle à la pourriture causée par l’isolat canadien de S. sclerotiorum (n° 321) étaient identifiées à l’aide d’un test spécifique de la pourriture au cours duquel les plants en fleur étaient inoculés avec des chevilles de mycélium. Cette étude examine la résistance partielle à la pourriture des quatre accessions suivantes, PAK54, PAK93, DC21 et K22, après inoculation avec des isolats australiens. Les groupes de compatibilité mycélienne et les haplotypes des régions des espaceurs intergéniques ont été identifiés chez 71 isolats provenant de champs de colza oléagineux et de lupin australiens. Onze isolats génétiquement différents ont affiché des variations quant à leur agressivité lorsqu’ils ont été utilisés pour inoculer neuf variétés de colza oléagineux et une accession chinoise. Les isolats CU8.24, CU10.17 et CU11.19 ont été choisis en fonction de leur diversité génétique, de leur taux de croissance in vitro et de leur énorme agressivité lors du criblage initial, puis ont servi à inoculer les quatre accessions de B. napus. Ces accessions ont développé des lésions particulièrement petites comparativement aux variétés témoins réceptives (‘AV Garnet’ et ‘Westar’), avec une fréquence moyenne de moins de 20% de lésions molles et affaissées chez PAK54, DC21 et K22, de 29% chez PAK93 et de plus de 88% chez les témoins réceptifs. L’examen microscopique a révélé que, en ce qui a trait aux plus petites lésions, les hyphes étaient généralement confinés au cortex des tiges, mais, en ce qui a trait plus grosses, il était possible de les trouver dans la moelle. Ces résultats montrent que les accessions PAK54, PAK93, DC21 et K22 de B. Napus peuvent être utilisées en Australie pour développer des variétés offrant une résistance partielle à S. sclerotiorum.
Introduction
Sclerotinia stem rot (SSR) of Brassica napus L. (oilseed rape) is caused by the ascomycete fungus Sclerotinia sclerotiorum (Lib.) De Bary. This disease is a major yield-limiting factor in countries that grow oilseed rape, including China, Canada, Australia and many European countries (Kharbanda & Tewari, Citation1996; Wang et al., Citation2009; Murray & Brennan, Citation2012). Stem infection is the main cause of yield loss and occurs via ascospore-infected petals that adhere to plants during flowering as described in a recent review by Derbyshire & Denton-Giles (Citation2016). Because SSR epidemics are highly dependent on rainfall leading up to and during the flowering period (Twengstrom et al., Citation1998; Koch et al., Citation2007), yield losses vary significantly from year to year (Del Rio et al., Citation2007), and have been reported in China to be as high as 80% (Wang et al., Citation2009). To manage the risk of SSR, growers primarily rely on crop rotation and prophylactic application of fungicides during flowering (Derbyshire & Denton-Giles, Citation2016). Over the last 30 years, world oilseed rape production has increased from 10 to 68 million tonnes (FAOSTAT, Citation2018), placing an increased strain on these management strategies. Development of commercial oilseed rape varieties with improved resistance to SSR would therefore benefit growers and the oilseed industry in general.
The majority of commercial oilseed rape varieties in Australia are susceptible to SSR (Uloth et al., Citation2015; Rana et al., Citation2017). However, a growing list of publications show that partial, quantitative resistance exists in B. napus germplasm and many of its close relatives (Mei et al., Citation2011; Uloth et al., Citation2013, Citation2015; Taylor et al., Citation2015; Rana et al., Citation2017). In some cases, resistance has been identified by inoculation of the main stem of adult plants (Buchwaldt et al., Citation2005; Gyawali et al., Citation2016). The resulting lesions on partially resistant genotypes are relative small with a dark brown to black border between healthy and infected tissue, and more importantly, the majority of the stem lesions remain firm (Buchwaldt et al., Citation2005; Gyawali et al., Citation2016). In contrast, susceptible lines develop typical whitish or tan coloured lesions that extend longitudinally as well as into the stem, resulting in soft and collapsed stems, which in the field can result in lodging of infected plants. Lignification of stem cortical cells has been observed during resistance responses to S. sclerotiorum infection and has been proposed as a mechanism that restricts the growth of the pathogen in stem tissue (Uloth et al., Citation2015, Citation2016).
It is well documented that S. sclerotiorum isolates vary in aggressiveness. Taylor et al. (Citation2015) tested the aggressiveness of 18 S. sclerotiorum isolates prior to selecting an aggressive and a weakly aggressive isolate for screening of 96 B. napus accessions. Differences in aggressiveness of S. sclerotiorum isolates have also been demonstrated for soybean (Glycine max (L.) Merr.) (Willbur et al., Citation2017). Careful considerations should therefore be made when selecting S. sclerotiorum isolates for resistance screening, since weakly aggressive isolates can increase the risk of false positive phenotypes.
