Abstract
Purpose
Curcumin is a polyphenolic compound that is suggested to dysregulate the ubiquitin-proteasome system (UPS). This study investigated the effects of curcumin supplementation on markers of UPS activity in response to muscle damage.
Methods
Twenty-three recreationally active male and females between the ages of 18-30 were randomized into a curcumin (CUR) or placebo (PLA) group. Both groups ingested 2 g of their respective supplement and 20 mg of piperine for 11 consecutive days. Following 8 consecutive days of supplementation, participants performed a 45-minute eccentrically-biased treadmill protocol at 60% VO2max. Muscle biopsies and delayed onset muscle soreness (DOMS) assessments were performed 30 minutes prior and 3, 24, 48, and 72 hours following exercise. Skeletal muscle ubiquitin, MAFbx/Atrogin-1, ubiquitin specific peptidase 19 (USP19), and chymotrypsin-like protease concentrations were measured using ELISA. A 3-way repeated measures ANOVA with pairwise comparisons was conducted with significance set at p ≤ 0.05.
Results
Compared to baseline, DOMS for both groups was significantly increased (p < 0.05) at all time points except 72 hours following exercise. No significant differences were found for USP19 between groups. Ubiquitin (p=.016) and MAFbx/Atrogin-1 (p=.006) were significantly lower for CUR compared to PLA. Additionally, MAFbx/Atrogin-1 was significantly greater for females (p=.013) compared to males. In males, curcumin resulted in significant reductions (p = .049) in chymotrypsin-like protease (p = .049).
Conclusion
While elevations in UPS activity were not observed in response to muscle damage, curcumin supplementation in humans does appear to dysregulate basal UPS activity in the presence of exercise-induced muscle damage.
Introduction
The ubiquitin proteasome system (UPS) is the primary cellular system responsible for controlled degradation of skeletal muscle proteins (Citation1–4). Degradation of these proteins are regulated by two critical and sequential steps: (Citation1) ubiquitination/polyubiquitination of the target protein and (Citation2) degradation of the polyubiquitinated target protein by the 26S proteasome along with recycling of the ubiquitin molecules. Three enzymes primarily govern this highly-regulated process: ubiquitin-activating enzyme (E1), ubiquitin-conjugating enzyme (E2), and ubiquitin-ligase enzyme (E3) (Citation1, Citation5). First, the E1 enzyme activates the COOH-terminal end of ubiquitin and forms a high-energy thioester bond between it and the cysteine residue via an ATP-dependent process. The activated ubiquitin is then transferred to the cysteine residue of the E2 enzyme via the formation of a new thioseter bond. This allows the E3 enzyme to interact with the E2-ubiquitin conjugate and transfer the ubiquitin onto the specific target protein via an isopeptide bond between the ε-amino group of a lysine residue in the target protein and the carboxy-terminal glycine residue 76 in ubiquitin (Citation1, Citation5, Citation6). This process is repeated a minimum of four times until the polyubiquitinated target protein is recognized by the 26S proteasome and is subsequently degraded (Citation7). The 26S proteasome is comprised of a 20S catalytic core and two 19S regulatory caps. The 20S subunit is responsible for the structural support of the proteasome along with the catalytic protease activity (Citation8). The 19S subunit unfolds and disasembles the polyubiquitin chain from the target protein and shuttles it to the 20S core (Citation9, Citation10). This unfolding and removal of ubiquitin from the target protein allows for ubiquitin recycling via the actions of deubiquitinating enzymes (DUB) (Citation11).
Unaccustomed eccentric exercise results in damage of the active skeletal muscle tissue, characterized by elevations in delayed onset muscle soreness (DOMS) (Citation12), inflammation (Citation13), sarcomeric disruption, Z-disk streaming (Citation14, Citation15), serum creatine kinase (Citation16), and troponin I concentrations (Citation17) as well as significant elevations in UPS activity (Citation5, Citation17–19). Being skeletal muscle is the largest protein reservoir in the body, elevations in UPS activity result in the degradation of a number of myofibrillar and sarcomeric proteins. Specifically, E3 ubiquitin-protein ligases, muscle RING-finger protein-1 (MuRF1) and muscle atrophy F-box (MAFbx/Atrogin-1) are responsible for ubiquitination of a number of myofibrillar and sarcomeric proteins including actin, myosin light/heavy chains, myosin binding protein C, troponin I, desmin, vimentin, and the transcriptional factors MyoD and eukaryotic initiation factor 3-f (Citation20–27). Induction of the UPS plays a pivotal role in ridding the cell of damaged/nonfunctional proteins in order to preserve function during periods of skeletal muscle damage. However, while elevations in UPS activity in response to exercise are necessary, chronic and/or hyper-UPS activity may have negative impacts and are associated with many clinical conditions.
Since the UPS plays a large role in degradation of myofibrillar and sarcomeric proteins, disruption of this system may potentially attenuate the large amounts of skeletal muscle atrophy observed during periods of prolonged fasting, inactivity, immobilization, or in clinical conditions such as cancer cachexia, spinal cord injury, sepsis, muscular dystrophy, and sarcopenia (Citation1). Therefore, several pharmacological compounds have been developed in an attempt to dysregulate the ubiquitinating process or catalytic properties of the proteasome (Citation28, Citation29). However, there is also a need to identify naturally-occurring, nutritional compounds with similar properties to provide an alternative to the negative side effects (i.e. diarrhea, vomiting, chest pain, pneumonia, fatigue, infusion reactions, heart failure, hematologic toxicities, etc.) associated with these drugs (Citation30). Most notably, curcumin (diferuloylmethane), a naturally-occurring polyphenolic compound extracted from turmeric root, has been shown to significantly inhibit UPS activity in both in vitro and in vivo animal studies (Citation31–35). Moreover, it has been reported high doses of curcumin supplementation in humans are well tolerated without significant side effects (Citation36).
