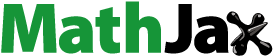
Abstract
To respond to the various issues of conventional drying (textural defects of collapse-shrinkage and case hardening, microbiological contamination, poor rehydrability, degradation of active molecules caused by high wet-bulb temperature, weak drying kinetics, and marketing difficulty), swell-drying has proven high relevance in combining conventional drying processes with the Instant Controlled Pressure Drop (D.I.C.). The international team of "Research & Engineering Platform for intensifying Drying Processes (REPID)" has studied phenomenological models and experimental trials combining D.I.C. with new Interval Hyper-Active Drying (IHAD) processes. Currently, IHAD includes interval starting accessibility drying (ISAD), interval infrared airflow drying (IIRAD), and interval microwave airflow drying (IMAD). All these "interval operations" are sandwiched into cycles, each comprising double separate independent mechanisms; a short, hyper-focused active period (that generates and sweeps out vapor to the surrounding environment) alternated by a passive period of internal water diffusion/moisture homogenization. Since surface evaporation is highly intensive, the wet-bulb temperature stays low, although highly effective drying kinetics. Thus, the operation results in avoiding biochemical damage risks. Coupled with the D.I.C. texturing-decontamination, these drying operations are very effective in terms of drying kinetics, energy consumption, and product quality of heat-sensitive solids. It has led to the manufacturing of effective drying equipment.
Introduction
Although the drying operation is one of the oldest preservation processes of various food products, critical issues of conventional drying processes (airflow, infrared, contact heating, microwaves, Etc.) have persisted. Regarding product quality, the main issues are collapse/shrinkage, microbiological contamination, degradation of active molecules, and low functional properties and kinetics. The low drying kinetics and high energy consumption could explain the weak performance of the drying process.[Citation1–3]
Then, to enhance drying operation, it is crucial to thoroughly understand the origin of each issue to advise adequate technological solutions.
Firstly, a low internal effective water diffusion mainly characterizes the natural products because of the weak permeability of the cell membranes. Water diffusivity significantly decreases during dehydration because of shrinkage, collapse, and compactness.[Citation4] Thus, the conventional drying processes always require high energy consumption, and the last stage causes a high bulb temperature level and an evident thermal degradation. Only freeze-drying (FD) ensures the matrix structure stability of the dried fruit and vegetable. Thanks to freezing/sublimation, it implies a high porosity, a great diffusion of water within the material, and an exciting rehydration process.[Citation5] Then, freeze-drying preserves the initial structure of the biological material and prevents shrinkage and collapse. However, it has very low evaporation kinetics by sublimation, low thermal conductivity, and too weak external surrounding vapor pressure. It is also one of the highest energy-consuming dehydration operations.[Citation6]
Secondly, one of the main issues regarding the final quality of conventionally dried products relates to the possible risks of various types of microbiological contamination. As Chitrakar et al.[Citation7] concluded that dried foods are not inherently microbiologically safe, and it is necessary to overhaul the process chain to achieve microbial safety. Indeed, although drying can prevent the growth of vegetative microorganisms and reduce the risks of microbial proliferation, some microorganisms often remain spores at the operation’s end.[Citation8] Some examples of these microorganisms are Aspergillus flavus and Aspergillus parasiticus molds, which could generate high carcinogenicity mycotoxins, usually when residual moisture exists after conventional drying.[Citation9] Thus, the drying operation should occur just after harvesting to ensure efficiency. However, it is essential to include an exceptionally effective decontamination process, whether industrial or artisanal conventional drying or freeze-drying. It must destroy microorganisms, molds, insects, and larvae while preserving the nutritional and organoleptic quality.
Thirdly, the interest in preserving food products requires the maintenance of nutritional and functional attributes. Despite the apparent interest in preserving a low wet-bulb temperature in conventional drying processes, the weak evaporation rate leads to an extended drying time, which could lead to excessive degradation of various nutritional and active sensory molecules.[Citation10,Citation11] Besides, except for freeze-drying, the rubbery behavior of the material inevitably generates shrinkage and collapse of the structure. They affect the final functional qualities of conventional dried foods as low rehydration kinetics and a weak water holding capacity (WHC),[Citation12] which cut their use in instant food products such as soups.
Finally, since the internal and external transfer mechanisms (heat and mass) during drying are complex,[Citation13,Citation14] poor results result in kinetics and energy consumption because of the interaction of these mechanisms. Thus, this study aims to tease out the main key points to address the critical issues of conventional drying processes.
Intensification ways for addressing the drying process issues
Effective process intensification must integrate fundamental thermodynamic and thermochemical analyses, identify the steps of the operation, and determine the various transfer phenomena at each stage. Instead of modeling the drying processes as coupled modes of heat and mass transfers, we privileged an approach based on comparing and identifying the limiting processes between successive transfers. Thus, various possibilities are foreseen, studied, and compared to choose the most appropriate way to respond and address the identified problems.
In this way, the airflow drying analysis has revealed three main stages:[Citation13,Citation15]
The first step concerns surface evaporation coupled with sweeping out toward the surrounding environment of the generated vapor. Its rate depends on the airflow speed, temperature, and humidity.
The second drying stage involves the following two procedures of surface evaporation and internal liquid water diffusion into the porous material. An interesting kinetic aspect in this step is the competition between the internal water diffusion and the superficial sweeping-out of the generated vapor. The convection heat is firstly devoted to generating the vapor and, optionally, a heat conduction transfer in the matrix:
(1)
(1)
EquationEquation (1)(1)
(1) shows that the higher the vapor production rate, the lower the wet-bulb temperature (WBT). Conversely, when the drying rate decreases, the WBT increases asymptotically up to the airflow temperature.
The third stage is the paradoxical stage of internal heat transfer (conduction) coupled with internal vapor generation and diffusion. It is worth noting that the diffusion driving force is the vapor pressure gradient. Thus, the vapor diffusion runs from the surface toward the material core.
The interaction between airflow and exchange surface occurs in stages 1 and 2. Its intensification requires decreasing the airflow humidity and increasing the exchange surface A, vapor-air transfer, and superficial water activity.
Thus, for the first stage (starting accessibility), the higher the airflow velocity, the higher the value of the mass transfer coefficient. Thus, higher airflow rates and temperatures are appropriate ways to increase the drying kinetics for this stage.
The second drying stage can have the internal diffusion of water as the limiting process. Consequently, a higher airflow rate does not necessarily increase the drying rate. Indeed, after optimization of the external conditions (airflow rate, temperature, and humidity), the drying operation becomes negligible external resistance (NER) during this stage. Then, NER characterizes the fundamental kinetics. If the kinetics of airflow drying depends on the airflow velocity, the water diffusion within the material is not the limiting process (Absence of Negligible External Resistance). Thus, Mounir Sabah et al.[Citation16] defined a critical airflow velocity (CAV) as the limit beyond which higher air velocity no longer impacts the drying rate. Hence, the internal water diffusion process controls the drying only once the airflow velocity exceeds CAV.[Citation15]
The 3rd drying phase follows the paradoxical mechanism. The fundamental analysis shows that intensification requires replacing the vapor transfer of the Fick-type mechanism with Darcy-type permeation transfer within the porous matrix. Then, microwave heating and evaporation,[Citation17] multi-flash drying (MFD),[Citation18] or superheated steam drying[Citation19] can act effectively. All these processes often ensure homogeneous and uniform heating in volume to generate vapor in the pores. Transfer by Darcy-type permeation (EquationEq. (2)(2)
(2) ) becomes possible and practical. It has the total pressure gradient as the driving force. Strong kinetics characterizes this process revealing the high drying rate encountered in microwave drying, multi-flash drying MFD, and superheated steam drying.
(2)
(2)
D.I.C. in addressing the drying process issues
Removing water in the rubbery zone before crossing the glass transition limit of water content and temperature (Wg, Tg), biological materials show significant deformation and reduced volume.[Citation20] To intensify the internal diffusion of water, it becomes necessary to remedy the shrinkage/collapse of biological materials. To address this issue, Instant Controlled Pressure Drop technology (D.I.C.) proved as a staple tool to provide a new way to expand biological matrices.
