Abstract
The recent invasion of the quagga mussel (Dreissena bugensis) in Lake Mead, Nevada–Arizona, USA has the potential to alter biological relationships in this western reservoir. We evaluated the potential impacts by examining several measurements in the Boulder Basin of Lake Mead after the introduction of quagga mussel (2007–2008). Analysis of variance did not reveal any basin-wide changes in chlorophyll a concentrations or water clarity (Secchi disk depth). Although significantly lower chlorophyll a concentrations were found in the outer basin, this reduction was likely related to the decline of dissolved phosphorus concentrations. The abundance of cladocerans, copepods or rotifers has not changed since 2007. Overall, the results suggest that there are no statistically significant changes to many of the standard water quality indices routinely measured in the Boulder Basin of Lake Mead; however, given the complexity of biological, chemical and physical processes driving this ecosystem, the long-term impacts of quagga mussels remain uncertain. This manuscript identifies impacts known to be altered by quagga and zebra mussels in other systems and aims to help lake managers develop experimental and monitoring programs that will accurately address the impacts of quagga mussels.
Two dreissenid mussels have been introduced into North America: (1) zebra mussels (Dreissena polymorpha), whose original range stretched across Europe prior to the last glaciation event, after which they were restricted in the Black, Caspian and Azov seas (CitationStarobogatov and Andreeva 1994); and (2) quagga mussels (Dreissena bugensis), initially identified in the Bug portion of the Dnieper-Bug Estuary near Nikolaev of Ukraine (CitationAndrusov 1890). The biology and ecology of these dreissenid mussels have been studied more extensively in North America since their discovery in the Laurentian Great Lakes in the late 1980s (CitationHebert et al. 1989, CitationMay and Marsden 1992, CitationMills et al. 1993, CitationNalepa and Schloesser 1993). Because of these mussels’ high fecundity, their passively dispersed planktonic veliger larval stage and their ability to attach to submerged objects with proteinaceous, byssal threads, they rapidly spread to other major basins, such as the Illinois River, Mississippi River and Hudson River (CitationRam and McMahon 1996). Before its discovery in the Boulder Basin of Lake Mead (Nevada) on 6 January 2007, the quagga mussel was primarily restricted to the Great Lakes region and the Mississippi River near St. Louis, Missouri. The presence of quagga mussels in Lake Mead in 2007 was the first confirmed introduction of a dreissenid species in the western United States, and it was also the first time that a large ecosystem was infested by quagga mussels without previous infestation by zebra mussels (CitationLaBounty and Roefer 2007). Following their introduction to Lake Mead, quagga mussels have colonized the lower Colorado River system as well as lakes and reservoirs in Arizona, California, Colorado and Utah. Zebra and quagga mussels have become arguably the most serious nonindigenous biofouling pest introduced into North American freshwater systems (CitationLaBounty and Roefer 2007). Dreissenid mussels have ecological, recreational and economic impacts on systems they invade because they can filter large quantities of water, impact other organisms, affect water quality and clog infrastructure. Although these invasive dreissenid species may impact ecosystems in different ways, they generally increase water clarity by removing suspended particles (e.g., phytoplankton, debris, silt and microzooplankton) in the water column (CitationGriffiths 1993, CitationLeach 1993, CitationMacIsaac 1996, CitationWong et al. 2003). The increased water clarity can then affect other ecosystem components; decreased densities of microalgae result in lower uptake rates for dissolved nutrients from the water column (CitationNicholls et al. 1999), and benthic algae and plants benefit from increased water clarity (CitationHecky et al. 2004). Mussels can alter the morphological and physical properties of their habitat areas, thereby affecting the availability of resources such as competition on food and space in the food web (CitationKaratayev et al. 1997, CitationVanderploeg et al. 2002, CitationHecky et al. 2004). Overall, these invasive dreissenid mussels are efficient ecosystem engineers that can alter the ecology of a system directly or indirectly.
Lake Mead, the largest reservoir in the United States in terms of volume (36.7 × 109 m3), is a 66,000 ha, deep subtropical reservoir on the lower Colorado River, Nevada–Arizona () (CitationLaBounty and Burns 2005). The limnology and ecology of Lake Mead is complicated by 4 inflows (Colorado River, Virgin River, Muddy River and Las Vegas Wash), 4 basins (Boulder, Virgin, Temple and Gregg) and variable seasonal and annual operation patterns. Lake Mead provides favorable environmental conditions, such as warm water, high calcium concentrations, rocky substrates, suitable pH and sufficient dissolved oxygen (CitationLaBounty and Burns 2005, CitationLaBounty and Roefer 2007), for quagga mussel growth, recruitment and reproduction. Some subsurface areas of Lake Mead have been almost completely covered by quagga mussels, especially those rocky subsurface areas (Moore B, National Park Service, Dec. 2008, pers. observ.). Past studies have identified a limited benthic community dominated by chironomids, oligochaetes and Asian clams in Lake Mead (CitationMelancon 1977, CitationPeck et al. 1987). A recent benthic survey conducted in 2008 identified quagga mussels as one of the most dominant species in the soft sediment (S. Chandra, University of Nevada, Apr 2009, pers. comm.). The purpose of this study was to evaluate whether quagga mussels have had impacts on chlorophyll a (Chl-a), water clarity and zooplankton by analyzing data collected from the outer, middle and inner regions of Boulder Basin prior to and following invasion.