The objectives of the present study were to: (i) identify genetically diverse and highly aggressive Australian isolates of S. sclerotiorum and (ii), to use these isolates to screen B. napus accessions previously identified as partially resistant to a Canadian isolate, #321 (Gyawali et al., Citation2016). Here we confirm that the four B. napus accessions, PAK93, PAK54, DC21 and K22, are partially resistant to highly aggressive isolates that represent the genetic diversity of the pathogen population in Western Australia. In addition, we describe the development of S. sclerotiorum in different stem tissues of these partially resistant accessions using light microscopy and various staining techniques.
Materials and methods
Plant material
A total of 15 B. napus varieties and accessions were used in this study (). To identify highly aggressive Australian S. sclerotiorum isolates, 11 isolates () were screened against germplasm set 1. The three most aggressive isolates (CU8.24, CU10.17 and CU11.19) were subsequently inoculated onto germplasm set 2, which included four B. napus accessions that have previously exhibited partial stem resistance to a Canadian S. sclerotiorum isolate (Gyawali et al., Citation2016). Seed of germplasm set 1 varieties were obtained from the Australian Grains Genebank (www.seedpartnership.org.au). Seeds of variety ‘Westar’ were obtained from the Brassica napus ERANET ASSYST population (Bus et al., Citation2011). The four partially resistant accessions, PAK54, PAK93, DC21 and K22, in germplasm set 2, were obtained from Agriculture and Agri-Food Canada, Saskatoon, Canada.
Table 1. Brassica napus accessions and varieties used in the present study.
Table 2. Australian Sclerotinia sclerotiorum isolates used in the present study.
Fungal isolates
In 2013 and 2014, 71 S. sclerotiorum isolates were collected from commercial fields of oilseed rape and lupin (Lupinus albus L.) in Western Australia where SSR is common. These isolates were part of a world collection of S. sclerotiorum isolates which was characterized by Clarkson et al. (Citation2017). One method involved sequencing of the intergenic spacer (IGS) region followed by identification of single nucleotide polymorphisms (SNP). This study showed that the Australian isolates represented three IGS-haplotypes, namely 3, 5 and 7 (). Following collection, a sclerotium of each isolate was surface sterilized in 70% ethanol for 1 min, washed in sterile water, blot-dried, cut in half and plated onto potato dextrose agar (PDA) (Becton Dickinson, Sparks, USA) using sterile techniques. Isolates were purified by hyphal tipping. Mature sclerotia that formed on the final subculture were dried and stored at 4°C. These sclerotia were used to regenerate isolate cultures when required.
Mycelial compatibility groups
Mycelial compatibility groups (MCG) were determined for the 71 isolates as previously described (Kohn et al., Citation1990; Sexton & Howlett, Citation2004; Sexton et al., Citation2006). In brief, the isolates were paired in all combinations on PDA amended with 75 μL L−1 of red food colouring (Queen Fine Foods, Brisbane, Australia). Incompatible interactions were identified by a barrage between their mycelium and a red line in the growth medium (Supplementary Fig. 3). All pairings were repeated at least twice. Based on IGS haplotype and mycelial compatibility testing, 11 genetically diverse Australian isolates were selected for in vitro growth assays and inoculation of B. napus germplasm set 1 ().
In vitro growth assays of S. sclerotiorum isolates
The 11 S. sclerotiorum isolates mentioned above were examined for two growth characteristics in vitro, namely colony diameter 48 h after transfer to PDA, and the ability of hyphae to acidify PDA medium supplemented with the pH-indicator bromophenol blue (50 mg L−1), which changes in colour from purple to yellow when the medium changes from pH 7 to pH 4 (Supplementary Fig. 1).
Sclerotinia screening of B. napus in germplasm set 1
Germplasm set 1 was screened for isolate aggressiveness and partial stem resistance using a method originally described by Buchwaldt et al. (Citation2005). At least three biological replicates (individual plants) were used for each isolate × variety/accession combination. Seeds were germinated in 5 cm diameter pots containing an all-purpose potting mix (UWA mix, Richgro, Perth, Australia) and incubated at 20°C with a 12 h light/dark cycle. After 3 weeks, seedlings were transplanted into 4 L pots and positioned in a greenhouse in a completely randomized design. Each pot was drip irrigated for 1 min every 2 days. Prior to bolting, each plant was fertilized with 2 g of Nitrophoska Perfkt™ fertilizer. Stem inoculations were performed once all accessions had reached 10% flowering, corresponding to growth stages 61–67 on the BBCH-scale (Lancashire et al., Citation1991). A 5 mm plug of actively growing S. sclerotiorum mycelium on PDA was placed on the main stem of each individual plant at the internode above the middle node and fixed in position with Parafilm. Plants of each variety/accession were also mock inoculated with PDA only. Infection was left to develop under ambient greenhouse conditions. Stem lesion length (mm) was measured at 12, 18 and 24 days post-inoculation (dpi). The area under the disease progression curve (AUDPC) was calculated using stem lesion length measurements. The AUDPC measurement incorporates temporal disease development information and is useful for determining quantitative resistance phenotypes (Simko & Hans-Peter, Citation2011). The overall aggressiveness of each isolate was determined by calculating the median AUDPC across all genotypes of germplasm set 1 (). Mean AUDPC data for each isolate × variety/accession combination is included in Supplementary Table 1. Significant differences were calculated by subjecting the raw, individual isolate × accession AUDPC data to one-way ANOVA and performing a Tukey’s Honest Significant Difference (HSD) post-hoc test (P ≤ 0.05).