D.I.C. is a thermomechanical treatment that consists of subjecting a product to a high saturated steam pressure (generally between 100 – 1000 kPa) for a short time (some seconds), followed by an instant controlled pressure drop (ΔP/Δt > 500 kPa/s) toward the vacuum (around 5 kPa) ().
Figure 1. Typical pressure/temperature-time profile for a D.I.C. processing cycle: (1) Introduction of biological material to the reactor; (2) Establishment of an initial vacuum in the processing reactor; (3) Injection of saturated dry steam at the selected pressure and maintenance of treatment pressure during selected time; (4) Instant controlled pressure drops toward a vacuum and (5) Releasing to atmospheric pressure.[Citation21]
![Figure 1. Typical pressure/temperature-time profile for a D.I.C. processing cycle: (1) Introduction of biological material to the reactor; (2) Establishment of an initial vacuum in the processing reactor; (3) Injection of saturated dry steam at the selected pressure and maintenance of treatment pressure during selected time; (4) Instant controlled pressure drops toward a vacuum and (5) Releasing to atmospheric pressure.[Citation21]](/cms/asset/972527df-5a6b-48f9-8d20-401c4282ff3c/ldrt_a_2223278_f0001_c.jpg)
The instant pressure drop triggers the autovaporization of the water (generated as a function of the temperature difference between the initial heating stage and the final equilibrium temperature), the swelling (which produces a new porous microstructure), and the instantaneous cooling of products (which stops thermal degradation)[Citation13]
Generally, D.I.C. brings higher porosity, lesser tortuosity, and greater water diffusivity for glassy-state biological materials. Pech-Almeida et al.[Citation21] overviewed numerous food drying research work proving that remedying the shrinkage phenomenon enhanced the effective diffusivity of water. shows the impact of D.I.C. treatment on an orange peel.
Figure 2. Micrographs zoomed 40 times of untreated dried orange peel (A) and D.I.C.-treated dried orange peel (B). Adopted from Allaf et al.[Citation22]
![Figure 2. Micrographs zoomed 40 times of untreated dried orange peel (A) and D.I.C.-treated dried orange peel (B). Adopted from Allaf et al.[Citation22]](/cms/asset/20a86dbc-c088-4a95-bd86-a2cf4bf05544/ldrt_a_2223278_f0002_b.jpg)
Swell-Drying (SD) combines conventional drying operations with Instant Controlled Pressure Drop (D.I.C.) treatment.[Citation23] It typically uses conventional drying operations such as air drying, microwave drying, and infrared drying to reach a moisture level capable of ensuring the glass transition initiated by D.I.C. Then, once biological materials get the optimal moisture content, D.I.C. is carried out to generate higher expansion and porosity and lower tortuosity. The final drying step, following D.I.C. treatment, usually undertakes with a higher water diffusion and a higher kinetic drying without shrinkage, which implies a lower energy consumption.[Citation13,Citation24–26] illustrates a typical swell drying operation: the case of tomatoes (Solanum lycopersicum L.).
Figure 3. Example of a typical swell-drying operation and schematic diagram of laboratory D.I.C. Equipment (ABCAR-D.I.C. Process, Compiegne, France): (1) D.I.C. reactor; (2) vacuum tank; (3) vacuum pump; (4) Trap, V1-V7-valves, S1, and S2 saturated steam injection, W1- cooling water.
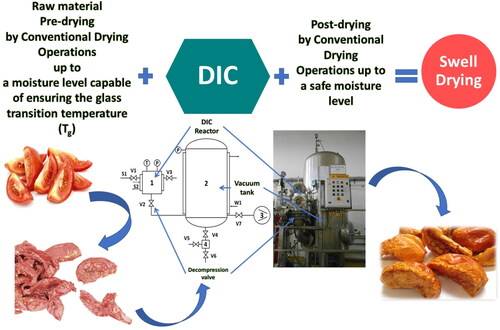
The controlled D.I.C. expansion improves the matrix porosity to reach similar, even superior freeze-dried snack material.[Citation27–30] SD increases the porosity considerably while the casehardening disappears altogether, producing an excellent crispy texture.[Citation31] Besides, it is worth noting that high snacking quality can occur even for starch-free material. Furthermore, SD expanded granule powder gets high specific functional behavior, such as high rehydration kinetics and water holding capacity (WHC). It also has low decantation and lengthy suspension time of powders.[Citation21,Citation32–35]
Moreover, SD reduces temperature for the final stage of the drying process, involves more intense evaporation, and thus an increase in preserving the nutritional attributes.[Citation21,Citation36–39]
Decisively, one of the most critical D.I.C. impacts is bacterial decontamination, leading to an extensive shelf-life of solids, grains, and powders. Indeed, by coupling Ultra High-Temperature/Short Time (UHT) treatment with a mechanical instant controlled pressure drop, D.I.C. implies greatly effective decontamination regarding bacteria, sporulates, mold, insects, and larvae by triggering an explosion of their cell walls.[Citation40–42] Indeed, the spore size is estimated between 1 to 50 µm with a moisture content of approximately 30%. EquationEquation (3)(3)
(3) shows that the pressure-drop of saturated steam pressure from 110 kPa toward a vacuum of 5 kPa results in an initial internal pressure in the volume of the spore of about 9000 kPa (90 bars):
(3)
(3)
Where:
ρapp is the apparent spore density; V is the spore volume; cp,d and cp,W are the specific heats of dry matter and water, respectively; W is the spore water content just before the pressure drops; Tt and Te are the treatment temperature and final equilibrium temperature, respectively; and Lv is the water evaporation heat. For ρapp =1150 kg m−3; W = 0.15 g H2O/g db; cpd=1050 J kg−1 K−1; cpW = 4180 J kg−1 K−1; Tt = 105 °C, and Te = 33 °C, the initial pressure just after the instant pressure-drop Pi,s would be about 79 bars, what it can explain the mechanical decontamination (by more than 4 decimal levels) of the sporulates during 5-s D.I.C. treatment.
Finally, D.I.C. coupled with conventional drying processes can address the main issues of drying processes (textural defects of collapse-shrinkage and case hardening, microbiological contamination, poor rehydrability, weak drying kinetics, and marketing difficulty). Therefore, SD inevitably plays new strategic trends in preserving agricultural production that could contribute to ensuring global human food security.
Interval Hyper-Active Drying (IHAD): from phenomenological modeling to specific novel swell-drying SD processes
We could define a new type of SD by associating D.I.C. with Interval Hyper-Active Drying (IHAD) processes. It led to lower energy consumption and minor wet-bulb temperature, which means higher SD process performance and product quality. Thus, IHAD uses various classical drying technologies modifying their used conditions; temperature and velocity of airflow drying, specific power and frequency of Infrared (IR) and microwave (MW) drying,[Citation17,Citation43,Citation44] etc. IHAD differs from intermittent operations by perfectly separating the two mentioned mechanisms. Hence, while the two simultaneous mechanisms of internal water diffusion/transfer in the porous matrix and vapor generation and sweeping out intercede at the exchange surface, IHAD drying operations attempt to ideally separate these two vital procedures.[Citation37,Citation45–47] Indeed, IHAD drying consists of transforming each conventional operation toward double independent processes of:
Great vapor generation and pronounced sweeping-out far from the product (tON)
Internal water diffusion state that aims at homogenizing water content through a similar Fick transfer, even in the case of microwave drying (tOFF). Therefore, the uniform moisture eradicates superficial and deep case hardening in convective airflow, infrared (IR), and microwave (MW) drying; it also avoids MW-burned nodal points.
Additionally, theoretical energy savings may only sometimes be achievable in practice. In this way, various experimental studies reinforced by phenomenological modeling of drying kinetics have enabled the assessment of the already three versions of IHAD (): (1) the Interval Starting Accessibility Drying (ISAD), (2) the Interval Microwave Airflow Drying (IMAD), and (3) the Interval Infrared Airflow Drying (IIRAD).
Each IHAD operation includes short, brief, hyper-concentrated, and intense moments of generation and sweeping away of vapor (tON), followed by an adequate tempering period devoted to the diffusion and homogenization of water within the matrix (tOFF). Regardless of IHAD drying operations, the stage tON of vapor generation and removal must be so intense that it must generally be very short-term with high transient efficiency. In contrast, the passive period tOFF fully respects the structure, porosity, tortuosity, and water diffusivity.
