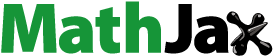
Abstract
In this study, we present the development of an innovative dry powder dexamethasone (Dex) nasal delivery system comprising Dex-loaded lipid/alginate nanoparticles incorporated within pectin microspheres (Dex/NPs-loaded pectin microspheres; DNM). DNM microspheres were characterized by the mean diameter of 2.76 ± 0.10 µm, zeta-potential of –36.2 ± 1.1 mV, and drug loading of 3.3 ± 0.3%. The morphology study revealed irregular microsphere surface forming external voids. In contact with simulated nasal fluid, DNM microspheres demonstrated desirable property of moderate swelling and ensured stronger mucoadhesion compared with conventional Dex-loaded pectin microspheres. The strategy of Dex incorporation within the lipid/alginate NPs resulted in prolonged Dex release in relation to Dex being directly entrapped within the conventional pectin microspheres. DNM microspheres showed excellent biocompatibility and rendered Dex permeation across the selected epithelial cell model similar to that of Dex solution. In conclusion, balanced biopharmaceutical properties of the proposed nasal Dex delivery system provides the potential for prolonged contact time with nasal mucosa, prolonged therapeutic effect, and improved patient compliance.
1. Introduction
Chronic rhinosinusitis is an inflammation of the nose and paranasal sinuses that lasts longer than 12 weeks. It can be divided in two main subtypes: chronic rhinosinusitis with and without nasal polyps. Chronic rhinosinusitis with nasal polyps affects about 20% of patients with chronic rhinosinusitis. It is characterized with symptoms of prominent nasal obstruction and reduction or complete loss of smell. However, this subtype tends to be more refractory to conventional medical treatment, requires more surgical intervention, and has greater morbidity.[Citation1] The first step in treatment of chronic rhinosinusitis with nasal polyps includes pharmacotherapy with corticosteroids. Endoscopic sinus surgery is recommended only when medical treatment fails.[Citation2] Even though nasal polyps can successfully be removed, post-surgical recurrence is common because the underlying inflammatory process fails to be addressed.[Citation3] Corticosteroids have shown to be the most effective drugs for chronic rhinosinusitis with nasal polyps treatment. Since orally applied corticosteroids have well-known side effects, topically applied corticosteroids are the natural choice.[Citation3] To be effective, topically applied corticosteroids have to reach the site of polyp growth, which is beyond the nasal valve. Current interests are focused on the development of nasal delivery device that could assure deposition of formulation at the site of polyp growth.[Citation4,Citation5] Besides the delivery device, characteristics of the formulation are also of great importance, since they can impact the therapeutic outcome. Rapid mucociliary clearance can reduce the corticosteroid residence time at the site of action. Unlike liquid formulations, dry powders have been shown to delay mucociliary clearance, prolonging the contact time between the drug and mucosa. Dry powders are also advantageous because they can assure higher drug concentration at the site of deposition and high formulation stability with no requirement for preservatives. Most of the dry powder formulations are based on mucoadhesive swellable polymers that can additionally prolong drug residence time at the deposition site.[Citation6] In this work, we present the development of corticosteroid-loaded lipid/alginate nanoparticles (NPs) incorporated in pectin-based microspheres with favorable biopharmaceutical properties in relation to targeted drug effect. This dual delivery system was prepared by spray-drying, a well-established method for the preparation of nasal powdered drug delivery systems, that offers the possibility to optimize dry particle characteristics and microencapsulated drug release.[Citation7,Citation8] Pectin and alginate are natural polysaccharides of biocompatible and nonirritant nature. They both show mucoadhesive properties due to interpenetration with mucin chains and hydrogen bond formation.[Citation9] The complex architecture of the delivery system proposed is aimed to ensure balanced swelling, mucoadhesion, and corticosteroid release, with the final aim to provide local therapeutic effect with lower corticosteroid dose, reducing the possibility for systemic side effects.
2. Materials and methods
2.1. Materials
Sodium alginate was purchased from NovaMatrix, Sandivka, Norway. Lecithin S100 was kindly donated from Lipoid, Ludwigshafen, Germany. Di-methyldioctadecylammonum bromide (DDAB) was purchased from Sigma-Aldrich, Germany. Dexamethasone (Dex) was obtained from Sanofi Aventis, Paris, France. Low methylester amidated pectin CF 025 (degree of esterification, 23%–28%; degree of amidation, 22%–25%) was kindly donated by Herbstreith & Fox, Nuenburg, Germany. Acetonitrile (ACN), tetrahydrofurane (THF), and methanol, gradient grade for liquid chromatography, were purchased from Merck, KGaA, Darmstadt, Germany. Spartan regenerated cellulose filters (0.2 μm) were obtained from Whatman, Maidstone, UK.
Simulated nasal fluid (SNF) was prepared as an aqueous solution containing NaCl (150.0 mM; Kemig, Croatia), KCl (40.0 mM; Kemig, Croatia), and CaCl2x2H2O (5.3 mM; Sigma-Aldrich, Germany)[Citation10] and it was used for swelling and in vitro release studies. Hank’s balanced salt solution (HBSS) with 5.3 mM Ca2+ (HBSS-Ca2+; pH 7.4) was prepared by dissolving KCl (5.4 mM), NaHCO3 (4.2 mM), NaCl (136.9 mM), D-glucose monohydrate (5.6 mM; all purchased from Kemig, Croatia), KH2PO4 (0.4 mM; Kemika, Croatia), Na2HPO4x2H2O (0.3 mM; Fluka Chemie AG, Switzerland), and CaCl2x2H2O (5.3 mM; Sigma-Aldrich, Germany) in double-distilled water[Citation11] and was used for cell viability and in vitro permeability studies. All other reagents were of analytical grade and purchased from Kemika, Croatia, and Sigma-Aldrich, Germany. Ultrapure water for chromatographic analyses was produced by Ultra ClearUV Plus (SG Wasseraufbereitung und Regenierstation GmbH, Germany). Mucin type II and gelatin type B from bovine skin were purchased from Sigma Aldrich, Steinheim, Germany. Calf nasal mucosa was obtained from the veterinary service (Turośń Kościelna, Poland). Calf nasal mucosa samples were stored at –20 °C and before the experiment were defrosted and cut into 5 mm in diameter and 2 mm thick pieces.