Research suggests curcumin’s influence on UPS activity is potentiated through its ability to inhibit isopeptidases (the largest class of DUB), protease activity within the 20S catalytic core of the proteasome (trypsin-like, chymotrypsin-like, and peptidyglutamyl-peptide hydrolase), and/or gene/protein expression of ubiquitin (Citation31–35). Therefore, research investigating UPS dysregulation has analyzed ubiquitin specific peptidase activity (i.e. USP11, USP19, USP22, etc.) as a measure of DUB responses, in addition to protease responses within the catalytic core of the proteasome (i.e. trypsin-like, chymotrypsin-like, and peptidyglutamyl-peptide hydrolase activity), and ubiquitin content itself to gain insight into any perturbations in ubiquitinating and proteasomal activity in this system. Additionally, many regulatory enzymes, including specific E3 ubiquitin-protein ligases (MuRF1 and MAFbx/Atrogin-1)], may directly or indirectly be influenced by treatments due to their role in ubiquitination of myofibrillar and sarcomeric proteins. Therefore, the purpose of this study was to determine the impact of curcumin supplementation on skeletal muscle ubiquitin, MAFbx/Atrogin-1, ubiquitin specific peptidase 19 (USP19), and chymotrypsin-like protease activity in the presence of elevations in UPS activity due to exercise-induced muscle damage. We hypothesized elevations in these markers of protein ubiquitination and proteasomal activity in response to the muscle damaging exercise bout would be repressed or attenuated by curcumin supplementation when compared to placebo.
Methods
Experimental approach
In a double-blinded, placebo-controlled, randomized design, participants were instructed to consume curcumin and piperine (CUR) or placebo and piperine (PLA) for 11 consecutive days. Following 8 consecutive days of supplementation, participants performed a 45 minute eccentrically-biased muscle damaging treadmill protocol. Muscle biopsies and delayed onset muscle soreness (DOMS) assessments were performed 30 minutes before and 3, 24, 48, and 72 hours after the exercise-induced muscle damage protocol.
Participants
Twenty-three apparently healthy, recreationally active [regular, consistent, structured exercise at least 3 times per week for at least 1 year prior to the onset of the study], men and women between the ages of 18–30 volunteered to participate in this study. Twenty-four participants were initially recruited for the study, completed informed consent forms, and participated in an initial familiarization session. Of the 24 participants, 23 completed the research study, as one participant withdrew for unrelated circumstances. All 23 participants who completed the study were utilized in data analysis. Enrollment was open to men and women of all ethnicities. Only participants considered as low risk for cardiovascular disease and with no contraindications to exercise as outlined by the American College of Sports Medicine (ACSM), and who had not consumed any nutritional supplements (excluding multi-vitamins) 1 month prior to the study were allowed to participate. All eligible subjects signed university-approved informed consent documents and approval was granted by the Institutional Review Board for Human Subjects of Baylor University. Additionally, all experimental procedures involved in the study conformed to the ethical consideration of the Declaration of Helsinki.
Familiarization, body composition, and maximal aerobic capacity (VO2max) assessment
Total body mass (kg) was determined on a standard dual beam balance scale (Detecto Bridgeview, IL, USA). Body composition analyses were determined using DEXA (Hologic Discovery Series W, Waltham, MA, USA). Quality control calibration procedures were performed on a spine phantom prior to each testing session. Total body water was determined by bioelectric impedance spectroscopy (ImpediMed Ltd., Australia). Based on previous studies in our laboratory, the accuracy of the DEXA for body composition assessment is ±3.7% as assessed by direct comparison with hydrodensitometry and scale weight (Citation37–39). In order to determine maximal aerobic capacity (VO2max), participants performed a volitional maximal cardiopulmonary exercise (VO2max) test on a laboratory treadmill using the Bruce protocol (Citation40). Participants were instructed to perform the test for as long as possible to ensure a true maximal attempt. Standard ACSM test termination criteria were monitored and followed throughout each test. Metabolic gases were obtained with the Parvo Medics 2400 TrueMax metabolic measurement system (Parvo Medics, Sandy, Utah) to determine maximal aerobic capacity. The mean coefficient of variation for this protocol has previously been shown to be 6.5% (range 2%–14%) (Citation40). Participant dietary intakes were not standardized; however, participants were instructed not to change their diet throughout the duration of the study.
Eccentric exercise bout
Exactly seven days following supplementation, participants performed an eccentrically-biased aerobic exercise bout that induced muscle damage. Previously published research from our laboratory has shown this protocol to increase markers of skeletal muscle injury (Citation41). On a downhill treadmill positioned at a grade of 10° (−17.6%), participants ran for 45 minutes at 60% of their previously-determined VO2max. Oxygen consumption, heart rate, and ratings of perceived exertion [RPE (using the Borg’s scale)] were monitored every five minutes, and the treadmill speed was adjusted accordingly to maintain intensity at 60% of VO2max.
Delayed onset muscle soreness assessment
As a corollary determinant toward the severity of muscle injury, the delayed onset of muscle soreness (DOMS) was assessed using a visual analog scale (VAS) as previously published (Citation12, Citation40). Muscle soreness quantified via a VAS has been shown to be positively correlated with peak creatine kinase activity, a well-established surrogate marker of skeletal muscle damage (Citation42). Participants rated their level of soreness immediately prior and 3, 24, 48, and 72 hours following the muscle damaging exercise bout by drawing an intersecting line across a 10-cm scale (0 = no soreness, 10 = extreme soreness). The distance of each mark was measured from 0, and the measurement was used as the perceived soreness level. Participants were instructed to give the perceived soreness from the muscles of the leg that was not biopsied to assess soreness related to the exercise alone.