Since IHAD has a higher evaporation rate during active periods tON, it does not suffer from the disadvantages of longer drying times in intermittent drying processes.[Citation48,Citation49] Moreover, lower energy consumption made it possible to use shorter energy sources. Henceforward, by appropriately redesigning the drying apparatus, ABCAR-DIC Process has solved the lower equipment utilization rates of various IHAD types.
Academic research and engineering work enabled the meaningful joining of each IHAD version with Instant Controlled Pressure Drop (D.I.C.) processing and texturing. These coupled procedures have simulated novel interval drying systems and new D.I.C. reactors, resulting in more relevant versions of swell-drying.
Interval Starting Accessibility Drying (ISAD)
In the airflow drying of a structured body in short intervals, the active step tON involves generating vapor from the exchange surface to sweep it away into the environment. Subsequently, the drying rate during tON can be exceptionally high by adopting the highest airflow velocity and temperature. Indeed, since these steps only incriminate the exchange surface without any implication of internal water diffusion, it is possible to increase the airflow velocity without being limited by a critical CAV value. The possibility of significantly increasing the airflow temperature is also worth noting, as the high evaporation rate could involve a low wet bulb temperature value. In addition, as each tempering step tOFF preceding the active step tON should allow the internal water to sufficiently homogenize the exchange zone, the superficial water activity aw,s should have the same water activity aw of the entire material. Therefore, vapor generation at the surface should be much more efficient.[Citation45]
The central part of the operation is devoted to tOFF as water diffusion steps because of the low value of internal water diffusion. Since the wet-bulb temperature is much lower in ISAD operation than in the conventional drying process, the water diffusion should decrease; thus, the total water diffusion time must be longer. However, the short time devoted to each active period tON and the low thickness of the dried food can contribute to reducing the passive period tOFF.[Citation45]
Estimation of active tON and passive tOFF steps of ISAD
During the active period tON, the amount of vapor per cycle mv/cycle extracted from the surface depends on the boiling vapor pressure pw,T at the considered temperature, as well as the activity of the water aw. Hence, it is possible to postulate (EquationEquation (4)(4)
(4) ):
(4)
(4)
For a light value of tON (a few seconds), it is possible to assume the homogeneity of drying over a small thickness e that makes it possible to write (EquationEquation (5)(5)
(5) ):
(5)
(5)
During the tempering step tOFF, the water diffusion must recharge the sample volume (A*e). The water that diffuses in the matrix has an amount substantially close to what the active step tON has previously removed:
(6)
(6)
These assumptions make it possible to estimate tOFF and establish it vs tON:
(7)
(7)
(8)
(8)
ISAD experimental studies
In conventional drying, heat-sensitive bioproducts cannot undertake high temperatures. This high temperature would cause overheating and over-drying of the surface layer (casehardening), which results in the degradation of product quality, low operating performance, and weak equipment reliability.[Citation50,Citation51] Indeed, drying time would increase, as well as energy consumption and operating costs.[Citation45] When the airflow parameters relate to negligible external resistance (NER), internal transfer resistance controls the drying kinetics. Then, the external heat and mass rate increase would not substantially increase drying kinetics.[Citation15] Indeed, as Allaf et al.[Citation13] reported, the drying surface can reach the equilibrium moisture content; therefore, continuous external heating heats the material without improving moisture evaporation.
ISAD subjects the products to concise and repeated active periods (tON of some seconds), alternated with tempering periods (tOFF of a few minutes) usually devoted to improving the water distribution of moisture throughout the product by internal water diffusion.[Citation45] Since ISAD active period (tON) allows almost exclusively using heat to create vapor, this procedure expects to reduce thermal damage. The heat-free passive stage (tOFF) involves controlling product water diffusion producing a weak moisture gradient.[Citation52]
On the other hand, it is well known that classical hot air drying infers the loss of functionality in cell membranes, which causes significant changes in sensory, functional, and nutritional quality. It also causes less drying performance and reveals overall operating costs.[Citation53–55] In contrast, ISAD process improves the quality of dehydrated food with lower energy consumption. Hence, it can expand markets, leading to advances in food processing industries. The experiments on different food matrices such as quince, strawberries, and crab meat[Citation45,Citation56,Citation57] have shown that drying by intervals can perfectly correlate with D.I.C. It leads to higher quality swell-drying regarding nutritional, microbiological decontamination, and sensory/functional characteristics of swell-dried products.
ISAD process performance and product quality
From an energy perspective, optimal drying corresponds to energy consumption almost matching the energy required for water evaporation.[Citation58] In this respect, experimental studies of ISAD have demonstrated that the critical point to intensifying drying operation is to reach during each tempering period the water homogeneity within the material matrix by diffusion.[Citation45] In this way, the studied operational conditions of ISAD have been the airflow velocity and temperature, the active drying time (tON), and the tempering time (tOFF). In addition, it is vital to respect the Negligible External Resistance (NER) condition.
In the particular case of quince fruit,[Citation45] the selected studies parameters were airflow velocities of 2, 3.5, and 5 m s−1, airflow temperature of 40 °C, active drying time (tON) of 15 s, and tempering time (tOFF) varying from 1 to 5 min. Furthermore, results showed that by raising the tempering (passive) periods, the effective drying rate increased significantly during the active tON periods, reducing the drying time by around 86% for continuous airflow drying. On the contrary, the effective drying rate was as slow as tOFF decreased. On the other hand, the experimental results obtained with quince and strawberries[Citation45,Citation57] proved the positive effects of higher tempering (passive) periods ISAD on color and aroma preservation. The ISAD fruit slices could keep color closer to the raw material, much better than those conventionally treated. The critical role of tempering periods in maintaining a low temperature of the product core can explain these results. Due to keeping the surface wet, ISAD allows the input energy to evaporate the surface water exclusively.[Citation45] shows temperature profiles of quince fruit under ISAD and continuous drying processes.
Figure 5. Effect of the drying regime on the efficiency of the quince fruit’s wet bulb temperature under an airflow velocity of 3.5 m/s. Adopted from Hajji et al.[Citation45]
![Figure 5. Effect of the drying regime on the efficiency of the quince fruit’s wet bulb temperature under an airflow velocity of 3.5 m/s. Adopted from Hajji et al.[Citation45]](/cms/asset/9fd965c4-0b53-4ef8-9222-e3ed6e8e8078/ldrt_a_2223278_f0005_c.jpg)
In addition, with extended tempering periods, ISAD leads to a significant gain in energy. shows that using ISAD operation in crab reduced the drying time to 89% compared to continuous convective air drying.[Citation56] The operating conditions were the airflow of 3.5 m s−1 and 45 °C, active drying time (tON) of 15 s, and tempering time (tOFF) of 120 s. This reduction in drying time is related to a better redistribution of moisture and temperature, with lower moisture gradients and the highest water content on the surface.[Citation45]
Table 1. Effect of ISAD on crab meat drying time and energy gain.
On the other hand, it is worth noticing that even intermittent operations can cause thermochemical degradation of food products. Removing water from the tissues induces texture degradations such as lower material volume, shrinkage/collapse, casehardening, or other deformation.[Citation59] Besides, the combined effect of temperature and heating time may decrease food’s functional quality, such as protein aggregation and degradation.[Citation60,Citation61] In this respect, Qixing et al.[Citation62] studied the temperature effect on the protein composition of bighead carp muscle and observed that more extended heating periods affected protein solubility. On the contrary, the low-temperature changes that trigger ISAD-optimized drying () could significantly reduce such quality deterioration. Thus, comparing the conventional convective drying of crab meat to ISAD with tON = 15 s and tOFF= 1 min, much lower protein degradation on ISAD samples was observed.[Citation56] Since longer tempering (passive) periods (tOFF) allow water to adjust better throughout the product, the next tON provokes a higher evaporation rate and lower wet-bulb temperature; thus, it protects the proteins from thermal denaturation.[Citation45,Citation56] Then, ISAD offers an improved functional and nutritional quality of dried products and energy efficiency, as evidenced by reduced heat inputs, shorter efficient active periods, and lower air consumption.[Citation45,Citation56,Citation57]
Finally, through short active intervals and optimized tempering times, ISAD is a promising drying technology able to mitigate some negative impacts of conventional drying processes. However, further investigation into the insertion of D.I.C. will have to prove higher swell-drying quality to potentiate this drying operation.