2.2. Preparation of lipid/alginate NPs
Dex-loaded lipid/alginate NPs were prepared according to the procedure described previously.[Citation11] Briefly, lecithin, DDAB, and Dex were dissolved in 96% ethanol at the concentrations of 25, 2.5, and 5 mg mL−1, respectively. Alginate was solubilized in distilled water at a concentration of 10 mg mL−1 and the aliquot of alginate solution (0.5 mL) was further diluted with distilled water to a volume of 23 mL. Dex-loaded NPs were obtained by the injection of 2 mL of ethanolic lipid/Dex solution into 23 mL of diluted alginate solution under magnetic stirring (900 rpm). The obtained NP suspension was characterized by final alginate concentration of 200 μg mL−1 and lecithin to alginate weight ratio of 10:1. Dex that precipitated during NPs production was separated using 0.45-μm membrane filters (Millipore, Switzerland).
2.3. Physical characterization of the NP size and surface charge
The size and zeta potential of NPs were measured by photon correlation spectroscopy (PCS) using Zetasizer 3000 HS (Malvern Instruments, UK). For this purpose, NP samples were diluted with 0.45-μm filtered distilled water and 10 mM NaCl solution, respectively. Zeta-potential measurements were performed at 25 °C. Samples were placed in the electrophoretic cell, where a potential of 150 mV was established.
2.4. Preparation of spray-dried dex/NP-loaded pectin microspheres
Pectin was dissolved in distilled water at the concentration of 40 mg mL−1. The pectin solution was added dropwise to Dex-loaded NP suspension to the final pectin concentration of 5 mg mL−1. Dex/NP-loaded pectin microspheres were prepared by spray-drying of Dex-loaded NP suspension with pectin in continuous phase using a Mini Spray Dryer Büchi 190 (Flawil, Switzerland) with a standard 0.7 mm nozzle. The drying conditions were as follows: feed flow rate of 0.25 L h−1, compressed air flow rate of 700 NL h−1, aspirator level of 50%, inlet air temperature of 135 °C, and outlet air temperature of 85 °C.
Dex-loaded pectin microspheres without NP were prepared as control. Dex was dissolved in a mixture of distilled water and ethanol. Pectin was dissolved in distilled water at the concentration of 40 mg mL−1 and was added to the Dex solution dropwise to reach the final pectin concentration of 5 mg mL−1. The content of Dex and ethanol were the same as in the NP suspension with pectin. Dex-loaded pectin microspheres were prepared by spray-drying of Dex/pectin solution as described previously.
2.5. Size and zeta-potential of the microspheres
The mean diameter and the size distribution of the microspheres were determined by microscopic imaging analysis with an Olympus BH-2 microscope, equipped with a CCD Camera ICD-42E (Ikegami Tsushinki Co., Japan) and a computer-controlled image analysis system (Optomax V, UK), with at least 3000 particles examined.
The zeta-potential of the microspheres was determined by PCS (Zetasizer 3000HS, Malvern Instruments, UK) at 25 °C. Before the measurement, the microspheres were dispersed in 0.45-μm filtered 10 mM NaCl and placed in the electrophoretic cell, where a potential of 150 mV was established.
2.6. Scanning electron microscopy
The morphology of the microspheres was observed by scanning electron microscopy (SEM) (VEGA3, TESCAN, Czech Republic). The samples were mounted on the specimen holder with a double-sided adhesive tape and were coated with a thin layer of gold and palladium applying a Quorum SC7620 sputter coater (Emitech, UK) under inert argon atmosphere and 10−2 mbar vacuum. The SEM was operated at an acceleration voltage of 5–10 kV.
2.7. Swelling studies
The liquid-absorbing capacity of the microspheres was determined by volumetric method using a Franz diffusion cell apparatus. A water-permeable polyamide membrane (0.45 µm pore size) with microsphere sample (10 mg) was placed between the donor and the receiver cell, which was filled with SNF or distilled water (16 mL). The system was thermostated at 37 °C.
The level of the medium in the sampling port of the receiver compartment was lowering due to the microsphere liquid uptake. At predetermined time intervals, the receiver compartment was replenished with SNF or distilled water up to the starting level. The liquid uptake of the microsphere sample was expressed as the volume of SNF or distilled water added per milligram of the microspheres in 15 min swelling process.
2.8. In vitro release studies
The release of Dex from the spray-dried microspheres was assessed under sink conditions over 5 h using a Franz diffusion cell apparatus, allowing the microspheres to hydrate slowly simulating humid conditions at the nasal mucosa. A water-permeable polyamide membrane with 0.45 µm pore size was placed between the donor and the receiver compartment. The receiver compartment was filled with SNF (16 mL), continuously stirred, and thermostated at 37 °C. The microspheres containing 0.15 mg of Dex were placed on the membrane at the donor side in a uniform layer. At predetermined time intervals, the aliquots (0.5 mL) were withdrawn from the receiver compartment and replaced with fresh SNF. The samples were analyzed for Dex content by ultra-performance liquid chromatography (UPLC) method described below. All the release experiments were performed in triplicate.
2.9. Cell culture conditions
Caco-2 cells (American Type Culture Collection [ATCC], MD) were cultured in Dulbecco’s modified Eagle’s cell culture medium with 4500 mg L−1 glucose, l-glutamine, and sodium bicarbonate, without sodium pyruvate (Sigma-Aldrich, Germany) supplemented with 10% fetal bovine serum (Biosera, France), nonessential amino acids (Lonza, Switzerland), and penicillin/streptomycin/amphotericin B (Lonza, Switzerland). The cells were passaged at 80%–90% confluence. The medium was changed every 48 h. The cultures were maintained at 95% humidity and 37 °C in an atmosphere of 5% CO2.