Supplementation protocol
In a double-blind randomized manner, participants were assigned to orally ingest 2 g of cellulose placebo (Nutricology, Alameda, CA, USA) or 2 g of curcumin [(1E,6E)-1,7-bis (4-hydroxy- 3-methoxyphenyl)-1,6- heptadiene-3,5-dione)] standardized to 95% curcuminoids [certificate of analysis verified 99% purity and 95% curcuminoids (Bulk Supplements, Henderson, NV, USA)]. Additionally, previous pharmacokinetic data has demonstrated in healthy male participants piperine increases curcumin bioavailability by 2000% and significantly elevates curcumin serum concentrations with a Tmax of 0.69 h ± 0.07 (Citation43). Therefore, both groups also supplemented with 20 mg of piperine [1-(5-[1,3-benzodioxol5-yl]-1-oxo-2,4-pentadienyl)] standardized to 95% 1-piperoylpiperidine (Natures Plus, Amityville, NY, USA). Participants ingested their respective supplement daily beginning 7 days prior to the muscle damaging exercise bout to ensure proper efficacy and saturation of the supplement continuing throughout the entire 11-day study. Compliance of the supplementation protocol was determined by a daily supplement compliance questionnaire and mean ± SD percent compliance for CUR and PLA was 99.10 ± 2.98% and 100 ± 0.00%, respectively.
Muscle biopsies
Percutaneous muscle biopsies (30–40 mg) were obtained from the middle portion of the vastus lateralis muscle using a fine needle aspiration (FNA) technique on the participant’s self-reported dominant leg. Biopsies were performed at the midpoint between the patella and the greater trochanter of the femur at a depth between 1 and 2 cm. A subcutaneous injection of 1% Lidocaine without epinephrine was used prior to the procedure in order anesthetize the area. After the initial biopsy, the remaining biopsies were made to extract tissue from approximately the same location as the initial biopsy by using the pre-biopsy scar, depth markings on the needle, and a successive incision that was made approximately 0.5 cm to the former from medial to lateral. After removal, adipose tissue was trimmed from the muscle specimens and immediately frozen and stored at 80 °C for later analysis. Muscle samples were obtained 30 min pre-exercise and 3, 24, 48, and 72 hours post-exercise.
Skeletal muscle cellular extraction and muscle protein quantification
Each muscle sample was weighed and subsequently homogenized using a commercial cell extraction buffer (Biosource, Camarillo, CA) and a tissue homogenizer. The cell extraction buffer was supplemented with 1 mM phenylmethanesulphonylfluoride (PMSF) and a protease inhibitor cocktail (Sigma Chemical Company, St. Louis, MO) with broad specificity for the inhibition of serine, cysteine, and metallo-proteases. Total protein was quantified from the skeletal muscle cellular extracts spectrophotometrically based on the DC colorimetric protein assay method at a wavelength of 750 nm (Bio-Rad Hercules, CA, USA) using bovine serum albumin as the protein standard.
Assessment of skeletal muscle markers of ubiquitination and proteasome activity
The concentrations of skeletal muscle ubiquitin, muscle atrophy F-box/Atrogin-1 (MAFbx/Atrogin-1), chymotrypsin-like protease, and ubiquitin specific peptidase 19 (USP19) content were measured using commercially-available ELISA kits (MyBioSource, San Diego, California, USA) based on manufacturer’s guidelines. The absorbances, which were directly proportional to the concentration of analyte in the sample, were measured at a wavelength of 450 nm using a microplate reader (iMark, Bio-Rad, Hercules, CA). A set of standards of known concentrations for each protein were utilized to construct a standard curve by plotting the net absorbance values of the standards against the respective peptide concentrations. Using data reduction software (Microplate Manager, Bio-Rad, Hercules, CA), protein concentrations were determined and expressed relative to muscle total protein concentration.
Statistical analyses
Statistical analyses were performed by utilizing separate 3-way [Condition (curcumin, placebo) x Gender (male, female) x Time (30 minutes pre-, 3-, 24-, 48-, and 72-hours post-exercise)] factorial analyses of variance (ANOVA) with repeated measures for DOMS, muscle ubiquitin, muscle MAFbx/Atrogin-1, muscle chymotrypsin-like protease activity and muscle USP19 content. If a significant interaction was found, simple effects analysis was conducted to determine where the interaction occurred. If a significant interaction was present, analysis of main effects was conducted using the simple effects, pairwise comparisons with a Bonferroni adjustment to compare dependent variables within each independent variable condition. If no interaction was present, then normal pairwise comparisons with a Bonferroni adjustment were used to test main effects. The magnitude of statistical significance was measured by effect size (Partial Eta-Squared), which estimates the ratio of variance in the dependent variable that is explained by the independent variable. Partial Eta Squared effect sizes (η2) are characterized 0.1–0.3 as small, 0.3–0.5 as medium, and ≥0.5 as large (Citation44). All statistical procedures were performed using SPSS 25.0 software and an alpha level of ≤0.05 was set for all statistical measures.
Results
Participant demographics and exercise bout responses
Sample size, along with the baseline means for age, height, weight, total body water, and body composition for the 23 participants are listed in . No significant differences were found between groups. Relative exercise intensity (%maxVO2/%maxHR) of the muscle damaging exercise bout for CUR and PLA (55.94 ± 5.74/87.63 ± 4.14 vs. 57.72 ± 4.50/85.35 ± 3.70) and average participant rating of perceived exertion of the muscle damaging exercise bout for CUR and PLA (14.75 ± 2.16 vs 14.73 ± 1.57) were not significantly different ( and ).