Interval Infra-Red Airflow Drying (IIRAD)
Conventional infrared drying of a structured matrix guarantees specific heat transfer between the exchange-surface A and IR source. When the two source and product surfaces are so close and perfectly parallel, the IR temperature TIR (expressed in Kelvin) interacts with the exchange surface of the product at Ts, including the product temperature TWBT within the global heat rate :
(9)
(9)
The higher the vapor production rate the lower the product temperature. The main risk of IR drying is the possibility of tremendous casehardening because if the drying rate significantly decreases, the temperature of the exchange surface increases.[Citation63] Exceptional internal water transfer contributes to preventing or reducing this risk. The greater the internal water diffusion, the lower the probability of casehardening.[Citation46] Since D.I.C. texturing can imply higher porosity, it often seems an excellent way to solve such a problem.
In addition, by adopting the interval drying method, it is sufficient that the active time tON be relatively low, and the tempering time tOFF be long enough to eliminate the risk of casehardening. Therefore, IIRAD allows using a relatively high-power IR source and low tON followed by an adequately passive period tOFF to obtain the homogenization of the water content in the material. Each cycle undergoes a good distribution of internal water, leading to the same water activity aws at the exchange area as the whole product water activity aw. Thus, the evaporation rate at the exchange surface becomes much higher. The wet-bulb temperature is much lower for IIRAD process than in conventional IR operation.[Citation46]
Estimation of active tON and passive tOFF periods of IIRAD
Since the effective water diffusivity is low, the water diffusion stage requires extensive tempering periods tOFF. However, the active period tON is so concise that it involves a thin dried thickness, significantly reducing the passive period tOFF.
Therefore, the quantity of vapor mv/cycle generated at the surface during the active period tON depends on the IR source power (expressed as W/cm2), the vapor mass transfer k closely depending on the airflow (EquationEquation (9)(9)
(9) ), and the surface water activity aw,s. Usually, IIRAD cycles are defined to avoid casehardening. Both water activities at the exchange surface aw,s and within the internal body aw would be similar. In addition, since tON is very short (a few seconds), we may assume that IR energy performs surface evaporation without reasonable change in the wet-bulb temperature, EquationEquation (9)
(9)
(9) becomes:
(10)
(10)
If a complete drying over a small thickness e undergoes at the product surface A, it is possible to have:
(11)
(11)
For each cycle, the tempering (passive) period tOFF consists of diffusing water to recharge this slice volume (A e) by about the same amount as the previous active period tON has eliminated. Here too, the necessary tOFF may be estimated as correlated with the effective water diffusivity Deff, tON, IR power and water content W dry basis:
(12)
(12)
The experimental data confirmed that the decrease in water content during the IIRAD drying operation needs to be accompanied by a considerable increase in the passive time tOFF. In addition, D.I.C. treatment can significantly improve the material’s porosity, which reveals an equally higher effective water diffusivity that reduces tOFF.
IIRAD process performance and product quality
Compared to conventional assisted infrared (IR) drying,[Citation64–66] IIRAD has the advantage of greater efficiency in terms of global drying time, total energy consumption, total nutritional quality, and microstructure.[Citation46] Because conventional IR drying depends on the penetration depth of infrared radiation that normally depends on the product, this operation can result in significant superficial casehardening because of a coupled heat received by the surface with a low drying rate.[Citation67–69] Contrary, IIRAD can significantly reduce this risk by providing heat only when the product surface is fully rewetted.[Citation46]
Several factors influence the final quality of IR-dried products: the drying time, temperature, distance between the infrared emitter and samples, and the high-power/long-time torque, the latter the most impacting factor triggering thermal damage revealed by discoloration and superficial browning.[Citation70–72] Usually, a low IR power or a greater distance between the sample and the IR source should be used for a longer drying time.[Citation73] In this respect, Aktaş et al.[Citation73] reported that a short distance of 10 cm caused regional browning on the surface of dried bread during IR drying; and Polat et al.[Citation74] avoided such degradation damage by involving multi-flash IR treatment. Other researchers used an intermittent version of infrared drying while alternating two and more drying techniques.[Citation68,Citation75] Nevertheless, unlike different types of intermittent drying processes, IIRAD shows that the wet-bulb temperature of the product does not exceed 22 °C despite the high IR power. While it is above 40 °C for continuous operation, IIRAD product quality is much better than continuous-IR dried products, specifically in nutrient preservation.[Citation46]
On the other hand, the studies conducted by Onwude et al.[Citation72] on energy consumption and Ziaforoughi et al.[Citation76] on intermittent IR processes show a sharp decrease in the evaporation system compared to conventional drying. In this respect, IIRAD has systematically reduced energy consumption compared to conventional drying methods and much more at high IR power.[Citation46,Citation77] High infrared flow IIRAD had halved the energy consumption compared to continuous IR drying.[Citation46]
Finally, we conclude that IIRAD can be much more interesting than conventional airflow IR drying for heat-sensitive materials by reducing energy consumption and preserving thermolabile nutrients.
Interval Microwave Airflow Drying (IMAD)
Vega-Mercado et al.[Citation78] have pondered Microwave drying (MW) as the fourth generation of drying technologies. Indeed, it offers an excellent opportunity to reduce drying time remarkably. However, in the same path as other drying processes, it involves the two fundamental procedures of 1/evaporation and sweeping away of vapor and 2/internal liquid water transfer of diffuse into the porous material following similar Fick law, including the gradient of the water content dry basis as the driving force.
Since these two mechanisms are successive, the slowest process is the limiting process. MW drying has the specificity of producing heat directly within the material. Indeed, heat generation occurs from the interaction between the dipolar water molecules and electrical waves without requiring heat conduction.[Citation17] The MW heat immediately produces vapor that undergoes Darcy-type transfer with the total pressure gradient as the driving force from the material volume to the superficial zone (EquationEquation (13)
(13)
(13) ):
(13)
(13)
where K is the permeation coefficient,
is the internal vapor velocity,
is the dry matrix velocity, and ν is the kinematic viscosity of vapor within the air. This mass transfer immediately follows MW heat production. If Deff is weak, the water homogenization becomes the limiting transfer in the porous matrix.[Citation16]
The transport of mass vapor toward the surrounding environment usually follows the airflow sweeping away. MW drying is thus specific in that the vapor production (evaporation) is strictly related to the level of MW power of waves interacting with the dipole water molecules. Unlike other drying operations, the evaporation rate does not impact the reduction of the internal temperature. MW drying has no concept of "evaporation system temperature" known as "wet-bulb temperature". On the other hand, the nodal areas randomly distributed in the volume have such a high temperature that burned spots can appear. This aspect is worse and detrimental when there is no homogenization of internal humidity. We suggested the new IMAD version to reduce these weaknesses, decrease the random presence of high-temperature areas, and remove possible textural damage from internal casehardening.[Citation16]
IMAD consists of intervals of discontinuous controlled conditions interspersing short active drying time (tON) with tempering periods tOFF, allowing a uniform internal distribution of water by diffusion, and decreasing the product temperature. The higher the MW power, the shorter the active period tON. Furthermore, an adequate passive period (tempering time) tOFF can reduce the internal temperature and prevents the possible creation of nodal areas in the material.[Citation16] On the other hand, IMAD process is more efficient by inserting airflow transport to sweep the vapor far from the product surface.[Citation16,Citation79] D.I.C. would pre-texture the tool to increase the internal water diffusivity.
IMAD process performance and product quality
Mounir Sabah et al.[Citation16] well defined and optimized IMAD in the case of shrimps. They showed that the drying rate could significantly increase when coupled with an adequate instant controlled pressure drop D.I.C. texturing. In fact, in the case of fresh shrimp by connecting a D.I.C. treatment (0.7 MPa per 130 s) to IMAD (MW power of 24 W/g wb, tON = 30 s, tOFF = 60 s), the drying time to reach 0.05 kg H2O/kg db (dry base) was about 2 min against more than 30 min without texturing. Therefore, D.I.C. texturing made IMAD shrimp significantly more effective for snacks than simple IMAD. In addition, the higher the porosity of the D.I.C. textured samples, the greater water permeability. Hence, Mounir Sabah et al.[Citation16] established for tON = 30 s, that the higher the porosity, the greater the MW-generated vapor’s permeability. In addition, the airflow (2.5 m/s) at room temperature (23 °C) sweeps this vapor far from the surface of the shrimp to the surrounding environment.
shows the effect of D.I.C.-IMAD operative conditions on the porosity ϕ of shrimp snacks. Furthermore, with a tempering period tOFF =60 s, D.I.C.-IMAD led to a significant well-controlled expansion of shrimp snacks, increasing the internal diffusion of water and, consequently, the drying rate while avoiding the internal overheated nodes.