2.10. Cell viability study
Caco-2 cells were seeded onto 96-well plates (Corning Incorporated Costar, Corning, NY, USA) at a density of 1.5 × 104 cells per well and allowed to reach confluence over 2 days.
Ten milligrams of spray-dried NP-loaded pectin microspheres were homogeneously dispersed in 1.33 mL of distilled water to reach the same Dex concentration as in Dex-loaded NP suspension with 5 mg mL−1 of pectin in the continuous phase[Citation11]. The dispersion was further diluted with HBSS-Ca2+ (pH 7.4) 2, 4, 8, and 16 times. Before the treatment with the microspheres, the cell culture medium was withdrawn, the cells were washed with HBSS-Ca2+, and exposed to different concentrations of the microspheres for 2 h at 37 °C. The cells incubated in HBSS-Ca2+ were used as a negative control. After 2 h of treatment with the microspheres, the cells were washed two times with HBSS-Ca2+ and incubated with fresh medium for 24 h. The cell viability was determined with the colorimetric MTT (3-[4,5-dimethylthiazol-2-yl]-2,5-diphenyl tetrazolium bromide, Sigma-Aldrich, Germany) assay. A total of 10 μL of MTT stock solution (5 mg mL−1) in phosphate-buffered saline (Lonza, Switzerland) was added to each well, and the cells were then incubated for 1 h at 37 °C. Afterwards, the medium was removed, the cells were lysed, and the formazan crystals dissolved by the addition of 100 µL of isopropanol per well. The amount of formazan was quantified spectrophotometrically at 570 nm (1420 Multilabel counter VICTOR3, Perkin Elmer, Waltham, MA, USA).
2.11. In vitro mucoadhesion test
Evaluation of mucoadhesive properties of the microspheres was performed using TA.XT. Plus Texture Analyzer (Stable Micro Systems, UK) and three different models of mucoadhesive layer: gelatin disc, mucin gel, and calf nasal mucosa. Experimental parameters of the process were chosen during preliminary tests and set as follows: pretest speed 0.5 mm s−1, test speed 0.1 m s−1, contact time 180 s, post-test 0.1 mm s−1, and applied force 1 N. The gelatin discs were made by pouring 30% (w/w) aqueous solution of gelatin onto a Petri dish. After cooling and solidification at room temperature, the gelatin layer was cut into discs with 5 mm diameter. The layer of mucin was prepared by sorbing 10% mucin gel on discs with cellulose fiber (5 mm in diameter). All tests were conducted at 37 ± 1 °C. Adhesive layers were adhered to an upper probe using a cyanoacrylate glue. The quantity of 20 mg of microsphere samples (Dex-loaded pectin microspheres used as control and Dex/NP-loaded pectin microspheres) was moisturized with 20 μL of SNF. The mucoadhesive properties were determined as the maximum detachment force (Fmax) and the work of adhesion (Wad)—calculated from the area under the force versus distance curve, expressed in μJ. The work of adhesion (Wad) was calculated by using the following formula:
where A is the area under the force versus distance curve, multiplication by 0.1 represents the conversion time measurement to distance (the sampler was raised at 0.1 mm s−1), then multiplication by 1000 to express the result in units of work (μJ).
2.12. In vitro permeability through epithelial model barrier
In vitro permeability studies using Caco-2 cell monolayer were performed as described previously.[Citation11] Caco-2 cells were seeded onto the polycarbonate 12-well Transwell inserts (0.4 μm mean pore size and 1.12 cm2 surface area, Corning Costar Inc., Corning, NY, USA) at a density of 2.5 × 104 cells per well and grown for 21 days. The cells were cultured with the cell culture medium volume of 0.5 mL in the apical and 1.5 mL in the basolateral compartment. The cell culture medium was changed every other day and 24 h before the permeability experiment. The transepithelial electrical resistance of the monolayers was measured using an epithelial volt/ohm meter EVOM equipped with STX-2 chopstick electrode (WPI Inc., Sarasota, FL, USA) to determine the formation of the monolayers and their integrity. The permeability studies were carried out in HBSS-Ca2+. The tested sample was Dex/NP-loaded pectin microspheres, which was prepared by homogeneously dispersing 10 mg of the microspheres in 1.33 mL of distilled water. The dispersion was further diluted with HBSS-Ca2+ in 1:1 ratio, to reach the final Dex concentration of 112 µg mL−1. Dex-loaded pectin microspheres, Dex suspension in HBSS-Ca2+ (112 μg mL−1), and Dex solution in HBSS-Ca2+ (60 μg mL−1) were used as controls. Dex-loaded pectin microspheres (10 mg) were dispersed in 1.93 mL of distilled water. The dispersion was further diluted with HBSS-Ca2+ in 1:1 ratio, to reach the final Dex concentration of 112 µg mL−1. Before the experiment, the monolayers were washed with HBSS-Ca2+, after which HBSS-Ca2+ was placed into the apical (0.5 mL) and basolateral (1.5 mL) compartments. The cell monolayers were then incubated for 30 min at 37 °C. At the beginning of the experiment, the apical compartment was emptied and 0.5 mL of the tested sample or control was added. Samples (0.4 mL) were taken from the basolateral compartment at regular time intervals over 120 min and replaced with the same volume of fresh thermostated HBSS-Ca2+ (37 °C). During the permeability experiment, the monolayers were incubated at 37 °C and 50 rpm on a horizontal orbital shaker. All the experiments were carried out in pentaplicate. The samples were analyzed for Dex content using UPLC (see Physical characterization of the NP size and surface charge section). The apparent permeability coefficient (Papp) was calculated according to the following equation[Citation12]:
where A is the surface area of the permeation barrier, t is the time at the end of permeability experiment, V1 and V2 are the media volumes in the apical and basolateral compartment, respectively, C0 is the initial concentration of Dex in the apical compartment, C1,t and C2,t are the final concentrations of Dex in the apical and basolateral compartment, respectively.