Materials and methods
Boulder Basin, the most downstream basin of Lake Mead, is where quagga mussels were originally identified and is thought to be the location where the introduction of the mussels occurred. It is the most intensively monitored basin due to its importance as a source of drinking water for the Las Vegas Valley, the downstream transport of water to California and Arizona, and its recreational value. Additionally, Boulder Basin receives all drainage from the Las Vegas Valley via the Las Vegas Wash that flows into Las Vegas Bay. The distance from Boulder Canyon to Hoover Dam (Black Canyon) is about 15 km, and the distance from the confluence of Las Vegas Wash to Hoover Dam is about 16 km (), with 3 descriptive regions, inner, middle and outer basins, based on their proximity to the Las Vegas Wash (CitationLaBounty and Burns 2005). The influx of nutrients from the Las Vegas Wash contributes to the higher productivity of the inner basin, while the deepest outer basin is more oligotrophic (CitationLaBounty and Burns 2005). The spatial heterogeneity of this basin can lead to significant ecological differences regarding the invasion of quagga mussels; therefore, 3 sampling stations, CR346.4 (36°03′42″ N, 114°44′21″W), LVB 7.3 (36°05′29″ N, 114°47′18″W) and LVB4.15 (36°07′01″ N, 114°49′50″W) representing the outer, middle and inner basins, respectively, were used to evaluate the potential impact of quagga mussels in Boulder Basin (). In recent years, Lake Mead's ecosystem has experienced significant natural and human-caused changes in addition to the quagga mussel invasion. For example, lake levels fluctuate yearly, and we are in the midst of a severe drought that began in 2000 (). Anthropogenic nutrient loading from Las Vegas Wash () to Las Vegas Bay and Boulder Basin by wastewater treatment plants has affected the chlorophyll concentrations in Las Vegas Bay and Boulder Basin. There is a long-term trend of decreasing total phosphorus (TP) loading from Las Vegas Wash to Las Vegas Bay, with the lowest amount recorded in 2005 (). Analysis of variance on monthly TP loading from 2000 to 2008 demonstrated that the difference from 2002 to 2008, however, was not significant (P > 0.05). From 2002 to 2008 the lake level was relatively lower due to the recent drought (). To minimize the impacts from TP loading and lake level on Chl-a concentration and other parameters, the data collected from 2002 to 2008 were used in the present investigation. Usually, the first year when invasive dreissenid mussels were found is set as the start of the post invasive period, such as zebra mussels in Lake Erie (CitationLeach 1993) and the Hudson River (CitationStrayer et al. 1999). Although the first Lake Mead-born cohort of quagga mussels might have settled in Boulder Basin of Lake Mead as early as August 2005, their exponential growth and spread started in 2007 (B. Moore, National Park Service, and W.H. Wong, University of Nevada Las Vegas, Sept. 2008, unpubl.). The impact from quagga mussels before 2007 is assumed to be negligible due to their lower abundance. As a result, pre- and post-quagga periods for the purpose of this study were set from 2002 to 2006 and 2007 to 2008, respectively. Overall, data collected from these stations from 2002 to 2008 should provide valuable insight on the potential ecological impacts of quagga mussels in Boulder Basin.
Figure 2 Lake Mead surface elevation (solid line) and total phosphorus (TP) discharge (triangle connected with dashed line) from Las Vegas Wash to Las Vegas Bay (triangle) from 1990 to 2009. Data for water surface elevation is from www.usgs.gov; data for TP loading was provided by Dr. Douglas Drury.
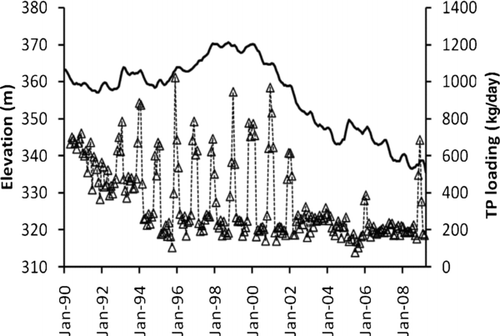
Chlorophyll a (Chl-a)
Chl-a data from the 3 sampling stations were collected weekly to monthly by Southern Nevada Water Authority (SNWA) and US Bureau of Reclamation (USBR) from 2002 to 2008. Integrated surface water samples (from surface to 5 or 6 m) were collected using a flexible hose. The sample was decanted into a sample bottle and filtered on-board using a Millipore Corporation manifold and vacuum pump apparatus. In most cases, 650 mL of water was filtered through a Whatman GF/C fiberglass filter. The filter was frozen until further processing in the laboratory could take place. Acetone was used in the extraction method for Chl-a following standard procedures established by American Public Health Association (APHA 2005). A spectrophotometer was used to measure the absorbance of Chl-a, and concentration was calculated based on Method 10200 H (2) described by APHA (2005). The average concentration of Chl-a by month was calculated and the annual means of monthly Chl-a concentrations were compared.