Figure 6. Effect of D.I.C.- IMAD operative conditions on the porosity ϕ of shrimp snacks: D.I.C. treatment parameters (vapor pressure P and time t) and MW parameters power level. D.I.C. 1 (0.4 MPa and 70 s), D.I.C. 2 (0.55 MPa and 100 s), and D.I.C. 3 (0.7 MPa and 130 s).[Citation16]
![Figure 6. Effect of D.I.C.- IMAD operative conditions on the porosity ϕ of shrimp snacks: D.I.C. treatment parameters (vapor pressure P and time t) and MW parameters power level. D.I.C. 1 (0.4 MPa and 70 s), D.I.C. 2 (0.55 MPa and 100 s), and D.I.C. 3 (0.7 MPa and 130 s).[Citation16]](/cms/asset/56e7206d-c025-4e9b-9a4b-91cd9f106aa4/ldrt_a_2223278_f0006_c.jpg)
shows the drying kinetics of IMAD and D.I.C.-IMAD shrimp snacks under different MW power densities and D.I.C. operative conditions. The instant controlled pressure-drop D.I.C., combined with the interval microwave airflow drying IMAD, achieves high and well-controlled quality with a high-performance process. In the case of D.I.C.-IMAD dried shrimps, the best reconstitution behavior required a rehydration time of 3 min. In fact, in shrimps without D.I.C. texturing, it has been highlighted that under high microwave power density, the effective rehydration diffusion increased from 0.53 to 1.15 10−9 m2/s in the case of 6 and 24 W/g wb, respectively. On the other hand, in shrimps with D.I.C. texturing and IMAD (under the same MW power density mentioned before), the effective rehydration diffusion increased considerably, from 97.83 to 1700.91 10−9 m2 s−1. Finally, combining D.I.C. with IMAD would allow a much more efficient swell-drying operation in quality control and process performance than conventional MW drying.
Figure 7. Drying kinetics of IMAD and D.I.C.-IMAD shrimp snacks: A) Effect of microwave power density level for shrimp pretreated by D.I.C. under conditions of 0.7 MPa and 130 s, B) Effect of operating parameters of D.I.C. texture pretreatment (P, t) at a microwave power density level of 24 W/g wb (wet base). D.I.C. 1 (0.4 MPa and 70 s), D.I.C. 2 (0.55 MPa and 100 s), and D.I.C. 3 (0.7 MPa and 130 s).[Citation16]
![Figure 7. Drying kinetics of IMAD and D.I.C.-IMAD shrimp snacks: A) Effect of microwave power density level for shrimp pretreated by D.I.C. under conditions of 0.7 MPa and 130 s, B) Effect of operating parameters of D.I.C. texture pretreatment (P, t) at a microwave power density level of 24 W/g wb (wet base). D.I.C. 1 (0.4 MPa and 70 s), D.I.C. 2 (0.55 MPa and 100 s), and D.I.C. 3 (0.7 MPa and 130 s).[Citation16]](/cms/asset/3ba4a323-d642-42ce-bd6f-26fbf4e8f318/ldrt_a_2223278_f0007_c.jpg)
Conclusion and perspectives
Any drying operation is the site of two mechanisms: (1) generation and transport of vapor to the surrounding environment and (2) diffusion transfer of water to standardize its concentration in the product. In many conventional cases, these two processes take place simultaneously. The transport of generated vapor can significantly increase with the airflow temperature and velocity or the specific power of IR or MW. However, water diffusion is often shallow in the porous matter, predominantly plants. Shrinkage and collapse further decline this diffusion. The impact of these coupled processes is too detrimental and harmful, implying low process kinetics and high product temperature.
Furthermore, it may result in possible thermal degradation and casehardening. This impact is only possible by significantly reducing the thickness or particle size or developing a new high-porosity structure. In this respect, the Instant Controlled Pressure-Drop D.I.C. operation led to a steady increase in porosity, expansion, and diffusion. Hence, swell drying (SD), the coupling of conventional drying operations to D.I.C., has shown through numerous studies that the drying time systematically decreased under optimized operative parameters, and the functional and sensory properties of SD products are preserved. Moreover, SD implies perfect decontamination of bacteria, mold, insects, and larvae, considerably extending shelf-life.
However, Hyper-Active Drying Intervals (IHAD) can further enhance SD. Indeed, researchers of the Research & Engineering Platform for intensifying Drying Processes (REPID) defined, designed, studied, and used at an industrial scale drying processes based on Interval Hyper-Active Drying (IHAD). It includes Interval Starting Accessibility Drying (ISAD), Interval Microwave Airflow Drying (IMAD), and Interval Infrared Airflow Drying (IIRAD). IHAD perfectly separates the two fundamental mechanisms in each drying operation. Then, coupled with D.I.C. texturing process, they all led to highly controlled drying operations improving the process performance (regarding energy consumption and drying kinetics), and the final product quality (microbiological vegetative and sporulated decontamination, good texture, preserved color, rapid rehydration…).
The fundamental studies based on conclusive art ideally separate the mechanisms involved in drying. They get synergy by inserting D.I.C. treatment as the means to remedy the preliminary and harmful issues of shrinkage and collapse, microbial contaminations, etc. The results allowed combining IHAD drying and D.I.C. texturing treatment in the cases of various types of products. The apparent improvements that D.I.C. brings to drying regardless of the operation lead to wonder, "is it coherent to use any drying operation without adding it to a D.I.C. treatment?"
Nomenclature | ||
A | = | Exchange surface (m2) |
aw | = | the water activity (/) |
aw,s | = | the superficial water activity (/) |
CAV | = | Critical Airflow Velocity (m s−1) |
cp,d | = | the specific heat of dry matter (J kg−1 K−1) |
cp,W | = | the specific heat of water (J kg−1 K−1) |
D.I.C. | = | Instant Controlled Pressure Drop |
Di | = | Inside mean pipe diameter (m) |
e | = | the small superficial thickness dried during tON (m) |
FD | = | Freeze-Drying |
fD | = | Darcy friction factor (/) |
h | = | Coefficient of convection heat transfer (W m−2 K−1) |
IHAD | = | Interval Hyper-Active Drying |
IIRAD | = | Interval Infrared Airflow Drying |
IMAD | = | Interval Microwave Airflow Drying |
ISAD | = | Interval Starting Accessibility Drying |
Lv | = | Latent heat of water vaporization (J kg−1) |
Lv | = | The water vaporization heat (J kg−1) |
md | = | Dry matrix mass (kg) |
MFD | = | Multi-Flash Drying |
mv/cycle | = | Amount of vapor per cycle (kg) |
mv/cycle | = | The evaporated water for a tON cycle (kg) |
NER | = | Negligible External Resistance |
Pi,s | = | Initial internal vapor pressure just after the instant pressure-drop (Pa) |
pw,T | = | The boiling vapor pressure (Pa) |
REPID | = | Research & Engineering Platform for intensifying Drying Processes |
SD | = | Swell-Drying |
Tair | = | Air temperature (K) |
Te | = | The final equilibrium temperature (K) |
TIR | = | Infrared IR temperature in Kelvin (K) |
tOFF | = | The tempering period of intermittent drying or interval drying (s) |
tON | = | The active period of intermittent drying or interval drying (s) |
Ts | = | Surface temperature (K) |
Ts | = | The exchange surface temperature of the product in Kelvin (K) |
Tt | = | The treatment temperature (K) |
UHT | = | Ultra-High-Temperature/Short Time |
V | = | The pore volume (m3) |
= | The dry matrix velocity, | |
= | The internal vapor velocity, (m s−1) | |
W | = | Water content dry basis (/) |
WBT | = | Wet-Bulb Temperature |
Wg, Tg | = | Glass transition water content dry basis and temperature |
WHC | = | Water Holding Capacity |
σ | = | Stefan-Boltzmann constant (5.67037442 10−8 W m−2 K−4) |
εIR | = | Emissivity (/) |
νv | = | The kinematic viscosity of vapor within the air (m2 s−1) |
ρapp | = | Apparent spore density (kg m−3) |
ρd | = | Dried matter density (kg m−3) |
ρH2O | = | Water density (kg m−3) |
= | Vapor apparent density (kg m−3) | |
= | Total pressure gradient (Pa m−1) | |
= | Rate of convection heat transfer (W) | |
= | Decreasing Rate of water content dry basis (s−1) | |
ΔP/Δt | = | Rate of instant controlled pressure drop (Pa s−1) |
= | Kinematic vapor viscosity (m2 s−1) |
References
- Xiao, M.; Yi, J.; Bi, J.; Zhao, Y.; Peng, J.; Hou, C.; Lyu, J.; Zhou, M. Modification of Cell Wall Polysaccharides during Drying Process Affects Texture Properties of Apple Chips. J. Food Qual. 2018, 2018, 1–11. DOI: 10.1155/2018/4510242.