2.13. Quantitave determination of Dex
The quantitative determination of Dex was performed by UPLC, as described previously.[Citation11] The chromatographic separation of Dex was carried out on the Waters Acquity UPLC BEH Shield RP18 column (2.1 × 100 mm, 1.7 μm particle size), using Agilent Infinity 1290 (Agilent Technologies, Santa Clara, CA, USA) and Waters ACQUITY UPLC H Class (Waters Corporation, Milford, MA, USA) instrument.
The quantitative determination of Dex in the samples from the basolateral compartments in the permeability studies and the samples from the receiver compartments in the in vitro release studies was performed on Waters ACQUITY UPLC H Class instrument using isocratic UPLC method (5 mM sodium acetate buffer (pH 4.5)/ACN: 68/32% (v/v), 55 °C, 0.7 mL min−1, and 254 nm detection wavelength, 10 μL injection volume).
The determination of Dex loading in the spray-dried microspheres was performed on Agilent Infinity 1290 instrument using gradient UPLC method (5 mM sodium acetate buffer (pH 4.5)/ACN: 50/50% (v/v) at 0 min, 25/75% at 0.2 min, 0/100% at 1.0 min; 55 °C, 0.7 mL min−1, 254 nm detection wavelength, 10 μL injection volume). The samples were accurately weighed in 25 mL volumetric flasks, followed by the addition of 2 mL of THF and 10 mL of diluent (methanol/ultrapure water, 60/40% (v/v)). After 20 min of sonication, the samples were filled with diluent up to the total volume of 25 mL.
Before analysis, all the samples were filtrated through 0.2 μm Spartan regenerated cellulose filters. For each sequence standard solutions were prepared in duplicate and injected alternately. At least five standard solution injections were given in each injection sequence. System suitability was evaluated according to the following criteria: relative standard deviation of the detector response factor for all standard solution injections in the sequence is not more than 3.0%, and tailing factor of Dex peak is not more than 2.0.
3. Results and discussion
3.1. Preparation and characterization of dex/NP-loaded pectin microspheres
Conventional nasal formulations such as solutions and suspensions are related to limited residence time at the nasal mucosa. The most promising concept offering prolonged formulation retention within the nasal cavity refers to the development of in situ gelling systems, based on either polymeric solutions that form gel under physiological conditions (e.g. due to temperature increase or ionic crosslinking[Citation13]) or polymeric microspheres that swell (i.e. form hydrogel) in the contact with mucous layer.[Citation14]
Based on our previous study on physicochemical characteristics, in vitro release profile and biocompatibility of Dex-loaded lipid-alginate NPs,[Citation11] those with DDAB/lecithin weight ratio of 1:10 (DN) were chosen for preparation of Dex/NP-loaded pectin microspheres for Dex nasal delivery. The main NP characteristics were consistent with those previously reported, confirming batch-to-batch repeatability. Briefly, DN NPs were characterized by mean diameter of 261.4 ± 5.7 nm, polydispersity index of 0.296, and zeta-potential of -32.5 ± 0.6 mV. Dex content in the NP suspension was 241.2 ± 6.1 μg mL−1.
Dex/NP-loaded pectin microspheres (DNM) were prepared by spray-drying of Dex-loaded NPs dispersed in pectin solution. Dex-loaded pectin microspheres (DM) were prepared by spray-drying of Dex dispersed in pectin solution and served as control. The main characteristics of the spray-dried microspheres are given in . Both systems were characterized by relatively high process yield (45-70%) that depended on the volume of spray-dried feed (100–800 mL). DNM were characterized by larger mean diameter (2.76 ± 0.10 µm) in comparison with DM (2.17 ± 0.01 µm), while there was no significant difference in zeta-potential of compared microspheres (–36.2 ± 1.1 mV vs. –36.8 ± 1.3 mV for DNM and DM, respectively). Spray-drying of both, Dex-loaded NP dispersion in pectin solution, as well as Dex dispersion in pectin solution, resulted in microspheres with high entrapment efficiency, yielding the drug loading of 3.3 ± 0.3 and 3.8 ± 0.9%, respectively.
Table 1. The main characteristics of Dex/NP-loaded pectin microspheres (DNM) and Dex-loaded pectin microspheres (DM).
The size range of DNM and DM microspheres corresponds to the size range of nasal powders produced previously by spray-drying technique.[Citation15–17] Such microparticles agglomerate spontaneously due to the high surface area to volume ratio and increased cohesive forces, thus falling in the range (≥ 10 μm) that allows for adequate nasal deposition.[Citation18]
DNM and DM microspheres were characterized by high entrapment efficiency, showing no significant difference in drug content (3.3 ± 0.3 and 3.8 ± 0.9%, respectively). Considering the targeted daily dose of Dex (400–800 µg[Citation3]) and the quantity of powder that can be administered per nostril per shot (about 10–25 mg[Citation18]), the obtained drug content within the microspheres is sufficient to enable nasal administration of Dex therapeutic dose. In addition, complex carrier developed to improve the Dex bioavailability/therapeutic effect, acts at the same time as a filler excipient that contributes largely to the total mass of the powder to be delivered, ensuring accurate Dex dosing.[Citation18]
3.2. Microsphere morphology
SEM images of DNM and DM microspheres are shown in . DM microspheres are of ideal spherical shape with slightly rough surface but no visible pores and surface discontinuities. On the contrary, DNM microspheres are wrinkled, that is, characterized by irregular surface forming external voids. Irregular particle morphology is usually associated with high values of Peclet number, which correlates convective and diffusive transport phenomena.[Citation19] However, the addition of surfactant has also been recognized as one of the crucial parameters affecting the morphology of particles.[Citation20] In this study, irregular particle morphology of DNM microspheres could be related to the presence of surface active lipids (used for the preparation of lipid/alginate NPs) within the spray-drying feed. It has already been reported that a decrease in the surface tension by introducing a surfactant into the aqueous system to be spray-dried results in a less stable droplet, and thus a “mushroom-like” form or “double convex disc” form can be obtained.[Citation20]
3.3. Swelling properties of Dex/NP-loaded pectin microspheres
Controlled swelling and hydrogel formation is a key characteristic for dry powders to be nasally applied. Hydration and swelling are both polymer—and environment—related factors. Overhydration causes extended swelling, resulting in slippery mucilage formation and reducing its adhesive strength.[Citation21]
Both DNM and DM microspheres absorbed significantly higher volumes of distilled water per milligram of microspheres (95.5 ± 3.4 and 102.0 ± 10.7 µL mg−1, respectively) compared with SNF (37.8 ± 5.3 and 49.8 ± 3.9 µL mg−1, respectively). The moderate swelling of pectin microspheres in biorelevant SNF is the result of cross-linking of pectin with divalent calcium ions present in SNF.[Citation22] The inverse relationship between the polymer cross-linking and swelling degree is well known.[Citation6]
Moderate swelling is expected to prolong the residence time of swollen microspheres at the deposition site. In addition, it could result in avoidance of potential mucosal damages or inconveniences to the patient.[Citation23]
Mahajan et al. have studied the swelling capacity of pectin-based microspheres intended for nasal delivery of ondansetron using phosphate buffer (pH 6.6) as the swelling medium.[Citation14] The swelling ability of the pectin-based microspheres was shown to be related to the pectin content in the preparation. However, the selected medium could not trigger the divalent-ion cross-linking of pectin, which is expected to occur in the biorelevant conditions.