Figure 1. Mean (± SD) percent (%) maximum of oxygen consumption (VO2) and heart rate (HR) between CUR (55.94 ± 5.74/87.63 ± 4.14) and PLA (57.72 ± 4.50/85.35 ± 3.70) in response to a eccentrically-biased muscle damaging protocol. No significant differences between CUR and PLA were found for %VO2 and HP (p > 0.05).
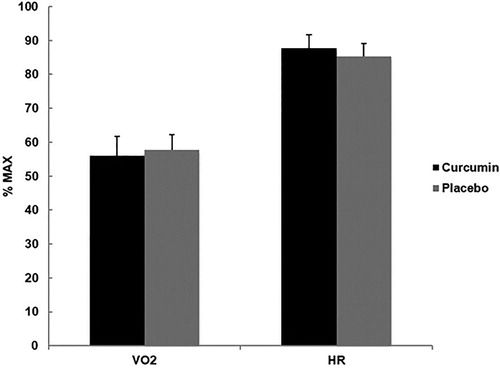
Figure 2. Mean (± SD) rating of perceived exertion (RPE) for CUR (14.75 ± 2.16) and PLA (14.73 ± 1.57) using the Borg’s scale during an eccentrically-biased muscle damaging protocol. No significant differences between groups were found (p > 0.05).
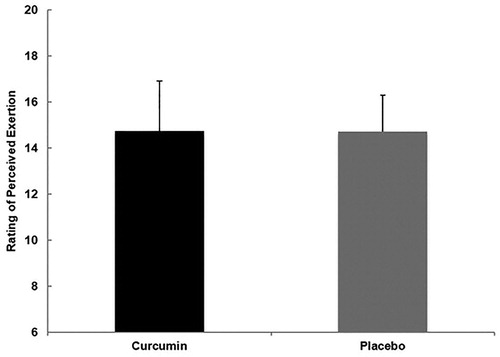
Table 1. Participant demographics.
Delayed onset muscle soreness
No significant interactions for time x group (p = .153, η2 = .088, group x gender (p = .764, η2 = .005), time x gender (p = .249, η2 = .071), or group x gender x time (p = .521, η2 = .043) were observed for DOMS. Additionally, no significant main effect for group (p = .504, η2 = .025) or gender (p = .699, η2 = .009) was found. However, there was a significant main effect for time (p < .001, η2 = .616). Pairwise comparisons indicated that across both conditions DOMS was significantly greater from baseline at all time points except 72 hours post exercise induced muscle damage (p = .082) ().
Figure 3. Perceived soreness for group (A) and gender x group (B) in response to an eccentrically-biased muscle damaging protocol. A significant time effect (p <.001) was found for both groups. Pairwise comparisons indicated that perceived soreness was significantly greater from baseline at all time points except 72 hours post exercise induced muscle damage (p =.082). No significant differences were found between genders or gender by group. Means (± SD) are shown above the bars. ∗Indicates a significant time effect (p < 0.05).
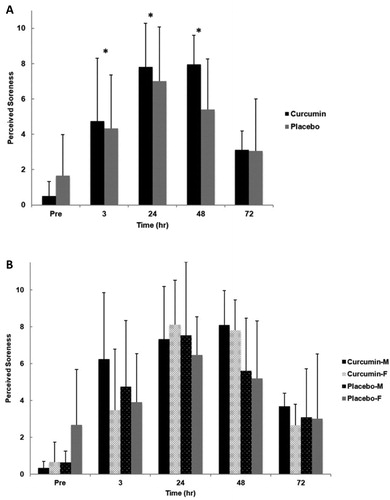
Skeletal muscle ubiquitin
No significant interactions for time x group (p = .422, η2 = .049), group x gender (p = .874, η2 = .001), time x gender (p = .881, η2 = .015), or group x gender x time (p = .554, η2 = .038) were observed for skeletal muscle ubiquitin content. Additionally, no significant main effect for gender (p = .635, η2 = .012) or time (p = .853, η2 = .017) was found. However, a significant main effect for group (p = 0.016, η2= .271) was observed where CUR was significantly lower (∼30%) than PLA regardless of time ().
Figure 4. Skeletal muscle ubiquitin for group (A) and gender x group (B) in response to an eccentrically-biased muscle damaging protocol. A significant group effect (p =.012) where CUR was significantly lower than PLA regardless of time. Means (± SD) are shown above the bars. ∗Indicates a significant group effect across all time points (p < 0.05).
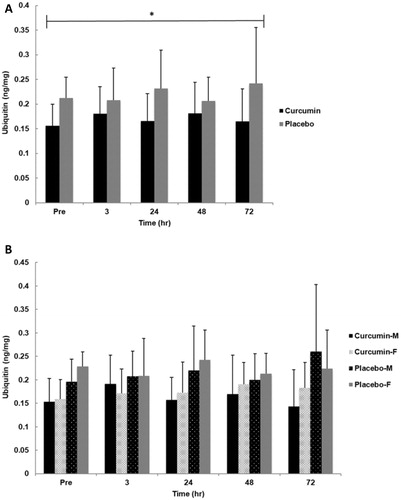
Skeletal muscle MAFbx/atrogin-1
No significant interactions for time x group (p = .370, η2 = .053), group x gender (p = .245, η2 = .071) time x gender (p = .682, η2 = .024), or group x gender x time (p = .337, η2 = .057) were observed for skeletal muscle MAFbx/Atrogin-1 content. Additionally, no significant main effect for time (p = .530, η2 = .037) was observed. However, a significant main effect for group (p = 0.006, η2 = .338) was observed where CUR was significantly lower (∼51%) than PLA regardless of time. There was also a significant main effect for gender (p = .013, η2 = .282) indicating females had significant greater values than males ().