Water clarity
Secchi disk data collected monthly by USBR and SNWA at the 3 sampling stations from 2002 to 2008 were used to evaluate the effect of quagga mussels on water transparency. Using a standard black and white Secchi disk and a plastic or metal cable marked with centimeters and meters, readings were taken by lowering the disk until it was no longer visible. All Secchi readings were made using a 1 m-long viewscope, black inside with a tilted clear plastic base. Use of the viewscope greatly reduces the influences of wind (and resulting wave action) and variable light, thus equalizing readings from time to time (CitationLaBounty 2008). The average Secchi reading for each month was calculated and used to represent the transparency of that month; annual averages were calculated for comparison.
Zooplankton
Monthly zooplankton samples were collected from 2002 to 2008 with a Wisconsin plankton net (with 64 μm mesh) towed from 0 to 30 m of the water column. The collected samples were placed in bottles with Lugol's solution. Identification and enumeration of these samples were conducted by Dr. John Beaver, BSA Environmental Services, Beachwood, Ohio. More information on the protocol can be found at the following website: http://www.bsaenv.com/aquatics/zoops/index.html. All samples were identified to species or genus level., For presentation purposes, however they were grouped into the following major zooplankton taxa: copepods, cladocerans, rotifers and quagga mussel veligers. Abundance of each group was calculated for each month and further compared to see whether any significant difference existed among years.
Statistics
One-way analysis of variance (ANOVA) was used to compare Chl-a concentration, Secchi disk depth and zooplankton abundance to determine whether there was any significant change from 2002 to 2008. If a significant difference was found, posthoc multiple comparisons were performed with Fisher's least significant difference (LSD). A T-test was used to compare the annual and seasonal (monthly) difference before (prior to 2007) and after (2007 to 2008) quagga mussel invasion into Lake Mead (prior to the T-test, the data were first tested using equality of variances to decide whether the T-test was to be carried with equal variances or unequal variances). Simple linear regression was used to analyze the temporal trend of Chl-a concentrations, the temporal trend of orthophosphate (OP) concentrations (T Tietjen, Southern Nevada Water Authority, unpubl.) and the relationship between Secchi disk depth and Chl-a. Stepwise multiple regression was used to examine the potential factors affecting Chl-a by using annual Chl-a concentration as the dependent variable and corresponding annual OP concentration, TP loading from Las Vegas Wash, and water level as independent variables. All variables left in the model are significant at the 0.15 level. Basin-wide difference before and after quagga mussel invasion was tested by 2-way ANOVA with station (CR346.4, LVB7.3 and LVB4.15) and period (prior to 2007 [2002–2006] and after [2007–2008]) as 2 independent variables. We used SAS 9.1 (SAS Institute Inc. Cary, NC) to perform all the statistical analysis. All tests used the significance level of α= 0.05 and high significance level of α= 0.01.
Results
Chl-a
Monthly Chl-aconcentrations at the 3 sampling stations () show that at LVB4.15 and LVB7.3, no difference in any month between pre- and post quagga periods was found (T-test, P > 0.05). At CR346.4, significantly lower Chl-a concentration was found in the post-quagga period in January, May, July, August and September, respectively (T-test, P < 0.05). At CR346.4, the lowest mean annual concentrations of Chl-a occurred in 2008 and 2007 and had a significant difference from those observed in 2002 to 2005 but no difference from those in 2006 (Table 1, supplemental information). At LVB7.3, Chl-a concentrations in 2006 to 2008 were lower than those observed in 2002 and 2003 at LVB7.3. No difference was found among years from 2002 to 2008 at LVB4.15 (Table 1, supplemental information). Chl-a in the post-quagga period (2007–2008) at CR346.4 was only 57% of that during the pre-quagga period (2002–2006), and T-test shows a significant difference between these 2 periods (P < 0.05, ). However, further analysis shows that the concentration of Chl-a decreased linearly with year at CR346.4, and OP concentration at this station also decreased linearly with year (). Stepwise multiple regression between Chl-a, OP, TP loading from Las Vegas Wash and water level reveals that OP concentration was the only variable affecting Chl-a concentration at this station: 82.9% of variation of Chl-a was explained by OP (Chl-a= 0.628 + 0.395 OP, R2= 0.829, P < 0.01). Although Chl-a concentrations also declined in the post-quagga period at LVB7.3 and LVB4.15, the difference was not significant (P > 0.05; ). Two-way ANOVA shows significant differences in Chl-a among the 3 stations (LVB4.15 > LVB7.3 = CR346.4, P < 0.01), while no significant difference was found before and after the quagga mussel invasion (P > 0.05). The difference among stations is thought to be caused by nutrients discharged from the Las Vegas Wash, which led to a more eutrophic inner basin (CitationLaBounty and Burns 2005). The annual TP loading from Las Vegas Wash from 2002 to 2006 and 2007 to 2008 did not differ significantly (T-test, P = 0.97). This is one possible reason that no significant difference was found between the pre- and post-invasion periods.