In this study, slightly higher swelling medium uptake was observed for DM microspheres when compared with DNM microspheres (). However, when swelling ability was expressed as the volume of medium absorbed per milligram of pectin, DNM microspheres were shown to swell more extensively than DM microspheres, especially in the absence of Ca2+-induced pectin crosslinking (i.e. in distilled water; 139.9 ± 5.0 vs. 106.6 ± 11.2 µL mg−1, respectively; ). A moderate difference in size between DNM and DM microspheres (2.76 ± 0.10 vs. 2.17 ± 0.01 μm, respectively) could be expected to influence only the burst swelling, rather than the total swelling capacity. Namely, DM microspheres were characterized by higher total surface area in comparison with DNM microspheres. Accordingly, in the first minute of microspheres swelling in SNF, DNM microspheres absorbed 29.6 ± 5.9 µL SNF per mg of pectin, while DM microspheres in the same period absorbed about 20% larger SNF volume (35.7 ± 8.2 µL mg−1 of pectin). However, 15-min swelling process yielded the higher SNF volume absorbed per milligram of pectin by DNM microspheres in relation with DM microspheres, showing their higher swelling ability. Therefore, it may be concluded that entrapment of Dex within the lipid/alginate NPs reduced its influence on the swelling capacity of the pectin matrix in relation with Dex being directly entrapped within the conventional pectin microspheres.
3.4. In vitro Dex release
The profiles of in vitro Dex release from DNM and DM microspheres in SNF are shown in .
Figure 3. The release profiles of Dex from Dex/NP-loaded pectin microspheres (DNM; •) and Dex-loaded pectin microspheres (DM; ▴) in SNF at 37 °C. The release profiles were assessed using Franz diffusion cell. The profile of Dex diffusion from the solution across the water-permeable polyamide membrane (▪) is also presented. Q represents cumulative amount of Dex released at time t. Data are expressed as the mean ± SD (n = 3).
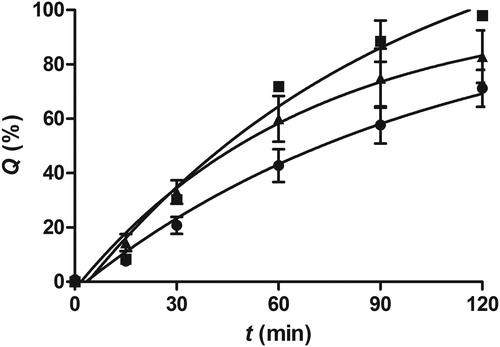
Dex release profiles from the spray-dried microspheres was determined using Franz diffusion cell apparatus with SNF as the receptor medium, triggering the cross-linking of pectin with the Ca2+ ions present in SNF. The experimental setup also allowed for slow microsphere hydration mimicking the humid conditions at the nasal mucosa. The profile of Dex diffusion from the solution in SNF across the water-permeable polyamide membrane was also determined, with the aim to assess the influence of resistance of the polyamide membrane to Dex diffusion on the overall Dex release rate. DNM microspheres were characterized by prolonged Dex release and a lower release rate than that observed for DM microspheres and Dex solution (). The results obtained indicated that Dex entrapment into lipid/alginate NPs before the incorporation into pectin matrix ensured prolonged Dex release and provided the potential for prolonged Dex delivery in vivo.
The results obtained are consistent with our previous findings related to the in vitro release of Dex from NP-loaded pectin in situ gel, suggesting that Dex release from NP is the rate-limiting step that governed the overall Dex release profile from NP-loaded gel.[Citation11]
3.5. Evaluation of Dex/NP-loaded pectin microsphere mucoadhesion
Mucociliary clearance that limits the contact time between the drug and the mucosal surface has been recognized as one of the most critical factors in nasal drug delivery system design.[Citation13] One of the approaches to improve the therapeutic effect of nasally applied drugs is to increase the drug residence time within the nasal cavity by designing mucoadhesive drug delivery systems. In this study, mucoadhesion test was performed to determine the adhesive strength of the pectin based microspheres, simulating in vivo conditions by microspheres swelling in SNF and using relevant mucoadhesive layers in addition to gelatin disc, such as mucin gel and calf nasal mucosa. The results obtained are given in .
Table 2. Mucoadhesion of Dex/NP-loaded pectin microspheres (DNM) and Dex-loaded pectin microspheres (DM).