Fig. 1 Area under the disease progress curve (AUDPC) using stem lesion length measurements for 10 B. napus entries (germplasm set 1) inoculated with 11 Australian Sclerotinia sclerotiorum isolates. Each box plot displays the median and the lower and upper quartiles for each isolate. Letters above bars represent significant differences between entries as determined by ANOVA and Tukey’s HSD post-hoc test (P ≤ 0.05). Isolate names tagged with an asterisk were selected for screening of B. napus germplasm set 2.
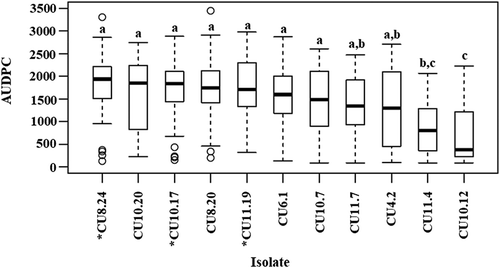
Sclerotinia screening of B. napus in germplasm set 2
Isolates CU8.24, CU10.17 and CU11.19 were used to screen germplasm set 2 () for partial stem resistance. The experiment was performed in a controlled environment using a stem inoculation method similar to that described by Buchwaldt et al. (Citation2005) and Gyawali et al. (Citation2016). Nine biological replicates (individual plants) were used for each isolate × variety/accession combination. Seeds were directly sown into 4 L pots containing an all-purpose potting mix (UWA mix, Richgro) and positioned in the growth room in a completely randomized design. Plants were grown under a diurnal cycle of 22°C with 16 h light (200 µmol m−1 s−2) and 20°C with 8 h dark at a relative humidity of 60%. Prior to bolting, each plant was fertilized with 2 g of Nitrophoska Perfkt™ fertilizer. Stem inoculations were performed when individual plants reached 50% flowering, corresponding to growth stage 65 on the BBCH-scale (Lancashire et al., Citation1991). Mycelium of each isolate was grown on glucose medium (20 g glucose, 2 g NH4NO3, 1 g KH2PO4, 0.1 g MgSO47H2, 3 g malic acid, 1 g NaOH, 20 g agar and 1000 mL distilled water). A 7 mm plug of actively growing S. sclerotiorum mycelium was placed on the middle internode of the main stem corresponding to the mid-point between the soil surface and the lowest floral branch and fixed in position with Parafilm. The temperature and humidity used for plant growth was maintained during the infection period. To be consistent with the method described by Gyawali et al. (Citation2016), stem lesion length (mm) was measured at 7, 14 and 21 dpi. At 21 dpi, the stem lesions were rated as collapsed, soft, firm and black. This corresponded to the following lesion colours: collapsed – whitish grey, soft – light grey, firm – brown, black – black. Sample images of infected stems were taken with a Canon PowerShot G5X camera at 21 dpi to show the differences between certain isolate × variety/accession combinations (Supplementary Fig. 2). The AUDPC was calculated for each isolate × variety/accession combination using stem lesion length measurements. Significant differences were calculated by subjecting the raw, individual data to one-way ANOVA. Tukey’s Honest Significant Difference (HSD) post-hoc test was used to determine significant differences between genotypes (P ≤ 0.005).
Macroscopic and microscopic examination of stem lesions
Macroscopic images of stem lesions were captured using a Canon PowerShot G5X camera. Subsequently, longitudinal and transverse sections were made through stem lesions of PAK54, PAK93, DC21 and K22 B. napus accessions. Lesions from susceptible control plants, ‘Westar’ and ‘AV Garnet’, were not sectioned due to extensive deterioration of the stem tissue. Sections were immersed in sterile water, and either stained with phloroglucinol-HCl (2 g phloroglucinol, 80 mL 20% ethanol, 20 mL conc. HCl) for 5 min, or fixed in 3 ethanol : 1 acetic acid overnight and stained with 0.01% trypan blue in lactophenol for 3 hours as previously described by Denton-Giles et al. (Citation2013). Following staining, tissues were washed in sterile water and mounted on glass slides in 40% glycerol. All samples were examined using a Nikon Eclipse E200 light microscope equipped with a Tucsen USB2.0 digital camera. Qualitative measurements of hyphal abundance in different stem tissues were made by examining three or more trypan blue-stained stem sections for each lesion sample.
Results
Genetic diversity of Australian S. sclerotiorum isolates
Of the 71 S. sclerotiorum isolates tested for vegetative compatibility, 46 paired with at least one other isolate, while 18 isolates were incompatible with all other isolates (Supplementary Fig. 3 and Supplementary Table 2). Seven of the isolates lost viability during testing. A total of 12 MCGs were identified and 11 were used for this study. Clarkson et al. (Citation2017) previously demonstrated that the 71 isolates described here grouped into three IGS haplotype groups, namely groups 3, 5 and 7. Notably, isolates within each of the 11 designated MCGs used in this study also grouped based on IGS-haplotype (Supplementary Table 2). Single isolates from each of the 11 MCGs that collectively included all three IGS haplotypes, were chosen for further in vitro and in planta analyses ().