- McKee, L. H. Microbial Contamination of Spices and Herbs: A Review. LWT Food Sci. Technol. 1995, 28, 1–11. DOI: 10.1016/S0023-6438(95)80004-2.
- Mustapha, M. K.; Ajibola, T. B.; Salako, A. F.; Ademola, S. K. Solar Drying and Organoleptic Characteristics of Two Tropical African Fish Species Using Improved Low-Cost Solar Driers. Food Sci. Nutr. 2014, 2, 244–250. DOI: 10.1002/fsn3.101.
- Mahiuddin, M.; Khan, M. I. H.; Kumar, C.; Rahman, M. M.; Karim, M. A. Shrinkage of Food Materials during Drying: Current Status and Challenges. Compr. Rev. Food Sci. Food Saf. 2018, 17, 1113–1126. DOI: 10.1111/1541-4337.12375.
- Krokida, M. K.; Karathanos, V. T.; Maroulis, Z. B. Effect of Freeze-Drying Conditions on Shrinkage and Porosity of Dehydrated Agricultural Products. J. Food Eng. 1998, 35, 369–380. DOI: 10.1016/S0260-8774(98)00031-4.
- Duan, X.; Yang, X.; Ren, G.; Pang, Y.; Liu, L.; Liu, Y. Technical Aspects in Freeze-Drying of Foods. Drying Technol. 2016, 34, 1271–1285. DOI: 10.1080/07373937.2015.1099545.
- Chitrakar, B.; Zhang, M.; Adhikari, B. Dehydrated Foods: Are They Microbiologically Safe? Crit. Rev. Food Sci. Nutr. 2019, 59, 2734–2745. DOI: 10.1080/10408398.2018.1466265.
- Mathot, A. G.; Postollec, F.; Leguerinel, I. Bacterial Spores in Spices and Dried Herbs: The Risks for Processed Food. Compr. Rev. Food Sci. Food Saf. 2021, 20, 840–862. DOI: 10.1111/1541-4337.12690.
- Trucksess, M. W.; Scott, P. M. Mycotoxins in Botanicals and Dried Fruits: A Review. Food Addit. Contam. Part A Chem. Anal. Control. Expo. Risk Assess. 2008, 25, 181–192. DOI: 10.1080/02652030701567459.
- Lim, Y. J.; Eom, S. H. The Different Contributors to Antioxidant Activity in Thermally Dried Flesh and Peel of Astringent Persimmon Fruit. Antioxidants 2022, 11, 597. DOI: 10.3390/antiox11030597.
- Oliveira-Alves, S. C.; Andrade, F.; Prazeres, I.; Silva, A. B.; Capelo, J.; Duarte, B.; Caçador, I.; Coelho, J.; Serra, A. T.; Bronze, M. R. Impact of Drying Processes on the Nutritional Composition, Volatile Profile, Phytochemical Content and Bioactivity of Salicornia Ramosissima J. Woods. Antioxidants 2021, 10, 1312. DOI: 10.3390/antiox10081312.
- Krokida, M. K.; Philippopoulos, C. Rehydration of Dehydrated Foods. Drying Technol. 2005, 23, 799–830. DOI: 10.1081/DRT-200054201.
- Allaf, T.; Allaf, K. Instant Controlled Pressure Drop (D.I.C) in Food Processing; Springer: New York, NY, 2014.
- Nguyen, D. Q.; Nguyen, T. H.; Allaf, K. Volumetric Heat Transfer Coefficient in Spray Drying of Soymilk Powder. Drying Technol. 2022, 40, 1146–1152. DOI: 10.1080/07373937.2020.1857768.
- Nguyen, T. H.; Lanoisellé, J. L.; Allaf, T.; Allaf, K. Experimental and Fundamental Critical Analysis of Diffusion Model of Airflow Drying. Drying Technol. 2016, 34, 1884–1899. DOI: 10.1080/07373937.2016.1155052.
- Mounir, S.; Amami, E.; Allaf, T.; Mujumdar, A.; Allaf, K. Instant Controlled Pressure Drop (DIC) Coupled to Intermittent Microwave/Airflow Drying to Produce Shrimp Snacks: Process Performance and Quality Attributes. Drying Technol. 2020, 38, 695–711. DOI: 10.1080/07373937.2019.1694537.
- Wray, D.; Ramaswamy, H. S. Novel Concepts in Microwave Drying of Foods. Drying Technol. 2015, 33, 769–783. DOI: 10.1080/07373937.2014.985793.
- Cong, D. T.; Haddad, M. A.; Rezzoug, Z.; Lefevre, L.; Allaf, K. Dehydration by Successive Pressure Drops for Drying Paddy Rice Treated by Instant Controlled Pressure Drop. Drying Technol. 2008, 26, 443–451. DOI: 10.1080/07373930801929300.
- Devahastin, S.; Mujumdar, A. S. Superheated Steam Drying of Foods and Biomaterials. In Modern Drying Technology; Tsotsas, E., Mujumdar, A. S., Eds.; Wiley Online Library, 2014; pp 57–84.
- Krokida, M. K.; Maroulis, Z. B. Effect of Drying Method on Shrinkage and Porosity. Drying Technol. 1997, 15, 2441–2458. DOI: 10.1080/07373939708917369.
- Pech-Almeida, J. L.; Téllez-Pérez, C.; Alonzo-Macías, M.; Teresa-Martínez, G. D.; Allaf, K.; Allaf, T.; Cardador-Martínez, A. An Overview on Food Applications of the Instant Controlled Pressure-Drop Technology, an Innovative High Pressure-Short Time Process. Molecules 2021, 26, 6519. DOI: 10.3390/molecules26216519.
- Allaf, T.; Tomao, V.; Besombes, C.; Chemat, F. Thermal and Mechanical Intensification of Essential Oil Extraction from Orange Peel via Instant Autovaporization. Chem. Eng. Process 2013, 72, 24–30. DOI: 10.1016/j.cep.2013.06.005.
- Mounir, S.; Allaf, T.; Mujumdar, A. S.; Allaf, K. Swell Drying: Coupling Instant Controlled Pressure Drop DIC to Standard Convection Drying Processes to Intensify Transfer Phenomena and Improve Quality—an Overview. Drying Technol. 2012, 30, 1508–1531. DOI: 10.1080/07373937.2012.693145.
- Mounir, S.; Téllez-Pérez, C.; Alonzo-Macías, M.; Allaf, K. Swell-Drying. In Instant Controlled Pressure Drop (D.I.C.) in Food Processing: From Fundamental to Industrial Applications; Allaf, T., Allaf, K., Eds.; Springer New York: New York, NY, 2014; pp 3–43
- Hajji, W.; Gliguem, H.; Bellagha, S.; Allaf, K. Impact of Initial Moisture Content Levels, Freezing Rate and Instant Controlled Pressure Drop Treatment (DIC) on Dehydrofreezing Process and Quality Attributes of Quince Fruits. Drying Technol. 2019, 37, 1028–1043. DOI: 10.1080/07373937.2018.1481867.