It has been resumed in the literature that polymer mucoadhesion properties are affected by its swelling properties, hydration rate, molecular weight, functional groups, molecular conformation, chain flexibility and mobility, allowing different mechanisms of mucoadhesion under relevant physiological conditions.[Citation24] Recent studies have shown the prominent mucoadhesive potential of low methylester pectin,[Citation25] confirming hydrogen bonding between pectin free carboxylic groups and mucin as one of the dominating pectin mucoadhesion mechanisms.[Citation26]
In this study, mucoadhesion of the pectin microspheres was affected by the presence of the lipid/alginate NPs as Dex carriers within the pectin matrix. Namely, the work of adhesion for DNM microspheres was 2.2, 1.5 and 1.7 fold higher than the work of adhesion for DM microspheres tested on the gelatin disc, mucin gel, and calf nasal mucosa, respectively. At the same time, maximal detachment force for DNM microspheres was 2.4, 1.9, and 1.1-fold higher than the maximal detachment force for DM microspheres, tested on the same mucoadhesive layers. The stronger mucoadhesion of DNM microspheres in relation with DM microspheres can partially be explained by the entrapment of Dex within the lipid/alginate NPs that reduced its influence on the wetting and swelling capacity of the pectin matrix, in relation with Dex being directly entrapped within the conventional pectin microspheres. It has been previously reported that faster hydration of polymer allows for greater interaction and entanglement of the polymer chains with mucin resulting in higher mucoadhesive force.[Citation24] In addition, the presence of surfactants can influence the entanglement of the polymer chains. Namely, it was shown that pectin forms complexes with different types of surfactants and that the morphology of the complexes formed exhibits different structures: from random coil structures to rod-like or extended random coil structures, depending on the type of surfactant and its concentration.[Citation27]
Finally, it can be assumed that the larger contact surface area of the wrinkled DNM microspheres contributed to their higher mucoadhesion in comparison with the conventional spherical DM microspheres. Peppas and coworkers already postulated that coarse and wrinkled surface of microparticles might improve their mucoadhesion in vivo.[Citation28]
Mucus layer covering the nasal mucosa triggered the development of various mucoadhesive dosage forms that ensured prolonged retention at the administration site and increased bioavailability of the nasally administered drug.[Citation14,Citation29–31] However, there is also an opposite strategy of the particulate delivery systems design focusing on the enhancement of particles penetration across the mucus gel layer to reach the underlying epithelium. According to the SWOT analysis of mucoadhesive and mucopenetrating particulate delivery systems, none of the two strategies can be considered superior over the other when it comes to mucosal drug delivery. In fact, novel trends based on combining both strategies in the same delivery system have been proposed.[Citation32]
The size and the surface characteristics of the spheres determine their interaction with and penetration through the mucus layer covering the epithelium. The particles in micrometer range stay at the surface of the mucus layer, being too large to enter the mucin fiber mesh spaces. Nanoparticles with the size range of 200–500 nm can penetrate through the mucus, depending on the mucus type and adhesive interactions.[Citation9] Recent studies in the field of mucosal drug delivery revealed that neutral polyethylene glycol (PEG)-coated nanosystems are able to penetrate mucus barrier efficiently, eliminating the adhesive interactions between the NPs and mucus, thus assuring closer contact with the epithelium.[Citation33]
In this work, NP-loaded pectin microspheres, due to their size, are expected to get trapped at the surface of the mucus layer and form a gel layer upon swelling. Therefore, the main concept to optimize the delivery system performance within this study was based on the mucoadhesiveness of the pectin used for the production of microcarrier system. However, further investigations could be directed toward the PEGylation of the NPs surface to explore how such modification would influence Dex release and NP diffusivity within both, the swollen pectin gel and mucus layer, while moving toward the epithelium surface.
3.6. Evaluation of Dex/NP-loaded pectin microsphere biocompatibility
Pectin is well known for its biocompatibility, being denoted as “generally regarded as safe” due to its long-term use in pharmaceutical and food industry.[Citation34] PecSys, an in situ gelling technology based on low-methoxy pectin, is used in an innovative nasal fentanyl formulation named PecFent.[Citation35] In our previous study, lipid/alginate NP-loaded pectin gel was proven to be biocompatible with the selected epithelial cell model. In this study, spray-dried NP-loaded pectin microspheres were also shown to be biocompatible. As expected, no cytotoxic effect was observed under the experimental conditions used. Namely, the viability of the cells exposed to DNM suspensions at pectin concentration ranging from 0.2 to 2.5 mg mL−1 varied between 100.5 ± 1.9% and 106.1 ± 2.3% (data not shown). These results served as the basis for the in vitro permeability studies to be conducted within the safe microsphere concentration range.
3.7. In vitro permeability through epithelial model barrier
The permeation of Dex across the Caco-2 monolayer was assessed to screen the influence of NP-loaded pectin microspheres on transmucosal Dex absorption and related systemic adverse effects. DM suspension as well as Dex solution and suspension in HBSS served as controls. The results obtained are shown in . The Papp value for Dex solution was comparable with the value reported in our previous study.[Citation11] As expected, Dex permeation from the suspension was lower than from the solution, owing to the non-dissolved fraction of the drug. For Dex suspension and DM suspension with the same Dex concentration, similar Papp values were obtained, suggesting that in both cases Dex permeation across the epithelial model barrier was determined by its dissolution rate, even though in the case of DM microspheres Dex needed to diffuse through the pectin gel layer of the swollen DM microspheres. Dex/NP-loaded pectin microspheres were characterized by the highest Dex permeation () with attenuation factor of 1.17 in relation to Dex solution. It is interesting to compare these results with those previously reported for NP-loaded pectin gel that was shown to reduce Dex permeation across the epithelial model barrier (attenuation factor of 0.81[Citation11]). We have previously shown that Dex permeation could partially be related to cell internalization of Dex loaded NPs, and that such process might be hindered by NP incorporation into pectin gel (attenuation factor of NP suspension vs. NP-loaded pectin gel 0.94 vs. 0.81). However, contrary to the in situ forming pectin gel with homogeneously dispersed Dex-loaded NPs, the swollen pectin microspheres seemed to provide more intimate contact with the epithelial barrier and higher concentration gradient at the site of absorption, acting as a drug reservoir at the cell monolayer surface, resulting in Dex Papp value comparable with that of Dex solution. Nevertheless, it must be considered that such drug reservoir effect at the mucosal surface could at the same time be beneficial since it could lead to improved therapeutic outcome.