A range of growth rates on PDA were observed for the 11 isolates, varying from 53.0 mm for isolate CU10.12 to 70.6 mm for isolate CU11.19 (Supplementary Fig. 1). All isolates were able to acidify the PDA medium from pH 7 to pH 4 as demonstrated by a colour change of pH-indicator bromophenol blue from purple to yellow (Supplementary Fig. 1).
Aggressiveness of Australian S. sclerotiorum isolates
The mean AUDPC values for each isolate × variety/accession combination in germplasm set 1 showed that the Australian S. sclerotiorum isolates were pathogenic on all nine Australian oilseed rape varieties and the Chinese accession (Supplementary Table 1), while mock inoculated plants did not show any symptoms. Significant differences in isolate aggressiveness were evident (). The five most aggressive isolates were CU8.24, CU10.20, CU10.17, CU8.20 and CU11.19, while CU10.12 and CU11.4 produced smaller lesions. Interestingly, these two isolates also had the slowest growth rate on PDA. Taken together, isolates CU8.24, CU10.17 and CU11.19 were highly aggressive on B. napus, demonstrated a rapid growth rate in vitro, and were genetically diverse. Therefore, these isolates were considered suitable for subsequent screening of B. napus accessions identified as partially resistant in a Canadian study (Gyawali et al., Citation2016).
Partial stem resistance of PAK54, PAK93, DC21 and K22 to S. sclerotiorum from Australia
In a previous study, partial stem resistance was demonstrated in B. napus accessions PAK54, PAK93, DC21 and K22 against a single Canadian S. sclerotiorum isolate, #321 (Gyawali et al., Citation2016). In the present study, the same four accessions were inoculated with three Australian isolates, CU8.24, CU10.17 and CU11.19, separately. AUDPC values calculated from stem lesion length on PAK54, PAK93, DC21 and K22 inoculated with each isolate were significantly smaller than on the susceptible controls, ‘Westar’ and ‘AV Garnet’ (). Collapsed lesions were not observed for any of the four accessions. Per cent soft lesions were 0% for both DC21 and K22, 11–22% for PAK54 and 11–44% for PAK93 (), confirming these accessions are partially resistant against highly aggressive S. sclerotiorum isolates from Australia. In contrast, ‘Westar’ and ‘AV Garnet’ had more than 88% soft and collapsed lesions for all three isolates. Sample images of infected stems for the isolate × variety/accession combinations are shown in Supplementary Fig. 3.
Fig. 2 (a) Area under the disease progress curve (AUDPC) using stem lesion length measurements for four Brassica napus accessions and two oilseed rape varieties, ‘Westar’ and ‘AV Garnet’ (germplasm set 2), inoculated with each of three Australian S. sclerotiorum isolates. Each box plot displays the median and the lower and upper quartiles. Letters above bars represent significant differences between entries as determined by ANOVA and Tukey’s HSD post-hoc test (P ≤ 0.005). (b) Stacked bar graph of stem lesion appearance 21 dpi; collapsed (white), soft (light grey), firm with small necrotic lesions (dark grey) and firm with small black lesions (black).
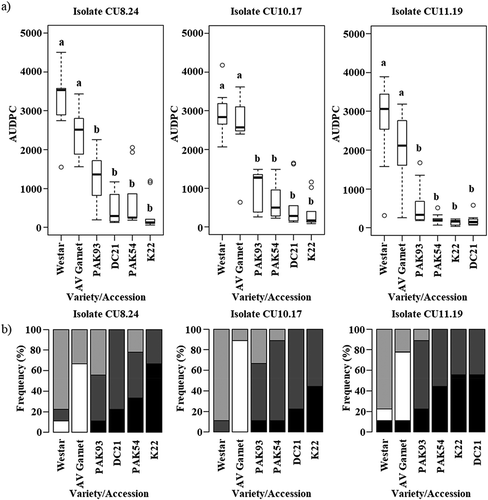
Stem cortex cells restrict growth of S. sclerotiorum
To further characterize the partial stem resistance response in inoculated stems of PAK54, PAK93, DC21 and K22, two stem lesions representing one small and one larger lesion were sectioned, photographed and examined macroscopically, between 35 and 51 dpi (). ‘Westar’ and ‘AV Garnet’ were not included due to extensive degradation of all stem tissues. Small lesions, less than 25 mm, were typically spherical or ovoid with a dark border between infected and healthy stem tissue (). Larger lesions between 25 and 30 mm were more elongated and often retained the dark border. Stem lesions were cut longitudinally in order to visualize the depth of symptoms. Smaller lesions were predominantly confined to the outer cortex of the stem, although a slight darkening of the pith was regularly observed. Larger lesions extended into the vascular ring and the pith was often discoloured. The dark border seen on the stem surface often extended internally into the stem ().