Figure 3 Chl-a concentrations at the 3 sampling stations in Boulder Basin of Lake Mead from 2002 to 2008.
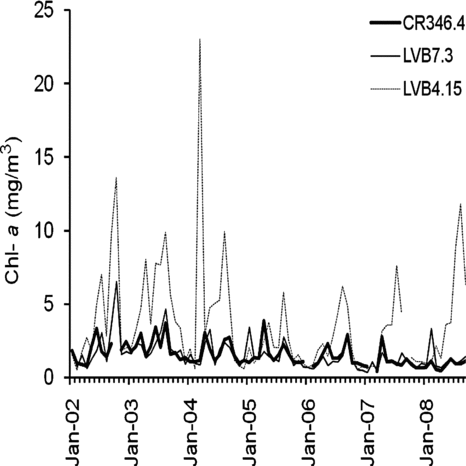
Figure 4 Chl-a concentrations within Boulder Basin before (2002–2006) and after (2007–2008) quagga mussel invasion (different letters on top of the bar in the same station show significant differences).
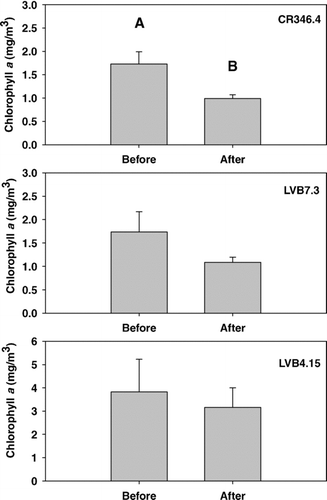
Figure 5 Temporal trend of Chl-a concentration (triangle and dashed line, Y = 364.1 − 0.181 X (R2= 0.85)) and OP concentration (dark square and solid line, Y = 832.2 − 0.414 X (R2= 0.84)) at CR346.4 of Boulder Basin. Primary Y-axis represents Chl-a, secondary Y-axis represent OP concentration and X-axis represents year.
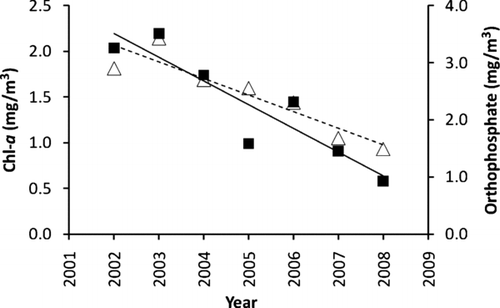
Water clarity
The monthly Secchi depths in CR346.4, LVB7.3 and LVB4.15 () show that no difference was found in any month at any of the 3 sampling stations between pre- and post quagga periods (T-test, P > 0.05). Secchi depths did not differ significantly among years in each of the 3 sampling stations (One-way ANOVA, P > 0.05), although the highest values were recorded in 2008 at CR346.4 and LVB7.3 (Table 2, supplemental information). The annual Secchi disk depth was either significantly correlated with the annual Chl-a concentration at CR346.4 (R2= 0.62, P < 0.05) or marginally related with the annual Chl-a at LVB7.3 (R2= 0.56, P = 0.05) and LVB4.15 (R2= 0.53, P = 0.06). The annual mean Secchi values for before and after quagga mussel invasion in each of the 3 stations did not show any significant difference (T-test, P > 0.05). Two-way ANOVA shows that no basin-wide impact from quagga mussels on water clarity was detected before and after the arrival of quagga mussels (P > 0.05), but LVB4.15 had lower Secchi disk depth than the other 2 stations. Our result is consistent with prior observation of an increasing trend in water clarity from the inner basin to the open water in Boulder Basin (CitationLaBounty 2008).
Zooplankton
The monthly abundance of cladocerans, copepods, rotifers and quagga mussel veligers was calculated (). For cladocerans, the monthly abundance in CR346.4, LVB7.3 and LVB4.15 did not differ before and after quagga mussel invasion (T-test, P > 0.05) except in July when abundance of cladocerans was lower in the post-quagga period (T-test, P < 0.05). For copepods, no difference was identified in any month at any station between pre- and post quagga periods (T-test, P > 0.05). No difference was found in any month from January to December in any of the 3 sampling stations between pre- and post quagga periods (T-test, P > 0.05). For rotifers, the abundance was not lower in the post-quagga period than pre-quagga period in any month of any of the 3 stations; the abundance of rotifers was even higher in September at CR346.4 or LVB7.3, or in June or September at LVB4.15 (T-test, P < 0.05). Quagga mussel veligers were first detected in March 2007 in the 3 stations. Veligers had one peak in early summer of 2007 and 2 peaks in 2008, with one in the summer and the other in the fall ( D).