Table 3. Permeation of Dex across Caco-2 monolayer from DNM suspension, DM suspension, Dex solution (60 µg mL−1), and Dex suspension (112 µg mL−1) in HBSS-Ca2+ presented by apparent permeability coefficients (Papp).
4. Conclusions
In this study, we propose the spray-dried NP-loaded pectin microspheres as Dex nasal delivery system for the effective treatment of chronic rhinosinusitis with nasal polyps. In general, dry powders ensure higher stability over liquid formulations excluding the need for preservatives and thus increasing the safety of drug administration. However, the proposed system offers other advantages over liquid formulations such as potential for prolonged contact time with nasal mucosa and prolonged therapeutic effect, by balancing swelling, mucoadhesion, and Dex release in contact with nasal fluid. Further development of nasal powder formulation requires concomitant consideration of an appropriate delivery device since combined administration and formulation parameters determine the potential for drug delivery beyond the nasal valve, that is, to the site where the polyps occur.
Acknowledgments
The authors thank Matija Gretić (University of Zagreb Faculty of Chemical Engineering and Technology, Zagreb, Croatia) for help with SEM analysis of the microsphere samples.
Additional information
Funding
References
- Ocampo, C. J.; Grammer, L. C. Chronic Rhinosinusitis. J. Allergy Clin. Immunol. Pract. 2013, 1, 205–211. DOI:10.1016/j.jaip.2012.12.001.
- Mullol, J.; Obando, A.; Pujols, L.; Alobid, I. Corticosteroid Treatment in Chronic Rhinosinusitis: The Possibilities and the Limits. Immunol. Allergy Clin. North Am. 2009, 29, 657–668. DOI:10.1016/j.iac.2009.07.001.
- Martino, B. J.; Church, C. A.; Seiberling, K. A. Effect of Intranasal Dexamethasone on Endogenous Cortisol Level and Intraocular Pressure. Int. Forum Allergy Rhinol. 2015, 5, 605–609. DOI:10.1002/alr.21514.
- Hansen, F. S.; Djupesland, P. G.; Fokkens, W. J. Preliminary Efficacy of Fluticasone Delivered by a Novel Device in Recalcitrant Chronic Rhinosinusitis. Rhinology 2010, 48, 292–299. DOI:10.4193/Rhino09.178.
- Vlckova, I.; Navrátil, P.; Kaňa, R.; Pavlicek, P.; Chrbolka, P.; Djupesland, P. G. Effective Treatment of Mild-to-Moderate Nasal Polyposis with Fluticasone Delivered by a Novel Device. Rhinology 2009, 47, 419–426. DOI:10.4193/Rhin09.024.
- Pereswetoff-Morath, L. Microspheres as Nasal Drug Delivery Systems. Adv. Drug Deliv. Rev. 1998, 29, 185–194. DOI:10.1016/S0169-409X(97)00069-0.
- Ré, M.-I. Formulating Drug Delivery Systems by Spray Drying. Dry. Technol. 2006, 24, 433–446. DOI:10.1080/07373930600611877.
- Tsotsas, E. Influence of Drying Kinetics on Particle Formation: A Personal Perspective. Dry. Technol. 2012, 30, 1167–1175. DOI:10.1080/07373937.2012.685139.
- Sosnik, A.; das Neves, J.; Sarmento, B. Mucoadhesive Polymers in the Design of Nano-Drug Delivery Systems for Administration by Non-Parenteral Routes: A Review. Prog. Polym. Sci. 2014, 39, 2030–2075. DOI:10.1016/j.progpolymsci.2014.07.010.
- Martinac, A.; Filipović-Grčić, J.; Voinovich, D.; Perissutti, B.; Franceschinis, E. Development and Bioadhesive Properties of Chitosan-Ethylcellulose Microspheres for Nasal Delivery. Int. J. Pharm. 2005, 291, 69–77. DOI:10.1016/J.IJPHARM.2004.07.044.
- Jurišić Dukovski, B.; Plantić, I.; Čunčić, I.; Krtalić, I.; Juretić, M.; Pepić, I.; Lovrić, J.; Hafner, A. Lipid/Alginate Nanoparticle-Loaded in Situ Gelling System Tailored for Dexamethasone Nasal Delivery. Int. J. Pharm. 2017, 533, 480–487. DOI:10.1016/j.ijpharm.2017.05.065.
- Cussler, E. L. Diffusion: Mass Transfer in Fluid Systems, 2nd Ed.; Cambridge University press, 1997.
- Karavasili, C.; Fatouros, D. G. Smart Materials: In Situ Gel-Forming Systems for Nasal Delivery. Drug Discov. Today 2016, 21, 157–166. DOI:10.1016/j.drudis.2015.10.016.
- Mahajan, H. S.; Tatiya, B. V.; Nerkar, P. P. Ondansetron Loaded Pectin Based Microspheres for Nasal Administration: In Vitro and In Vivo Studies. Powder Technol. 2012, 221, 168–176. DOI:10.1016/j.powtec.2011.12.063.
- Alhalaweh, A.; Andersson, S.; Velaga, S. P. Preparation of Zolmitriptan–Chitosan Microparticles by Spray Drying for Nasal Delivery. Eur. J. Pharm. Sci. 2009, 38, 206–214. DOI:10.1016/j.ejps.2009.07.003.
- Karavasili, C.; Bouropoulos, N.; Sygellou, L.; Amanatiadou, E. P.; Vizirianakis, I. S.; Fatouros, D. G. PLGA/DPPC/Trimethylchitosan Spray-Dried Microparticles for the Nasal Delivery of Ropinirole Hydrochloride: In Vitro, Ex Vivo and Cytocompatibility Assessment. Mater. Sci. Eng. C 2016, 59, 1053–1062. DOI:10.1016/J.MSEC.2015.11.028.