Figure 5. Skeletal muscle MAFbx/Atrogin-1 for group (A) and gender x group (B) in response to an eccentrically-biased muscle damaging protocol. A significant group effect (p =.016) where CUR was significantly lower than PLA regardless of time. Also, a significant main effect for gender (p =.013) was observed where females had significant greater values than males. Means (± SD) are shown above the bars. ∗Indicates a significant group effect across all time points (p < 0.05).
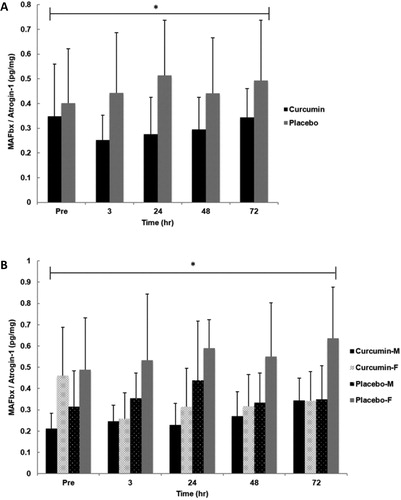
Skeletal muscle chymotrypsin-like protease
No significant main effect for group (p = .150, η2 = .106), gender (p = .817, η2 = .003) or time (p = .355, η2 = .056) was observed for skeletal muscle chymotrypsin-like protease content. Additionally, no significant interactions for time x group (p = .078, η2 = .103), time x gender (p = .407, η2 = .051), or group x gender x time (p = .932, η2 = .011) for were found. However, a significant group x gender interaction (p = .049, η2 = .188) was observed where males had lower values with curcumin supplementation while females had slightly higher ().
Figure 6. Skeletal muscle chymotrypsin-like protease activity for group (A) and gender x group (B) in response to an eccentrically-biased muscle damaging protocol. No significant differences were found between groups. However, a significant main effect for group x gender (p =.049) was observed where males had markedly lower values with curcumin supplementation while females had slightly higher. Means (± SD) are shown above the bars. ∗Indicates a significant group effect across all time points (p < 0.05).
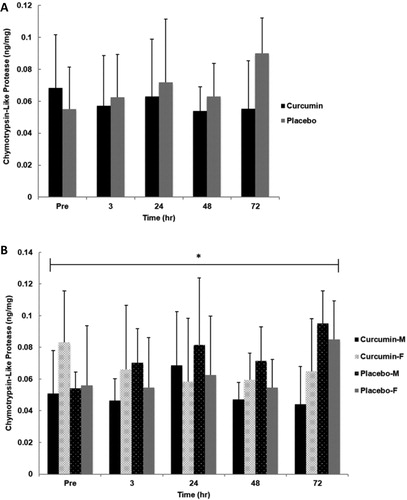
Skeletal muscle ubiquitin specific peptidase 19
No significant main effect for group (p = .108, η2 = .130), gender (p = .373, η2 = .042) or time (p = .153, η2 = .094) was observed for skeletal muscle ubiquitin specific peptidase 19 content. Additionally, no significant interactions for time x group (p = .212, η2 = .079), time x gender (p = .704, η2 = .018), group x gender (p = .492, η2 = .025), or time x group x gender (p = .776, η2 = .013) were found ().
Discussion
This is the first study to date investigating the impacts of curcumin on protein ubiquitination and proteasome activity in humans or in an exercise model. Our results demonstrate curcumin supplementation effectively suppressed markers of protein ubiquitination in human skeletal muscle. We observed that respective skeletal muscle ubiquitin and MAFbx/Atrogin-1 protein concentrations were significantly lower (∼30% & ∼51%) in CUR compared to PLA, respectively, regardless of time. Additionally, we observed significant DOMS increases in both groups, corroborating the eccentrically-biased exercise bout. This suggests curcumin-mediated decreases in ubiquitinating activity occurred in the presence of exercise-induced muscle damage. Regardless, exercise-induced elevations in UPS activity cannot be confirmed due to the lack of increases in our markers of protein ubiquitination or proteasome activity over time. We speculate this lack of UPS response may be due to variability in subject training experience or muscle damage incurred by the exercise protocol (Citation45). Contrary to previous research (Citation46), our study demonstrates curcumin’s ability to effectively dysregulate UPS activity through suppression of basal protein ubiquitination without any attenuating impacts on perceived soreness. Interestingly, we found no statistical differences in chymotrotrypsin-like protease or USP19 content between groups indicating curcumin did not suppress DUB activity or catalytic activity of the proteasome itself. This provides further evidence of curcumin-mediated UPS dysregulation occurring during the ubiquitinating process rather than at the proteasomal level. Nevertheless, while chymotrypsin-like protease and USP19 are our surrogate markers of DUB and proteasome activity, they may not represent the collective activity of all DUBs and proteolytic activities of the proteasome. Moreover, we also found a group x gender interaction where males had lower values of chymotrypsin-like protease content with curcumin supplementation compared to females. Additionally, females collectively had greater values of MAFbx/Atrogin-1. Due to the novelty in these markers and lack of existing data in humans, gender differences cannot be explained by these data alone. Collectively, these data demonstrate curcumin supplementation is able to disrupt the UPS in humans and in the presence of exercise-induced muscle damage.