Figure 7 Zooplankton abundance (#/L) at the 3 sampling stations in Boulder Basin of Lake Mead from 2002 to 2008. A: cladocerans; B: copepods; C: rotifers; and D: quaggga mussel veligers.
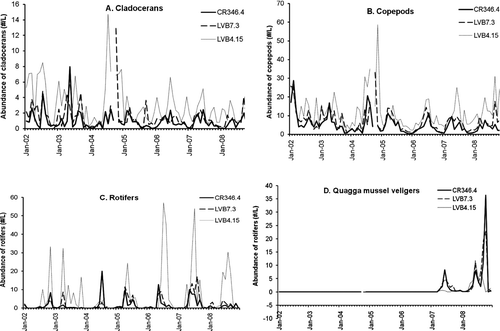
Based on the annual abundance of different groups of zooplankton (Table 3, supplemental information), copepods, cladocerans and rotifers did not show significant difference among years (one-way ANOVA, P > 0.05), and T-test did not detect any significant difference before and after quagga mussel invasion (T-test, P > 0.05). Because no veligers were found before 2007, the concentrations of veligers were significantly higher in 2007 and 2008 than in other years (ANOVA, P < 0.01). However, the post-quagga-period veliger concentrations were only marginally higher than those of the pre-quagga period (T-test, P = 0.08). For abundance of zooplankton, 2-way ANOVA shows no basin-wide difference before and after the quagga mussel invasion in cladocerans, copepods and rotifers (P > 0.05), but the difference among the 3 stations for each group was significant (LVB4.15 > LVB7.3 = CR346.4, P < 0.01). Two-way ANOVA also shows the abundance of veligers of post-quagga mussel period was significantly higher than before the 2007 period (P < 0.01), but no difference was found among stations (P > 0.05).
Discussion
In Boulder Basin of Lake Mead, no basin-wide impact on Chl-a concentrations by quagga mussels was detected. Although Chl-adecreased significantly in the open waters of Boulder Basin (CR346.4) following the arrival of quagga mussels, the reduction was likely caused by a concurrent decline in dissolved phosphorus because the biological production in Lake Mead is primarily limited by phosphorus (CitationLaBounty 2008 and references therein). It is impossible to quantify the degree to which quagga mussels can impact Chl-a or water clarity because we have neither sufficient records of quagga mussel abundance nor do we know how efficiently these mussels can filter water in this system. Nevertheless, it is useful to make some calculations to estimate the maximum possible effects. Based on the maximum recorded density of quagga mussels and shell length frequency for different substrates (e.g., silt [184 individuals/m3] vs. rock [2505 individuals/m3]) in 2007 (W.H. Wong, University of Nevada Las Vegas, and B. Moore, National Park Service, Sept 2008, unpubl.), and assuming that (1) the filtration rates with different sizes are similar to those recorded in Lake Erie (CitationZhang et al. 2008 and references therein) and (2) mussels are actively feeding 100% of the time, we estimated that it takes these mussels 169 days to filter the water of the entire Boulder Basin. Based on the monthly record of quagga mussel veligers in the Boulder Basin and assuming that their filtration rates are similar to those recorded for zebra mussels in the western Lake Erie (CitationMacIsaac et al. 1992), we estimated that it takes these veligers 264 days to filter the entire basin. By combining the filtration rates of veligers, juveniles and adults, it takes at least 103 days for these mussels to filter the entire basin. The density of Dreissena on Hen Island Reef west of the Pelee Island in western Lake Erie was about 25,000 individuals/m3 in October of 1990 and 1991, and the filtration rate of these mussels was capable of filtering a 7 m water column between 3.5 and 18.8 time per day (CitationMacIsaac et al. 1992). Compared to other systems such as western Lake Erie and the Hudson River where it only took dreissenid mussels 1–3 days to filter the entire water body (CitationBunt et al. 1993, CitationRoditi et al. 1996), the filtration capacity of these invasive quagga mussels in Boulder Basin of Lake Mead is very low. Lower filtration capacity is likely the main reason we have not seen any significant basin-wide change in chlorophyll; or if there is any change in Chl-a at a specific site such as CR346.4, the impact from quagga mussels must be very limited as the key driver of biological production is phosphorus (). Another potential factor that could constrain the impact of quagga mussels on chlorophyll is the “benthic-pelagic” boundary layer above the mussel bed (CitationAckerman et al. 2001, CitationZhang et al. 2008). Because Lake Mead is a deep reservoir, quagga mussels living at the bottom of the lake may indeed be subject to the boundary layer effect. However, the boundary layer effect in Lake Mead should not be as significant as it is in Lake Erie or other systems where the lake bottom is a large portion of the subsurface area. Lake Mead formed when impounded Colorado River water filled deep canyons, and, furthermore, there are several “islands” located in the middle of Boulder Basin such as Sentinel Island and Boulder Island. Hence, a large portion of the basin's rocky subsurface area is vertical from the bottom to the water surface.