- Saladini, B.; Bigucci, F.; Cerchiara, T.; Gallucci, M. C.; Luppi, B. Microparticles Based on Chitosan/Pectin Polyelectrolyte Complexes for Nasal Delivery of Tacrine Hydrochloride. Drug Deliv. And Transl. Res. 2013, 3, 33–41. DOI:10.1007/s13346-012-0086-y.
- Tiozzo Fasiolo, L.; Manniello, M. D.; Tratta, E.; Buttini, F.; Rossi, A.; Sonvico, F.; Bortolotti, F.; Russo, P.; Colombo, G. Opportunity and Challenges of Nasal Powders: Drug Formulation and Delivery. Eur. J. Pharm. Sci. 2018, 113, 2–17. DOI:10.1016/J.EJPS.2017.09.027.
- Vehring, R. Pharmaceutical Particle Engineering via Spray Drying. Pharm. Res. 2008, 25, 999–1022. DOI:10.1007/s11095-007-9475-1.
- Iskandar, F.; Gradon, L.; Okuyama, K. Control of the Morphology of Nanostructured Particles Prepared by the Spray Drying of a Nanoparticle Sol. J. Colloid Interface Sci 2003, 265, 296–303. DOI:10.1016/S0021-9797(03)00519-8.
- Ugwoke, M. I.; Verbeke, N.; Kinget, R. The Biopharmaceutical Aspects of Nasal Mucoadhesive Drug Delivery. J. Pharm. Pharmacol. 2001, 53, 3–22. DOI:10.1211/0022357011775145.
- Tho, I.; Arne Sande, S.; Kleinebudde, P. Cross-Linking of Amidated Low-Methoxylated Pectin with Calcium During Extrusion/Spheronisation: Effect on Particle Size and Shape. Chem. Eng. Sci. 2005, 60, 3899–3907. DOI:10.1016/J.CES.2005.02.023.
- Gavini, E.; Rassu, G.; Muzzarelli, C.; Cossu, M.; Giunchedi, P. Spray-Dried Microspheres Based on Methylpyrrolidinone Chitosan as New Carrier for Nasal Administration of Metoclopramide. Eur. J. Pharm. Biopharm. 2008, 68, 245–252. DOI:10.1016/J.EJPB.2007.05.002.
- Sensoy, D.; Cevher, E.; Sarıcı, A.; Yılmaz, M.; Özdamar, A.; Bergişadi, N. Bioadhesive Sulfacetamide Sodium Microspheres: Evaluation of Their Effectiveness in the Treatment of Bacterial Keratitis Caused by Staphylococcus aureus and Pseudomonas aeruginosa in a Rabbit Model. Eur. J. Pharm. Biopharm. 2009, 72, 487–495. DOI:10.1016/J.EJPB.2009.02.006.
- Joergensen, L.; Klösgen, B.; Simonsen, A. C.; Borch, J.; Hagesaether, E. New Insights into the Mucoadhesion of Pectins by AFM Roughness Parameters in Combination with SPR. Int. J. Pharm. 2011, 411, 162–168. DOI:10.1016/J.IJPHARM.2011.04.001.
- Hagesaether, E.; Sande, S. A. In Vitro Measurements of Mucoadhesive Properties of Six Types of Pectin. Drug Dev. Ind. Pharm. 2007, 33, 417–425. DOI:10.1080/03639040600920630.
- Joshi, N.; Rawat, K.; Bohidar, H. B. Influence of Structure, Charge, and Concentration on the Pectin–Calcium–Surfactant Complexes. J. Phys. Chem. B 2016, 120, 4249–4257. DOI:10.1021/acs.jpcb.6b00016.
- Peppas, N. A.; Buri, P. A. Surface, Interfacial and Molecular Aspects of Polymer Bioadhesion on Soft Tissues. J. Control. Release 1985, 2, 257–275. DOI:10.1016/0168-3659(85)90050-1.
- Abdel Mouez, M.; Zaki, N. M.; Mansour, S.; Geneidi, A. S. Bioavailability Enhancement of Verapamil HCl via Intranasal Chitosan Microspheres. Eur. J. Pharm. Sci. 2014, 51, 59–66. DOI:10.1016/J.EJPS.2013.08.029.
- Zaki, N. M.; Awad, G. A.; Mortada, N. D.; Abd ElHady, S. S. Enhanced Bioavailability of Metoclopramide HCl by Intranasal Administration of a Mucoadhesive In Situ Gel with Modulated Rheological and Mucociliary Transport Properties. Eur. J. Pharm. Sci. 2007, 32, 296–307. DOI:10.1016/J.EJPS.2007.08.006.
- Wang, Y.; Li, M.; Qian, S.; Zhang, Q.; Zhou, L.; Zuo, Z.; Lee, B.; Toh, M.; Ho, T. Zolpidem Mucoadhesive Formulations for Intranasal Delivery: Characterization, in Vitro Permeability, Pharmacokinetics, and Nasal Ciliotoxicity in Rats. J. Pharm. Sci. 2016, 105, 2840–2847. DOI:10.1016/J.XPHS.2016.03.035.
- Netsomboon, K.; Bernkop-Schnürch, A. Mucoadhesive vs. mucopenetrating Particulate Drug Delivery. Eur. J. Pharm. Biopharm. 2016, 98, 76–89. DOI:10.1016/J.EJPB.2015.11.003.
- Huckaby, J. T.; Lai, S. K. PEGylation for Enhancing Nanoparticle Diffusion in Mucus. Adv. Drug Deliv. Rev. 2018, 124, 125–139. DOI:10.1016/J.ADDR.2017.08.010.
- Watts, P.; Smith, A. PecSys: In Situ Gelling System for Optimised Nasal Drug Delivery. Expert Opin. Drug Deliv. 2009. DOI:10.1517/17425240902939135.
- Watts, P.; Smith, A.; Perelman, M. Nasal Delivery of Fentanyl. Drug Deliv. Transl. Res. 2013, 3, 75–83. DOI:10.1007/s13346-012-0078-y.