Fig. 3 (Colour online) Images of stem lesion development and qualitative analysis of S. sclerotiorum hyphal abundance in stem tissue of four partially resistant Brassica napus accessions (white scale bars = 25 mm). Microscopic images (×40) of sectioned and stained stem lesions. White dashed line shows the border between the cortex and vascular tissues (black scale bars = 100 µm). Observations of hyphal abundance in the cortex (co), vascular tissue (va) and stem pith (pi). (-) no hyphae, (+) few hyphae, (++) many hyphae (NA) no rating due to tissue maceration.
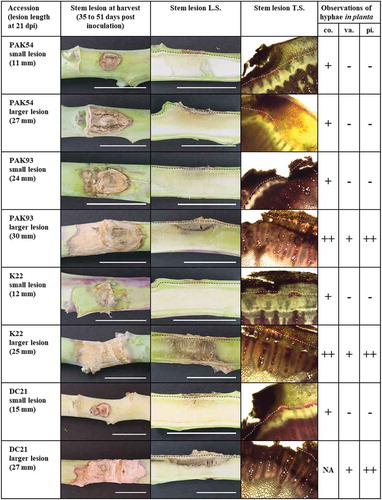
To relate the macroscopic observations described above to development of S. sclerotiorum hyphae, transverse sections through each stem lesion were made and stained. In small lesions, hyphae were observed in the cortex in proximity to the point of inoculation, but neither in the vascular tissue nor in the pith ( and ). Within larger lesions, hyphae were abundant in the cortex (), and observed at relatively low frequency in the vascular tissues of both the protoxylem and metaxylem (), but were dense and prolific in the pith (). Together these results show that the S. sclerotiorum resistance response in the stem resides primarily in the cortical tissue and to a lesser extent in the vascular tissue. Once the pathogen enters into the pith it creates a dense network of hyphae.
Fig. 4 (Colour online) Light micrographs showing S. sclerotiorum hyphae in stems of partially resistant B. napus accessions. Samples were stained with trypan blue in lactophenol. (a) Infected tissue layers are labelled with double-arrow lines, including cortex (co), vascular tissue (va) and pith (pi). Single-arrow lines show lesion (le), fibre cap (fc), phloem (ph), protoxylem (px) and metaxylem (mx). (b) Hyphae growing in the cortex of a stem lesion on K22. (c) Hyphae protruding from the protoxylem of a stem lesion on PAK93. (d) Hyphae growing laterally through the protoxylem ray cells, and (e) protruding from the metaxylem in K22. (f) Hyphae proliferating in the pith of DC21. Scale bar = 100 µm.
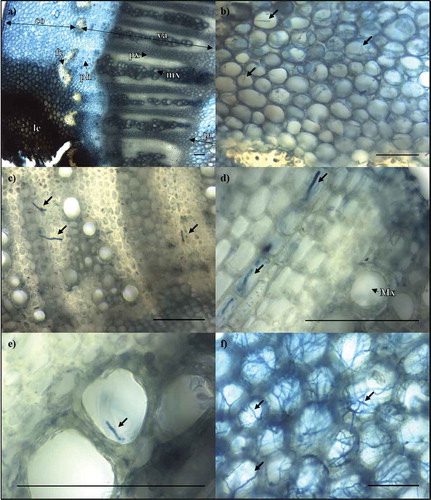
Discussion
Partial resistance to S. sclerotiorum has been reported in multiple crops including pea (Pisum sativum L.) (Porter, Citation2012), soybean (Bastien et al., Citation2012) and sunflower (Helianthus annuus L.) (Davar et al., Citation2010) and results in a significant reduction of disease as opposed to a total absence of disease, as reviewed by St. Clair (Citation2010). The present study showed that four B. napus accessions, PAK93, PAK54, DC21 and K22, have partial stem resistance against three aggressive and genetically diverse S. sclerotiorum isolates from Australia. The conclusions of this study are based on a rigorous stem inoculation assay that produced significantly smaller lesions than two susceptible control varieties, 21 days post inoculation. Lesions did not cause the stems to collapse in any of the accessions, but PAK93 had 29% soft lesions on average. The same four accessions were partially resistant to a single Canadian S. sclerotiorum isolate in a previous study (Gyawali et al., Citation2016). Partial stem resistance has since then been verified with more Canadian isolates (unpublished data).
Sclerotinia sclerotiorum infection of the B. napus stem causes the greatest damage to yield, as it restricts the primary flow of assimilate and water to the developing seeds. The pathogen can also infect leaves, petioles, upper branches and pods of B. napus. However, these infections do not normally cause major yield loss. The stem inoculation method used in this study was designed to closely mimic the manner in which S. sclerotiorum naturally infects B. napus stems in the field (Buchwaldt et al., Citation2005; Gyawali et al., Citation2016). Stem inoculations performed on ‘Westar’, ‘AV Garnet’, PAK93, PAK54, DC21 and K22 accessions utilized a minimal glucose medium to imitate petals as a nutrient source. Natural SSR field infection normally occurs during the B. napus flowering period (Twengstrom et al., Citation1998). Therefore, inoculations were performed on the main stem of flowering plants, similar to infection under field conditions. Versions of this method have been used extensively for screening B. napus germplasm for stem resistance (Garg et al., Citation2010; Uloth et al., Citation2013; Wu et al., Citation2013).