- Mounir, S. Texturing of Chicken Breast Meat as an Innovative Way to Intensify Drying: Use of a Coupled Washing/Diffusion CWD Phenomenological Model to Enhance Kinetics and Functional Properties. Drying Technol. 2015, 33, 1369–1381. DOI: 10.1080/07373937.2015.1030029.
- Téllez-Pérez, C.; Cardador-Martínez, A.; Mounir, S. M.; Montejano-Gaitán, J. G.; Sobolik, V.; Allaf, K. Effect of Instant Controlled Pressure Drop Process Coupled to Drying and Freezing on Antioxidant Activity of Green “Poblano” Pepper (Capsicum Annuum L.). FNS. 2013, 04, 321–334. DOI: 10.4236/fns.2013.43043.
- Téllez-Pérez, C.; Sabah, M. M.; Montejano-Gaitán, J. G.; Sobolik, V.; Martínez, C. A.; Allaf, K. Impact of Instant Controlled Pressure Drop Treatment on Dehydration and Rehydration Kinetics of Green Moroccan Pepper (Capsicum Annuum). Procedia Eng. 2012, 42, 978–1003. DOI: 10.1016/j.proeng.2012.07.491.
- Mounir, S.; Besombes, C.; Al-Bitar, N.; Allaf, K. Study of Instant Controlled Pressure Drop DIC Treatment in Manufacturing Snack and Expanded Granule Powder of Apple and Onion. Drying Technol. 2011, 29, 331–341. DOI: 10.1080/07373937.2010.491585.
- Téllez-Pérez, C.; Sobolik, V.; Montejano-Gaitán, J. G.; Abdulla, G.; Allaf, K. Impact of Swell-Drying Process on Water Activity and Drying Kinetics of Moroccan Pepper (Capsicum Annum). Drying Technol. 2015, 33, 131–142. DOI: 10.1080/07373937.2014.936556.
- Alonzo-Macías, M.; Montejano-Gaitán, G.; Allaf, K. Impact of Drying Processes on Strawberry (Fragaria Var. Camarosa) Texture: Identification of Crispy and Crunchy Features by Instrumental Measurement. J. Texture Stud. 2014, 45, 246–259. DOI: 10.1111/jtxs.12070.
- Mounir, S.; Allaf, T.; Berka, B.; Hassani, A.; Allaf, K. Instant Controlled Pressure Drop Technology: From a New Fundamental Approach of Instantaneous Transitory Thermodynamics to Large Industrial Applications on High Performance–High Controlled Quality Unit Operations. C. R. Chim. 2014, 17, 261–267. DOI: 10.1016/j.crci.2013.10.019.
- Mounir, S.; Allaf, K. Three-Stage Spray Drying: New Process Involving Instant Controlled Pressure Drop. Drying Technol. 2008, 26, 452–463. DOI: 10.1080/07373930801929334.
- Nguyen, D. Q.; Nguyen, T. H.; Mounir, S.; Allaf, K. Effect of Feed Concentration and Inlet Air Temperature on the Properties of Soymilk Powder Obtained by Spray Drying. Drying Technol. 2018, 36, 817–829. DOI: 10.1080/07373937.2017.1357040.
- Cardador-Martínez, A.; Pech-Almeida, J. L.; Allaf, K.; Palacios-Rojas, N.; Alonzo-Macías, M.; Téllez-Pérez, C. A Preliminary Study on the Effect of the Instant Controlled Pressure Drop Technology (DIC) on Drying and Rehydration Kinetics of Maize Kernels (Zea mays L.). Foods 2022, 11, 2151. DOI: 10.3390/foods11142151.
- González-Sánchez, T.; Delgado-Alvarado, A.; Herrera-Cabrera, B. E.; Pérez-Luna, G. Efecto del proceso de deshidratación en el color y compuestos bioactivos de jitomate (Solanum lycopersicum L.). Agro Productividad. 2016, 9(4), 33–40.
- Mounir, S.; Téllez-Pérez, C.; Sunooj, K. V.; Allaf, K. Texture and Color Characteristics of Swell-Dried Ready-to-Eat Zaghloul Date Snacks: Effect of Operative Parameters of Instant Controlled Pressure Drop Process. J. Texture Stud. 2020, 51, 276–289. DOI: 10.1111/jtxs.12468.
- Albitar, N.; Mounir, S.; Besombes, C.; Allaf, K. Improving the Drying of Onion Using the Instant Controlled Pressure Drop Technology. Drying Technol. 2011, 29, 993–1001. DOI: 10.1080/07373937.2010.507912.
- Nguyen, D. Q.; Mounir, S.; Allaf, K. Comparative Study of Methods for Producing Gum Arabic Powder and the Impact of DIC Treatment (Instant Controlled Pressure Drop) on the Properties of the Product. Drying Technol. 2019, 37, 1068–1080. DOI: 10.1080/07373937.2018.1483402.
- Setyopratomo, P.; Fatmawati, A.; Allaf, K. Texturing by Instant Controlled Pressure Drop DIC in the Production of Cassava Flour: Impact on Dehydration Kinetics, Product Physical Properties and Microbial Decontamination. Proceeding of the World Congress on Engineering and Computer Science Vol. I; WCECS, Lect. Notes Comput. Sci. 2009, San Francisco, USA.
- Debs, E. Destruction des micro-organismes par voir thermo-mécanique contrôlee dans des produits solides en morceaux ou en poudre. Application aux épices et aromates. University of La Rochelle, La Rochelle, France, 2000.
- Hazime, Z. Study of the destruction of bacterial spores by Instant Controlled Pressure-Drop (DIC). Université Bretagne Sud/Université de la Rochelle, France, 2022.
- Riadh, M. H.; Ahmad, S. A. B.; Marhaban, M. H.; Soh, A. C. Infrared Heating in Food Drying: An Overview. Drying Technol 2015, 33, 322–335. DOI: 10.1080/07373937.2014.951124.
- Gupta, M. K.; Sehgal, V. K.; Arora, S. Optimization of Drying Process Parameters for Cauliflower Drying. J. Food Sci. Technol. 2013, 50, 62–69. DOI: 10.1007/s13197-011-0231-5.
- Hajji, W.; Bellagha, S.; Allaf, K. Energy-Saving New Drying Technology: Interval Starting Accessibility Drying (ISAD) Used to Intensify Dehydrofreezing Efficiency. Drying Technol 2022, 40, 284–298. DOI: 10.1080/07373937.2020.1788072.
- Rekik, C.; Besombes, C.; Hajji, W.; Gliguem, H.; Bellagha, S.; Mujumdar, A. S.; Allaf, K. Study of Interval Infrared Airflow Drying: A Case Study of Butternut (Cucurbita Moschata). LWT Food Sci. Technol. 2021, 147, 111486. DOI: 10.1016/j.lwt.2021.111486.
- Haddad, J.; Juhel, F.; Louka, N.; Allaf, K. A Study of Dehydration of Fish Using Successive Pressure Drops (DDS) and Controlled Instantaneous Pressure Drop (DIC). Drying Technol 2004, 22, 457–478. DOI: 10.1081/DRT-120029993.
- Delele, M. A.; Weigler, F.; Mellmann, J. Advances in the Application of a Rotary Dryer for Drying of Agricultural Products: A Review. Drying Technol. 2015, 33, 541–558. DOI: 10.1080/07373937.2014.958498.
- Maldaner, V.; Coradi, P. C.; Nunes, M. T.; Müller, A.; Carneiro, L. O.; Teodoro, P. E.; Ribeiro Teodoro, L. P.; Bressiani, J.; Anschau, K. F.; Müller, E. I. Effects of Intermittent Drying on Physicochemical and Morphological Quality of Rice and Endosperm of Milled Brown Rice. LWT 2021, 152, 112334. DOI: 10.1016/j.lwt.2021.112334.
- Gulati, T.; Datta, A. K. Mechanistic Understanding of Case-Hardening and Texture Development during Drying of Food Materials. J. Food Eng. 2015, 166, 119–138. DOI: 10.1016/j.jfoodeng.2015.05.031.
- Khalloufi, S.; Almeida-Rivera, C.; Bongers, P. A Theoretical Model and Its Experimental Validation to Predic the Porosity as a Function of Shrinkage and Collapse Phenomena During Drying. Food Res Inter. 2009, 42(8), 1122–1130.