Top-down control on primary production by dreissenid mussels is mainly due to their efficient filtering behavior. Since zebra mussels were first discovered in Lake Erie in 1988, Chl-a concentration decreased by 43% in the western basin and 27% in the west-central basin between 1988 and 1989 (CitationLeach 1993). A 20-year (1983–2002) dataset shows a downward trend of −0.07μg/L/yr in the central basin of Lake Erie, but no trend was found before the arrival of zebra mussel (CitationRockwell et al. 2005). In the Hudson River, New York, zebra mussels were found to reduce chlorophyll concentrations by 90% of the pre-zebra mussel level (CitationCaraco et al. 2006). In some areas, such as in Green Bay of Lake Michigan, Chl-a decreased immediately following dreissenid invasion but increased afterward (CitationQualls et al. 2007, CitationDe Stasio et al. 2008). A recent model of Lake Erie suggests that zebra mussels decreased algal biomass when they first invaded and their population was small (6000 individuals/m2). However, when the population grew larger (120,000 individuals/m2), dreissenid mussels increased nondiatom inedible algae by excreting a large amount of ammonia and phosphate (CitationZhang et al. 2008). The density of quagga mussels collected from 138 locations in Lake Mead in 2007 was 505 ± 667 mussel/m2 (B. Moore, National Park Service, and W.H. Wong, University of Nevada Las Vegas, unpubl.), which was lower compared to other ecosystems such as western Lake Erie (CitationMacIsaac et al. 1992). Therefore, the top-down control function from these invasive mussels in the deep Lake Mead may not be as significant as those found in the relatively shallow western Lake Erie.
At each of the 3 sampling stations, variations of Chl-a concentrations explained more than 50% of the variation of Secchi depth readings, which is similar to the results obtained by CitationLaBounty and Burns (2005). The key reason why there is no detectable impact from quagga mussels on water clarity is there has been no significant basin-wide reduction on Chl-a following the arrival of quagga mussels. Apart from Chl-a, water clarity decreased with dropping surface elevation in Boulder Basin (CitationLaBounty and Burns 2005) due to increased exposure of lake bottom, resulting in resuspension of fine former lake-bottom sediment. Water surface elevation needs to be considered when evaluating Secchi depth in Boulder Basin. The water surface elevation before quagga mussel invasion was about 9 m higher than in the post-quagga period (). The immediate effect of dreissenid mussels on water clarity is obvious in a short time for shallow lakes and basins, such as the western basin and west-central basin of Lake Erie; between 1988 and 1989, the Secchi disk transparencies increased by 1.24 m (85%) and 1.75 m (52%), respectively (CitationLeach 1993). During the first 4–5 years of dreissenid invasion, the deeper eastern basin of Lake Erie did not demonstrate any immediate improvement in water clarity (CitationMakarewicz et al. 1999) until more quaggas established in this basin for almost 10 years (CitationBarbiero and Tuchman 2004). Because Lake Mead is a deep and stratified reservoir with relatively low densities of quagga mussels in 2007 and 2008, a significant increase in water clarity was not observed following quagga mussel introduction. Similarly, water clarity enhancement after dreissenid invasion was not observed in several systems, such as the western basin of Lake Erie (CitationBarbiero and Tuchman 2004), part of Green Bay of Lake Michigan (CitationQualls et al. 2007) and the Hudson River (CitationStrayer et al. 1999). This is partially due to the large inputs of suspended solids into these lake systems and the Hudson River. For instance, spring water clarity in Lake Erie's western and west-central basins has declined significantly in the post-dreissenid period, although chlorophyll did decline by about 50% during both spring and summer following Dreissena invasion (CitationBarbiero and Tuchman 2004). This discrepancy in the responses of water clarity and chlorophyll is probably a consequence of both the large sediment loads entering the western basin and resuspension of unassimilated nonalgal particulates. But it is not clear whether the impact from sediment loading and resuspension is an issue in the Boulder Basin of Lake Mead.