The 11 Australian S. sclerotiorum isolates used in the present study had different levels of aggressiveness on B. napus germplasm set 1. This permitted selection of the most aggressive isolates for screening of the partially resistant B. napus entries in germplasm set 2. It is well documented that S. sclerotiorum isolates from around the world vary in aggressiveness on plant species. Ge et al. (Citation2012) showed that isolates collected from B. napus in Australia varied in aggressiveness when inoculated back onto B. napus varieties. Taylor et al. (Citation2015) demonstrated that isolates collected from diverse plant species in the UK exhibited differences in aggressiveness when inoculated onto a set of B. napus varieties. Differences in aggressiveness have also been shown on other hosts, including dandelion (Taraxacum officinale L.) (Riddle et al., Citation1991), sunflower (Davar et al., Citation2011), peanut (Arachis hypogaea L.) (Lujan et al., Citation2016), eggplant (Solanum melongena) (Tok et al., Citation2016) and soybean (Willbur et al., Citation2017).
Macroscopic and microscopic examination of stem lesions demonstrated that the stem cortex of partially resistant accessions is able to restrict the growth of S. sclerotiorum. Contrastingly, when fungal hyphae were observed in the pith they were associated with the development of larger lesions. Uloth et al. (Citation2016) showed that stem cortex thickness was correlated with stronger partial stem resistance to S. sclerotiorum in B. napus. Furthermore, the stem cortex has also been implicated in restricting the growth of the blackleg pathogen [Leptosphaeria maculans (Desm.) Ces. et De Not] in some accessions of oilseed rape that have quantitative resistance to this disease (Huang et al., Citation2009, Citation2014). Since the stem cortex plays an important role in disease resistance, screening methods should avoid wounding of the stem tissue. In addition, it is advisable to inoculate B. napus stems at the same growth stage and internode, since cortex thickness can influence the size of stem lesions and the frequency of lesions that lead to collapsed and soft stem tissue.
Hyphae of S. sclerotiorum were observed in the vascular tissues of PAK93, DC21 and K22, but not in PAK54. It has previously been hypothesized that only susceptible genotypes of B. napus exhibit vascular invasion by S. sclerotiorum (Uloth et al., Citation2016). We clearly demonstrate that protoxylem and metaxylem cells of partially resistant B. napus accessions also can be invaded by S. sclerotiorum, confirming the notion that partial stem resistance can cause an array of phenotypes but primarily results in slowing of the pathogen’s growth (St. Clair, Citation2010). The presence of S. sclerotiorum in the vascular tissue of B. napus plants suggests that S. sclerotiorum could theoretically spread to the roots of the plant via the xylem. This infection pathway has been demonstrated for L. maculans in B. napus (Sprague et al., Citation2007), but requires further investigation for the S. sclerotiorum–B. napus pathosystem.
The ability of PAK93, PAK54, DC21 and K22 to maintain durable partial stem resistance in the field remains to be tested, as partial stem resistance to both Canadian and Australian S. sclerotiorum isolates has only been demonstrated in growth chamber and glasshouse environments (this study; Gyawali et al., Citation2016). Screening for SSR partial stem resistance in the field has proven challenging in the past as consistent infection from ascospores can be difficult to achieve across a trial site and across successive seasons (Bradley et al., Citation2006). To date, only a single SSR mapping study has utilized natural infection from ascospores for phenotyping SSR resistance (Wei et al., Citation2014). An alternative option to ascospore inoculation is to directly inoculate field grown plants. However, it is difficult to measure yield losses using this method due to the number of stems that would require inoculation within each field plot. Developing consistent, reproducible field testing strategies for assaying SSR resistance in B. napus remains a technological challenge for both researchers and breeders alike.
Mapping of quantitative trait loci (QTL) conferring resistance to S. sclerotiorum in PAK54, PAK93, DC21 and K22 is in progress. Molecular markers linked to these QTL will be useful in breeding programmes where partial stem resistance in the four accessions is crossed into adapted oilseed rape breeding lines. A combination of marker-assisted-selection and the stem inoculation test is likely the most effective way to develop new varieties with improved resistance to S. sclerotiorum. Identification and cloning of the genes that govern SSR partial stem resistance within the cortex is the next logical step towards a genetic solution for SSR.
Supplemental Material
Download MS Word (3 MB)Acknowledgements
The authors would like to acknowledge Dr Kith Jayasena for collection of the Australian Sclerotinia sclerotiorum isolates used in this study.
Supplementary material
Supplemental data for this article can be accessed online here: https://doi.org/10.1080/07060661.2018.1516699
Additional information
Funding
References
- Australian Grains Genebank. [accessed 2018 Apr 22]. www.seedpartnership.org.au.