There have been multiple proposed mechanisms to explain the observed dysregulation in UPS activity. Curcumin has been reported to induce proteasomal malfunction through directly binding to the 20S subunit (likely due to curcumin’s carbonyl carbons susceptible to nucleophilic attack by Thr 1 within the β5 subunit of the proteasome) inhibiting chymotrypsin-like, trypsin-like, and peptidylglutamyl-peptide hydrolase protease activity (Citation33), or indirectly by inhibiting DUB activity (Citation34), inducing oxidative stress (Citation47, Citation48), increasing misfolded and/or oxidized proteins, or suppressing ubiquitin gene/protein expression (Citation32). While the mechanism cannot be elucidated from these findings, our data provides evidence that curcumin suppresses ubiquitin protein expression and decreases ubiquitinating activity of the E3 ubiquitin protein ligase MAFbx/Atrogin-1. Theoretically, decreases in ubiquitination processes may result in marked suppressions in protein degradation and attenuate muscle protein breakdown. However, extreme UPS suppression may also lead to toxic cellular conditions due to the cell’s efforts to degrade and rid themselves of these damaged polyubiquitinated proteins. This may negatively influence enzymatic activity in the cell, hinder transcriptional/translational capabilities, and lead to significant decreases in proteasome activity resulting in suppressions in the chymotrypsin-like, trypsin-like, and peptidylglutamyl-peptide hydrolase protease activity of the 20S subunit. However, our marker of DUB activity (USP19) and proteasome activity (chymotrypsin-like protease) did not change in response to muscle damage with curcumin supplementation. Therefore, curcumin does not appear to influence the proteasome itself but rather the preluding ubiquitinating process.
Our current results illustrate curcumin-mediated USP dysregulation by decreasing catalytic enzymes within the proteasome. Previous in vitro (Citation31–35) and in vivo (Citation33) data reinforces our findings which specifically highlights inhibition of DUB activity and dysregulation of free ubiquitin and ubiquitin-protein conjugates (Citation31–35). While we also demonstrated no collective significant difference in chymotrypsin-like protease for both genders, we did observe in males that curcumin resulted in significantly less activity than females. However, in mouse neuro 2a cells treated with curcumin Jana et al. (Citation32) demonstrated a dramatic inhibition of chymotrypsin-like, trypsin-like, and peptidyl glutamyl-peptide hydrolase protease activity and observed an accumulation of ubiquinated proteins accompanied with decreases in free ubiquitin in a dose-dependent manner. Moreover, our study corroborates the decreases in ubiquitin and opposes the decreases in DUB and catalytic proteasomal activity found by Mullally & Fitzpatrick (Citation34) and Jana et al. (Citation32), while also providing evidence that curcumin may further disrupt ubiquitinating activity through suppression of the E3 ubiquitin protein ligase MAFbx/Atrogin-1. Future research needs to investigate this possible source of UPS dysregulation and whether other E3 ligases are similarly influenced in humans.
The major finding of this study is that curcumin supplementation in humans does appear to dysregulate the UPS at rest and in the presence of exercise-induced muscle damage. This curcumin-induced protein ubiquitination malfunction provides preliminary evidence of curcumin supplementation’s potential therapeutic role in decreasing the myofibrillar and sarcomeric protein losses associated with atrophic scenarios. This is relevant during periods of prolonged fasting, inactivity, immobilization, or in other clinical conditions. Therefore, a limitation of the present study is that healthy, recreationally active individuals may not translate to these scenarios in which the UPS is up-regulated. Moreover, our limited sample size and variability in muscle damage between subjects may be responsible for the lack of exercise-induced elevations in UPS activity and curcumin’s subsequent impact on these markers. Thus, more research is needed to provide further insight into curcumin’s influence on this system and the mechanisms that regulate its activity. Lastly, another limitation of this study is the use of an eccentrically-biased muscle damaging protocol. Therefore, using an alternative exercise model such as with an isokinetic dynamometer or resistance exercise may yield a different outcome.
This is the first study to investigate curcumin’s influence on UPS activity in humans and also employing an exercise model. Our study was designed to determine the impact of curcumin supplementation on skeletal muscle ubiquitin, MAFbx/Atrogin-1, USP19, and chymotrypsin-like protease activity at rest and in response to exercise-induced muscle damage. Interestingly, we found significantly lower values in ubiquitin and MAFbx indicating marked suppression in basal UPS activity occurring at rest and in the presence of exercise-induced muscle damage. As a result, we conclude that while UPS activity was not elevated in response to the muscle damaging exercise bout, curcumin supplementation in humans does appear to dysregulate basal UPS activity in the presence of exercise-induced muscle damage.
Disclosure statement
No potential conflict of interest was reported by the author(s).
Additional information
Funding
References
- Bilodeau PA, Coyne ES, Wing SS. The ubiquitin proteasome system in atrophying skeletal muscle: Roles and regulation. Am J Physiol Cell Physiol. 2016;311(3):C392–C403. doi:10.1152/ajpcell.00125.2016.
- Jagoe RT, Goldberg AL. What do we really know about the ubiquitin-proteasome pathway in muscle atrophy? Current Opinion in. Clin Nutr Metab Care. 2001;4(3):183–90.
- Schiaffino S, Dyar KA, Ciciliot S, Blaauw B, Sandri M. Mechanisms regulating skeletal muscle growth and atrophy. Febs J. 2013;280(17):4294–314. doi:10.1111/febs.12253.
- Wing S, Lecker S, Jagoe R. Proteolysis in illness-associated skeletal muscle atrophy: from pathways to networks. Crit Rev Clin Lab Sci. 2011;48(2):49–70. doi:10.3109/10408363.2011.586171.
- Murton AJ, Constantin D, Greenhaff PL. The involvement of the ubiquitin proteasome system in human skeletal muscle remodelling and atrophy. Biochim Biophys Acta. 2008;1782(12):730–43. doi:10.1016/j.bbadis.2008.10.011.
- Passmore LA, Barford D. Getting into position: the catalytic mechanisms of protein ubiquitylation. Biochem J. 2004;379(Pt 3):513–25. doi:10.1042/BJ20040198.
- Thrower JS, Hoffman L, Rechsteiner M, Pickart CM. Recognition of the polyubiquitin proteolytic signal. Embo J. 2000;19(1):94–102. doi:10.1093/emboj/19.1.94.
- Tanaka K. The proteasome: overview of structure and functions. Proc Jpn Acad, Ser B, Phys Biol Sci. 2009;85(1):12–36. doi:10.2183/pjab.85.12.