- Takougnadi, E.; Boroze, T.-E. T.; Azouma, O. Y. Development of an Intermittent Drying Process of Onion. Cogent Food Agric. 2018, 4, 1422225. DOI: 10.1080/23311932.2017.1422225.
- Motevali, A.; Minaei, S.; Khoshtagaza, M. H. Evaluation of Energy Consumption in Different Drying Methods. Energy Convers. Manag. 2011, 52, 1192–1199. DOI: 10.1016/j.enconman.2010.09.014.
- Dehghannya, J.; Hosseinlar, S.-H.; Heshmati, M. K. Multi-Stage Continuous and Intermittent Microwave Drying of Quince Fruit Coupled with Osmotic Dehydration and Low Temperature Hot Air Drying. Innov. Food Sci. Emerg. Technol. 2018, 45, 132–151. DOI: 10.1016/j.ifset.2017.10.007.
- Chryat, Y.; Romdhana, H.; Esteban-Decloux, M. Reducing Energy Requirement for Drying of Beet-Pulp: Simulation of Energy Integration between Superheated Steam and Air Drying Systems. Drying Technol. 2017, 35, 838–848. DOI: 10.1080/07373937.2016.1220952.
- Gliguem, H.; Hajji, W.; Rekik, C.; Allaf, K.; Bellagha, S. Evaluating the Performances of Interval Starting Accessibility Drying (ISAD) through Protein and Total Polyphenol Contents of Blue Crabmeat (Portunus Segnis). Processes 2021, 9, 1698. DOI: 10.3390/pr9101698.
- Hajji, W.; Bellagha, S.; Allaf, K. Interval Starting Accessibilities Drying (ISAD) as an Intensification Step in Dehydrofreezing of Strawberries. In Proceedings of the 22nd International Drying Symposium, Massachusetts, USA, 2022.
- Kowalski, S. J.; PawŁowski, A. Intermittent Drying of Initially Saturated Porous Materials. Chem. Eng. Sci. 2011, 66, 1893–1905. DOI: 10.1016/j.ces.2011.01.044.
- Brasiello, A.; Adiletta, G.; Russo, P.; Crescitelli, S.; Albanese, D.; Di Matteo, M. Mathematical Modeling of Eggplant Drying: Shrinkage Effect. J. Food Eng. 2013, 114, 99–105. DOI: 10.1016/j.jfoodeng.2012.07.031.
- Duc Pham, N.; Khan, M. I. H.; Joardder, M. U. H.; Rahman, M. M.; Mahiuddin, M.; Abesinghe, A. M. N.; Karim, M. A. Quality of Plant-Based Food Materials and Its Prediction during Intermittent Drying. Crit. Rev. Food Sci. Nutr. 2019, 59, 1197–1211. DOI: 10.1080/10408398.2017.1399103.
- Abraha, B.; Admassu, H.; Mahmud, A.; Tsighe, N.; Shui, X. W.; Fang, Y. Effect of Processing Methods on Nutritional and Physico-Chemical Composition of Fish: A Review. MOJ Food Process. Technol. 2018, 6, 376–382. DOI:10.15406/mojfpt.2018.06.00191
- Qixing, J.; Zhengran, M.; Shuoshuo, W.; Yanshun, X.; Fengyu, T.; Xueqin, X.; Peipei, Y.; Wenshui, X. Effect of Temperature on Protein Compositional Changes of Big Head Carp (Aristichthys Nobilis) Muscle and Exudates. FSTR. 2014, 20, 655–661. DOI: 10.3136/fstr.20.655.
- Wang, H-c.; Zhang, M.; Adhikari, B. Drying of Shiitake Mushroom by Combining Freeze-Drying and Mid-Infrared Radiation. Food Bioprod. Process 2015, 94, 507–517. DOI: 10.1016/j.fbp.2014.07.008.
- Praveen Kumar, D. G.; Hebbar, H. U.; Ramesh, M. N. Suitability of Thin Layer Models for Infrared–Hot Air-Drying of Onion Slices. LWT Food Sci. Technol. 2006, 39, 700–705. DOI: 10.1016/j.lwt.2005.03.021.
- Onwude, D. I.; Hashim, N.; Chen, G. Recent Advances of Novel Thermal Combined Hot Air Drying of Agricultural Crops. Trends Food Sci Technol 2016, 57, 132–145. DOI: 10.1016/j.tifs.2016.09.012.
- Wang, Y.; Zhang, M.; Mujumdar, A. S.; Chen, H. Drying and Quality Characteristics of Shredded Squid in an Infrared-Assisted Convective Dryer. Drying Technol 2014, 32, 1828–1839. DOI: 10.1080/07373937.2014.952379.
- Umesh Hebbar, H.; Rastogi, N. K. Mass Transfer during Infrared Drying of Cashew Kernel. J. Food Eng 2001, 47, 1–5. DOI: 10.1016/S0260-8774(00)00088-1.
- Pan, Z.; Shih, C.; McHugh, T. H.; Hirschberg, E. Study of Banana Dehydration Using Sequential Infrared Radiation Heating and Freeze-Drying. LWT Food Sci. Technol. 2008, 41, 1944–1951. DOI: 10.1016/j.lwt.2008.01.019.
- Vishwanathan, K. H.; Giwari, G. K.; Hebbar, H. U. Infrared Assisted Dry-Blanching and Hybrid Drying of Carrot. Food Bioprod. Process 2013, 91, 89–94. DOI: 10.1016/j.fbp.2012.11.004.
- Boateng, I. D.; Yang, X.-M. Process Optimization of Intermediate-Wave Infrared Drying: Screening by Plackett–Burman; Comparison of Box-Behnken and Central Composite Design and Evaluation: A Case Study. Ind. Crops Prod. 2021, 162, 113287. DOI: 10.1016/j.indcrop.2021.113287.
- Chua, K. J.; Chou, S. K. A Comparative Study between Intermittent Microwave and Infrared Drying of Bioproducts. Int. J. Food Sci. Technol. 2005, 40, 23–39. DOI: 10.1111/j.1365-2621.2004.00903.x.
- Onwude, D. I.; Hashim, N.; Abdan, K.; Janius, R.; Chen, G. Experimental Studies and Mathematical Simulation of Intermittent Infrared and Convective Drying of Sweet Potato (Ipomoea batatas L.). Food Bioprod. Process 2019, 114, 163–174. DOI: 10.1016/j.fbp.2018.12.006.
- Aktaş, M.; Şevik, S.; Aktekeli, B. Development of Heat Pump and Infrared-Convective Dryer and Performance Analysis for Stale Bread Drying. Energy Convers. Manag. 2016, 113, 82–94. DOI: 10.1016/j.enconman.2016.01.028.
- Polat, A.; Taskin, O.; Izli, N. Intermittent and Continuous Infrared Drying of Sweet Potatoes. Heat Mass Transfer 2022, 58, 1709–1721. DOI: 10.1007/s00231-022-03212-3.
- Sui, Y.; Yang, J.; Ye, Q.; Li, H.; Wang, H. Infrared, Convective, and Sequential Infrared and Convective Drying of Wine Grape Pomace. Drying Technol. 2014, 32, 686–694. DOI: 10.1080/07373937.2013.853670.
- Ziaforoughi, A.; Esfahani, J. A. A Salient Reduction of Energy Consumption and Drying Time in a Novel PV-Solar Collector-Assisted Intermittent Infrared Dryer. Sol. Energy 2016, 136, 428–436. DOI: 10.1016/j.solener.2016.07.025.
- Afzal, M. T. Intermittent Far Infrared Radiation Drying. American Society of Agricultural and Biological Engineers, St. Joseph, Michigan Paper number 036201, Annual Meeting 2003.
- Vega-Mercado, H.; Marcela Góngora-Nieto, M.; Barbosa-Cánovas, G. V. Advances in Dehydration of Foods. J. Food Eng. 2001, 49, 271–289. DOI: 10.1016/S0260-8774(00)00224-7.
- Azharul, K. C.-L. Microwave-Assisted Pulsed Fluidized and Spouted Bed Drying. In Intermittent and Nonstationary Drying Technologies: Principles and Applications, Azharul, K., Chung-Lim, L., Wang, Y., Mujumdar, A.S., Zhang, M., Eds.; CRC Press, 1st edition 2017, p. 24.