- Bastien M, Huynh TT, Giroux G, Iquira E, Rioux S, Belzile F. 2012. A reproducible assay for measuring partial resistance to Sclerotinia sclerotiorum in soybean. Can J Plant Sci. 92:279–288.
- Bradley CA, Henson RA, Porter PM, LeGare DG, Del Rio LE, Khot SD. 2006. Response of canola cultivars to Sclerotinia sclerotiorum in controlled and field environments. Plant Dis. 90:215–219.
- Buchwaldt L, Li R, Hegedus DD, Rimmer SR 2005. Pathogenesis of Sclerotinia sclerotiorum in relation to screening for resistance. In: Proceedings of the 13th International Sclerotinia Workshop; Jun 12–16; Monterey (CA, USA). p. 22.
- Bus A, Korber N, Snowdon RJ, Stich B. 2011. Patterns of molecular variation in a species-wide germplasm set of Brassica napus. Theor Appl Genet. 123:1413–1423.
- Clarkson JP, Warmington RJ, Walley PG, Denton-Giles M, Barbetti MJ, Brodal G, Nordskog B. 2017. Population structure of Sclerotinia subarctica and Sclerotinia sclerotiorum in England, Scotland and Norway. Front Microbiol. doi:10.3389/fmicb.2017.00490
- Davar R, Darvishzadeh R, Majd A. 2011. Genotype-isolate interaction for resistance to Sclerotinia sclerotiorum in sunflower. Phytopathol Mediterr. 50:440–449.
- Davar R, Darvishzadeh R, Majd A, Ghosta Y, Sarrafi A. 2010. QTL mapping of partial resistance to basal stem rot in sunflower using recombinant inbred lines. Phytopathol Mediterr. 49:330–341.
- Del Rio LE, Bradley CA, Henson RA, Endres GJ, Hanson BK, McKay K, Halvorson M, Porter PM, Le Gare DG, Lamey HA. 2007. Impact of Sclerotinia stem rot on yield of canola. Plant Dis. 91:191–194.
- Denton-Giles M, Bradshaw RE, Dijkwel PP. 2013. Ciborinia camelliae (Sclerotiniaceae) induces variable plant resistance responses in selected species of Camellia. Phytopathology. 103:725–732.
- Derbyshire MC, Denton-Giles M. 2016. The control of sclerotinia stem rot on oilseed rape (Brassica napus): current practices and future opportunities. Plant Path. 65:859–877.
- FAOSTAT, 2018. FAOSTAT, production, crops. accessed 2018 Jan. [http://faostat3.fao.org/browse/Q/QC/E].
- Garg H, Atri C, Sandhu PS, Kaur B, Renton B, Banga SK, Singh H, Singh C, Barbetti MJ, Banga S. 2010. High level of resistance to Sclerotinia sclerotiorum in introgression lines derived from hybridization between wild crucifers and the crop Brassica species B. napus and B. juncea. Field Crops Res. 117:51–58.
- Ge XT, Li YP, Wan ZJ, You MP, Finnegan PM, Banga SS, Sandhu PS, Garg H, Salisbury PA, Barbetti MJ. 2012. Delineation of Sclerotinia sclerotiorum pathotypes using differential resistance responses on Brassica napus and B. juncea genotypes enables identification of resistance to prevailing pathotypes. Field Crops Res. 127:248–258.
- Gyawali S, Harrington M, Durkin J, Horner K, Parkin IAP, Hegedus DD, Bekkaoui D, Buchwaldt L. 2016. Microsatellite markers used for genome-wide association mapping of partial resistance to Sclerotinia sclerotiorum in a world collection of Brassica napus. Mol Breed. 36:1–13.
- Huang YJ, Pirie EJ, Evans N, Delourme R, King GJ, Fitt BDL. 2009. Quantitative resistance to symptomless growth of Leptosphaeria maculans (phoma stem canker) in Brassica napus (oilseed rape). Plant Pathol. 58:314–323.
- Huang YJ, Qi A, King GJ, Fitt BDL. 2014. Assessing quantitative resistance against Leptosphaeria maculans (phoma stem canker) in Brassica napus (oilseed rape) in young plants. PLoS One. doi:10.1371/journal.pone.0084924
- Kharbanda PD, Tewari JP. 1996. Integrated management of canola diseases using cultural methods. Can J Plant Pathol. 18:168–175.
- Koch S, Dunker S, Kleinhenz B, Rohrig M, Von Tiedemann A. 2007. Crop loss-related forecasting model for Sclerotinia stem rot in winter oilseed rape. Phytopathology. 97:1186–1894.
- Kohn LM, Carbone I, Anderson JB. 1990. Mycelial interactions in Sclerotinia sclerotiorum. Exp Mycol. 14:255–267.
- Lancashire PD, Bleiholder H, Van Den Boom T, Langelüddeke P, Strauss R, Weber E, Witzenberger A. 1991. A uniform decimal code for growth stages of crops and weeds. Ann Appl Biol. 119:561–601.