- Kish-Trier E, Hill CP. Structural biology of the proteasome. Annu Rev Biophys. 2013;42:29–49. doi:10.1146/annurev-biophys-083012-130417.
- Liu J, Shaik S, Dai X, Wu Q, Zhou X, Wang Z, Wei W. Targeting the ubiquitin pathway for cancer treatment. Biochim Biophys Acta. 2015;1855(1):50–60. doi:10.1016/j.bbcan.2014.11.005.
- Reyes-Turcu FE, Ventii KH, Wilkinson KD. Regulation and cellular roles of ubiquitin-specific deubiquitinating enzymes. Annu Rev Biochem. 2009;78:363–97. doi:10.1146/annurev.biochem.78.082307.091526.
- Buford TW, Cooke MB, Redd LZ, Hudson GM, Shelmadine BD, Willoughby DS. Protease supplementation improves muscle function after eccentric exercise. Med Sci Sports Exercise. 2009;41(10):1908–14.
- Fielding RA, Manfredi TJ, Ding W, Fiatarone MA, Evans WJ, Cannon JG. Acute phase response in exercise. III. neutrophil and IL-1 beta accumulation in skeletal muscle. Am J Physiol. 1993;265(1):R166–R172. doi:10.1152/ajpregu.1993.265.1.R166.
- Fridén J, Sjöström J, Ekblom B. A morphological study of delayed muscle soreness. Experientia. 1981;37(5):506–7. doi:10.1007/BF01986165.
- Fridén J, Sjöström J, Ekblom B. Myofibrillar damage following intense eccentric exercise in man. Int J Sports Med. 1983;4(3):170–6. doi:10.1055/s-2008-1026030.
- Nosaka K, Clarkson P. Relationship between post-exercise plasma CK elevation and muscle mass involved in the exercise. Int J Sports Med. 1992;13(06):471–5. doi:10.1055/s-2007-1021300.
- Willoughby DS, Taylor M, Taylor L. Glucocorticoid receptor and ubiquitin expression after repeated eccentric exercise. Med Sci Sports Exercise. 2003;35(12):2023–31.
- Thompson HS, Scordilis SP. Ubiquitin changes in human biceps muscle following exercise-induced damage. Biochem Biophys Res Commun. 1994;204(3):1193–8. doi:10.1006/bbrc.1994.2589.
- Willoughby DS, Rosene J, Myers J. HSP-72 and ubiquitin expression and caspase-3 activity after a single bout of eccentric exercise. J Exercise Physiol Online. 2003;6(2):96–104.
- Clarke BA, Drujan D, Willis MS, Murphy LO, Corpina RA, Burova E, Rakhilin SV, Stitt TN, Patterson C, Latres E, et al. The E3 ligase MuRF1 degrades myosin heavy chain protein in dexamethasone-treated skeletal muscle. Cell Metab. 2007;6(5):376–85. doi:10.1016/j.cmet.2007.09.009.
- Cohen S, Brault JJ, Gygi SP, Glass DJ, Valenzuela DM, Gartner C, Latres E, Goldberg AL. During muscle atrophy, thick, but not thin, filament components are degraded by MuRF1-dependent ubiquitylation. J Cell Biol. 2009;185(6):1083–95. doi:10.1083/jcb.200901052.
- Csibi A, Cornille K, Leibovitch MP, Poupon A, Tintignac LA, Sanchez AMJ, Leibovitch SA. The translation regulatory subunit eIF3f controls the kinase-dependent mTOR signaling required for muscle differentiation and hypertrophy in mouse. PloS One. 2010;5(2):e8994doi:10.1371/journal.pone.0008994.
- Fielitz J, Kim M-S, Shelton JM, Latif S, Spencer JA, Glass DJ, Richardson JA, Bassel-Duby R, Olson EN. Myosin accumulation and striated muscle myopathy result from the loss of muscle RING finger 1 and 3. J Clin Invest. 2007;117(9):2486–95. doi:10.1172/JCI32827.
- Kedar V, McDonough H, Arya R, Li HH, Rockman HA, Patterson C. Muscle-specific RING finger 1 is a bona fide ubiquitin ligase that degrades cardiac troponin I. Proc Natl Acad Sci USA. 2004;101(52):18135–40. doi:10.1073/pnas.0404341102.
- Polge C, Heng A-E, Jarzaguet M, Ventadour S, Claustre A, Combaret L, Béchet D, Matondo M, Uttenweiler-Joseph S, Monsarrat B, et al. Muscle actin is polyubiquitinylated in vitro and in vivo and targeted for breakdown by the E3 ligase MuRF1. Faseb J. 2011;25(11):3790–802. doi:10.1096/fj.11-180968.
- Sandri M. Protein breakdown in muscle wasting: role of autophagy-lysosome and ubiquitin-proteasome. Int J Biochem Cell Biol. 2013;45(10):2121–9. doi:10.1016/j.biocel.2013.04.023.
- Tintignac LA, Lagirand J, Batonnet S, Sirri V, Leibovitch MP, Leibovitch SA. Degradation of MyoD mediated by the SCF (MAFbx) ubiquitin ligase. J Biol Chem. 2005;280(4):2847–56. [Database] doi:10.1074/jbc.M411346200.
- Jamart C, Raymackers JM, Li An G, Deldicque L, Francaux M. Prevention of muscle disuse atrophy by MG132 proteasome inhibitor. Muscle Nerve. 2011;43(5):708–15. doi:10.1002/mus.21949.
- Yin L, Krantz B, Russell NS, Deshpande S, Wilkinson KD. Nonhydrolyzable diubiquitin analogues are inhibitors of ubiquitin conjugation and deconjugation. Biochemistry. 2000;39(32):10001–10. doi:10.1021/bi0007019.