No major group of zooplankton showed any significant change with the exception of the presence of the planktonic quagga mussel veliger. The higher abundance of zooplankton in LVB4.15 should be associated with the higher chlorophyll concentration in the inner basin. It has been speculated that zooplankton food resources were depleted by the filtering activity of dreissenid mussels when they established in the Great Lakes (CitationMills et al. 1993). Also, like zebra mussels (CitationMacIsaac et al. 1995, CitationStrayer et al. 1996, Jack and Thorp 2000, CitationWong et al. 2003), quagga mussels can consume zooplankton directly, especially microzooplankton such as rotifers. Field sampling and in situ enclosure experiments have shown that dreissenid mussels can impart a strong top-down control on zooplankton by direct grazing on zooplankton or through indirect competition for food with zooplankton. In the western basin of Lake Erie, rotifer abundance declined by 74% between 1988 and the 1989–1993 period, which occurred coincident with the establishment of dreissenid mussels (CitationMacIsaac et al. 1995). But cladoceran density did not change, although copepod and copepod nauplii declined by 39–69% between 1988 and 1993. In the Hudson River, microzooplankton such as rotifers, tintinnids and copepod nauplii dropped to 10–20% of their pre-invasion levels (CitationPace et al. 1998). Based on the present investigation, the major food resources (phytoplankton and chlorophyll) of zooplankton have not yet experienced significant depletion and, therefore, their populations are still healthy in Boulder Basin.
Overall, there has been no significant basin-wide impact on Chl-a, water clarity or zooplankton abundance in Boulder Basin due to quagga mussels 2 years after they were found in Lake Mead. At 8 stations in Boulder Basin, it seems there is an increase in veliger concentrations (#/L), but that the values do not differ statistically from April 2007 (0.4), April 2008 (2.0) to April 2009 (3.8; C. Holdren, US Bureau of Reclamation, unpubl. data). Quagga and zebra mussel veligers prefer hard substrates such as rocks on which to settle and grow. Lake Mead was created by impounding the Colorado River to fill canyons; 49% of its subsurface area is hard substrate, which is much higher than other systems such as the Hudson River that are composed of only 7% hard substrate (CitationStrayer et al. 1996). Populations of these invasive mussels will likely continue to grow because of the large proportion of hard subsurface area, an almost unlimited calcium supply from the Colorado River and a year-round favorable water temperature. Accordingly, if the invasive quagga mussel population continues to thrive, the potential ecological consequences should not be overlooked. The loss of chlorophyll peaks and the deepest Secchi depths recorded in the open water of Boulder Basin in recent years may be a warning of changes that may still come (CitationLaBounty 2008; T. Tietjen, Southern Nevada Water Authority, pers. observ.). Although it has been reported that quagga mussels can maintain positive growth at very low Chl-a concentration (0.05 mg/m3; CitationBaldwin et al. 2002), food could be a likely potential limiting factor for quagga mussels because Lake Mead is a less productive system, especially in the outer portion of Boulder Basin where Chl-a is <1 mg/m3 (). If the mussel population reaches its carrying capacity in the near future, there may not be any detectable impact from quagga mussels in this system; or if there is any impact, it may not be as significant as in other systems such as Lake Erie or the Hudson River. To gain a better understanding of the consequences and potential consequences of quagga mussels in Lake Mead, a long-term standardized monitoring plan, Interagency Monitoring Action Plan (I-MAP) for Quagga Mussels in Lake Mead, has been developed to track mussel size, abundance and distribution at more than 50 sampling sites throughout Lake Mead (CitationWong and Gerstenberger 2008). The plan was implemented by lake managers and participating agencies in late summer 2009. Strategic monitoring will help lake managers better understand and accurately quantify quagga mussel impact in this large reservoir in the Western United States.
Summary
Two years after the arrival of quagga mussels at Boulder Basin of Lake Mead, there has been no basin-wide significant impact on Chl-a or water clarity to date. Major zooplankton groups have not shown adverse responses to quagga mussel invasion. This investigation suggested little ecological impact of quagga mussels following the first 2 years of colonization in the Boulder Basin of Lake Mead. This is likely due to the low density of quagga mussels in the Boulder Basin of Lake Mead in the early stage of their introduction. Given the well established ecological consequences of quagga mussels on other systems, and coupled with lower chlorophyll and higher water clarity in the open water of Boulder Basin in recent years, Lake Mead needs to be monitored strategically to mitigate any potential adverse impacts in the future. Scientifically based mathematical models will also be helpful for better estimating quagga mussel populations and their impacts on this ecosystem.
Supplemental Tables
Download ()Acknowledgments
We thank Dr. Douglas Drury for nutrient loading data from Las Vegas Wash. Assistance from Mark Sappington, Dr. Jennell M. Miller, Dr. Craig J. Palmer and Warren Turkett are appreciated. We also thank Dr. Chad Cross and Dr. Sheniz Moonie for their support in statistical analysis. Review by Alan Sims, Warren Turkett, Scott Schiefer and Lawrence Bazel improved the quality of this manuscript. This study was carried out through a Great Basin Cooperative Ecosystem Studies Unit agreement between the National Park Service and the University of Nevada, Las Vegas. It was supported by Southern Nevada Public Land Management Act funding awarded to the National Park Service, Lake Mead National Recreation Area. The constructive comments from 3 anonymous reviewers were helpful in improving the quality of this manuscript.