- Lujan P, Sanogo S, Puppala N, Randall J. 2016. Factors affecting mycelium pigmentation and virulence of Sclerotinia sclerotiorum on Valencia peanut. Can J Plant Sci. 96:461–473.
- Mei J, Qian L, Disi JO, Yang X, Li Q, Li J, Frauen M, Cai D, Qian W. 2011. Identification of resistant sources against Sclerotinia sclerotiorum in Brassica species with emphasis on B. oleracea. Euphytica. 177:393–399.
- Murray G, Brennan JP 2012. The current and potential costs from diseases of oilseed crops in Australia. [ accessed 2018 Mar]. https://www.grdc.com.au/__data/assets/pdf_file/0021/82641/grdcreportdiseasecostoilseedspdf.pdf.pdf.
- Porter L. 2012. Pea germplasm with partial resistance to Sclerotinia sclerotiorum extends the time required by the pathogen to infect host tissue. Crop Sci. 52:1044–1050.
- Rana K, Atri C, Gupta M, Akhatar J, Sandhu PS, Kumar N, Jaswal R, Barbetti MJ, Banga SS. 2017. Mapping resistance responses to Sclerotinia infestation in introgression lines of Brassica juncea carrying genomic segments from wild Brassicaceae B. fruticulosa. Sci Rep. 7:5904.
- Riddle GE, Burpee LL, Boland GJ. 1991. Virulence of Sclerotinia sclerotiorum and S. minor on dandelion (Taraxacum officinale). Weed Sci. 39:109–118.
- Sexton AC, Howlett BJ. 2004. Microsatellite markers reveal genetic differentiation among populations of Sclerotinia sclerotiorum from Australia canola fields. Curr Genet. 46:357–365.
- Sexton AC, Whitten AR, Howlett BJ. 2006. Population structure of Sclerotinia sclerotiorum in an Australian canola field at flowering and stem-infection stages of the disease cycle. Genome. 49:1408–1415.
- Simko I, Hans-Peter P. 2011. The area under the disease progress stairs: calculation, advantage and application. Phytopathology. 102:381–389.
- Sprague SJ, Watt M, Kirkegaard JA, Howlett BJ. 2007. Pathways of infection of Brassica napus roots by Leptosphaeria maculans. New Phytol. 176:211–222.
- St. Clair DA. 2010. Quantitative disease resistance and quantitative resistance loci in breeding. Annu Rev Phytopathol. 48:247–268.
- Taylor A, Coventry E, Jones JE, Clarkson JP. 2015. Resistance to a highly aggressive isolate of Sclerotinia sclerotiorum in a Brassica napus diversity set. Plant Path. 64:932–940.
- Tok FM, Dervis S, Arslan M. 2016. Analysis of genetic diversity of Sclerotinia sclerotiorum from eggplant by mycelial compatibility, random amplification of polymorphic DNA (RAPD) and simple sequence repeat (SSR) analyses. Biotechnol Biotec Eq. 30:921–928.
- Twengstrom E, Sigvald R, Svensson C, Yuen J. 1998. Forecasting Sclerotinia stem rot in spring sown oilseed rape. Crop Prot. 17:405–411.
- Uloth MB, Clode PL, You MP, Barbetti MJ. 2016. Attack modes and defence reactions in pathosystems involving Sclerotinia sclerotiorum, Brassica carinata, B. juncea and B. napus. Ann Bot. 117:79–95.
- Uloth MB, You MP, Barbetti MJ. 2015. Host resistance to Sclerotinia stem rot in historic and current Brassica and B. juncea varieties: critical management implications. Crop Pasture Sci. 66:841–848.
- Uloth MB, You MP, Finnegan PM, Banga SS, Banga SK, Sandhu PS, Yi H, Salisbury PA, Barbetti MJ. 2013. New sources of resistance to Sclerotinia sclerotiorum for crucifer crops. Field Crops Res. 154:40–52.
- Wang JX, Ma HX, Chen Y, Zhu XF, Yu WY, Tang ZH, Chen CJ, Zhou MG. 2009. Sensitivity of Sclerotinia sclerotiorum from oilseed crops to boscalid in Jiangsu Province of China. Crop Prot. 28:882–886.
- Wei DY, Mei JQ, Fu Y, Disi JO, Li JN, Qian W. 2014. Quantitative trait loci analyses for resistance to Sclerotinia sclerotiorum and flowering time in Brassica napus. Mol Breed. 34:1797–1804.
- Willbur JF, Ding S, Marks ME, Lucas H, Grau CR, Groves CL, Kabbage M, Smith DL. 2017. Comprehensive sclerotinia stem rot screening of soybean germplasm requires multiple isolates of Sclerotinia sclerotiorum. Plant Dis. 101:344–353.
- Wu J, Cai G, Tu J, Li L, Liu S, Luo X, Zhou L, Fan C, Zhou Y. 2013. Identification of QTLs for resistance to sclerotinia stem rot and BnaC.IGMT5.a as a candidate gene of the major resistance QTL SRC6 in Brassica napus. PLoS One. doi:10.1371/journal.pone.0067740