- Merin NM, Kelly KR. Clinical use of proteasome inhibitors in the treatment of multiple myeloma. Pharmaceuticals (Basel). 2014;8(1):1–‐20. doi:10.3390/ph8010001.
- Dikshit P, Goswami A, Mishra A, Chatterjee M, Jana NR. Curcumin induces stress response, neurite outgrowth and prevent NF-kappaB activation by inhibiting the proteasome function . Neurotox Res. 2006;9(1):29–37. doi:10.1007/BF03033305.
- Jana NR, Dikshit P, Goswami A, Nukina N. Inhibition of proteasomal function by curcumin induces apoptosis through mitochondrial pathway. J Biol Chem. 2004;279(12):11680–5. doi:10.1074/jbc.M310369200.
- Milacic V, Banerjee S, Landis-Piwowar KR, Sarkar FH, Majumdar APN, Dou QP. Curcumin inhibits the proteasome activity in human colon cancer cells in vitro and in vivo. Cancer Res. 2008;68(18):7283–92. doi:10.1158/0008-5472.CAN-07-6246.
- Mullally JE, Fitzpatrick FA. Pharmacophore model for novel inhibitors of ubiquitin isopeptidases that induce p53-independent cell death. Mol Pharmacol. 2002;62(2):351–8. doi:10.1124/mol.62.2.351.
- Si X, Wang Y, Wong J, Zhang J, McManus BM, Luo H. Dysregulation of the ubiquitin-proteasome system by curcumin suppresses coxsackievirus B3 replication. J Virol. 2007;81(7):3142–50. doi:10.1128/JVI.02028-06.
- Fernández-Lázaro D, Mielgo-Ayuso J, Seco Calvo J, Córdova Martínez A, Caballero García A, Fernandez-Lazaro CI. Modulation of exercise-induced muscle damage, inflammation, and oxidative markers by curcumin supplementation in a physically active population: a systematic review. Nutrients. 2020;12(2):501. doi:10.3390/nu12020501.
- Shelmadine B, Cooke M, Buford T, Hudson G, Redd L, Leutholtz B, Willoughby DS. Effects of 28 days of resistance exercise and consuming a commercially available pre-workout supplement, NO-Shotgun, on body composition, muscle strength and mass, markers of satellite cell activation, and clinical safety markers in males. J Int Soc Sports Nutr. 2009;6(1):16. doi:10.1186/1550-2783-6-16.
- Spillane M, Schwarz N, Leddy S, Correa T, Minter M, Longoria V, Willoughby DS. Effects of 28 days of resistance exercise while consuming commercially available pre- and post-workout supplements, NO-Shotgun and NO-Synthesize® on body composition, muscle strength and mass, markers of protein synthesis, and clinical safety markers in males. Nutr Metab (Lond). 2011;8(1):78. doi:10.1186/1743-7075-8-78.
- Willoughby DS, Leutholtz BD. Aspartic acid supplementation combined with 28 days of heavy resistance training has no effect on body composition, muscle strength, and serum hormones associated with the hypothalamo-pituitary-gonadal axis in resistance-trained men. Nutr Res. 2013;33(10):803–10. doi:10.1016/j.nutres.2013.07.010.
- Fielding RA, Frontera WR, Hughes VA, Fisher EC, Evans WJ. The reproducibility of the Bruce protocol exercise test for the determination of aerobic capacity in older women. Med Sci Sports Exercise. 1997;29(8):1109–13.
- Buford TW, Cooke MB, Shelmadine BD, Hudson GM, Redd L, Willoughby DS. Effects of eccentric treadmill exercise on inflammatory gene expression in human skeletal muscle. Appl Physiol Nutr Metab. 2009;34(4):745–53. doi:10.1139/H09-067.
- Kim J, Lee J. The relationship of creatine kinase variability with body composition and muscle damage markers following eccentric muscle contractions. Journal of Exercise Nutrition & Biochemistry. 2015;19(2):123–9. doi:10.5717/jenb.2015.15061910.
- Shoba G, Joy D, Joseph T, Majeed M, Rajendran R, Srinivas PS. Influence of piperine on the pharmacokinetics of curcumin in animals and human volunteers. Planta Med. 1998;64(04):353–6. doi:10.1055/s-2006-957450.
- Bakeman R. Recommended effect size statistics for repeated measures designs. Behav Res Methods. 2005;37(3):379–84. doi:10.3758/bf03192707.
- Fernandes JFT, Lamb KL, Twist C. Exercise-induced muscle damage and recovery in young and middle-aged males with different resistance training experience. Sports (Basel). 2019;7(6):132. doi:10.3390/sports7060132.
- Nicol LM, Rowlands DS, Fazakerly R, Kellett J. Curcumin supplementation likely attenuates delayed onset muscle soreness (DOMS). Eur J Appl Physiol. 2015;115(8):1769–77. doi:10.1007/s00421-015-3152-6.
- Bhaumik S, Anjum R, Rangaraj N, Pardhasaradhi BV, Khar A. Curcumin mediated apoptosis in AK-5 tumor cells involves the production of reactive oxygen intermediates. FEBS Lett. 1999;456(2):311–4. doi:10.1016/s0014-5793(99)00969-2.
- Woo J-H, Kim Y-H, Choi Y-J, Kim D-G, Lee K-S, Bae JH, Min DS, Chang J-S, Jeong Y-J, Lee YH, et al. Molecular mechanisms of curcumin-induced cytotoxicity: induction of apoptosis through generation of reactive oxygen species, down-regulation of Bcl-XL and IAP, the release of cytochrome c and inhibition of Akt. Carcinogenesis. 2003;24(7):1199–208. doi:10.1093/carcin/bgg082.