References
- [APHA] American Public Heath Association . 2005 . Standard Methods for the Examination of Water and Wastewater. , 21st ed. , Washington , DC : American Public Health Association .
- Ackerman , J D , Loewen , M R and Hamblin , P F . 2001 . Benthic-Pelagic coupling over a zebra mussel reef in western Lake Erie . Limnol Oceanogr. , 46 : 892 – 904 .
- Andrusov , N I . 1890 . Dreissena rostriformis Desh in the Bug River . Vestnik Estestvoznaniya. , 6 : 261 – 262 .
- Baldwin , B S , Mayer , M S , Dayton , J , Pau , N , Mendilla , J , Sullivan , M , Moore , A , Ma , A and Mills , E L . 2002 . Comparative growth and feeding in zebra and quagga mussels (Dreissena polymorpha and Dreissena bugensis): implications for North American lakes . Can J Fish Aquat Sci. , 59 : 680 – 694 .
- Barbiero , R P and Tuchman , M L . 2004 . Long-term dreissenid impacts on water clarity in Lake Erie . J Great Lakes Res. , 30 : 557 – 565 .
- Bunt , C M , MacIsaac , H J and Sprules , W G . 1993 . Pumping rates and projected filtering impacts of juvenile zebra mussels (Dreissena polymorpha) in Western Lake Erie . Can J Fish Aquat Sci. , 50 : 1017 – 1022 .
- Caraco , N F , Cole , J J and Strayer , D L . 2006 . Top down control from the bottom: Regulation of eutrophication in a large river by benthic grazing . Limnol Oceanogr. , 51 : 664 – 670 .
- De Stasio , B T , Schrimpf , M B , Beranek , A E and Daniels , W C . 2008 . Increased chlorophyll a, phytoplankton abundance, and cyanobacteria occurence following invasion of Green Bay, Lake Michigan by dreissenid mussels . Aquatic Invasions. , 3 : 21 – 27 .
- Griffiths , R W . 1993 . “ Effects of zebra mussels (Dreissena polymorpha) on the benthic fauna of Lake St. Clair. Lewis Publishers ” . In Zebra mussels: Biology, Impacts, and Control , Edited by: Nalepa , T F and Schloesser , D W . 439 – 451 . Boca Raton , FL : Lewis Publishers .
- Hebert , P D , Muncaster , B W and Mackie , G L . 1989 . Ecological and genetic studies on Dreissena polymorpha (Pallas) - a new mollusk in the Great Lakes . Can J Fish Aquat Sci. , 46 : 1587 – 1591 .
- Hecky , R E , Smith , R E , Barton , D R , Guildford , S J , Taylor , W D , Charlton , M N and Howell , T . 2004 . The nearshore phosphorus shunt: a consequence of ecosystem engineering by dreissenids in the Laurentian Great Lakes . Can J Fish Aquat Sci. , 61 : 1285 – 1293 .
- Jack , J D and Thorp , J H . 2000 . Effects of the benethic suspension feeder Dreissena polymorpha on zooplankton in a large river . Freshwat Biol. , 44 : 569 – 579 .
- Karatayev , A Y , Burlakova , L E and Padilla , D K . 1997 . The effects of Dreissena polymorpha (Pallas) invasion on aquatic communities in eastern Europe . J Shellfish Res. , 16 : 187 – 203 .
- LaBounty , J. F. 2008 . Secchi transparency of Boulder Basin, Lake Mead, Arizona-Nevada: 1990–2007 . Lake Reserv Manage , 24 : 207 – 218 .
- LaBounty , J F and Burns , N M . 2005 . Characterization of boulder basin, Lake Mead, Nevada Arizona, USA - Based on analysis of 34 limnological parameters . Lake Reserv Manage. , 21 : 277 – 307 .
- LaBounty , J F and Roefer , P . 2007 . Quagga mussels invade Lake Mead . LakeLine. , 27 : 17 – 22 .
- Leach , J H . 1993 . “ Impacts of zebra mussel (Dreissena polymorpha) on water quality and fish spawning reefs in Western Lake Erie. Lewis Publishers ” . In Zebra mussels: Biology, Impacts, and Control , Edited by: Nalepa , T F and Schloesser , D W . 381 – 397 . Boca Raton , FL : Lewis Publishers .
- MacIsaac , H J . 1996 . Potential abiotic and biotic impacts of zebra mussels on the inland waters of North America . Am Zool. , 36 : 287 – 299 .
- MacIsaac , H J , Lonnee , C J and Leach , J H . 1995 . Suppression of microzooplankton by zebra mussels: Importance of mussel size . Freshw Biol. , 34 : 379 – 387 .
- MacIsaac , H J , Sprules , W G , Johannsson , O E and Leach , J H . 1992 . Filtering impacts of larval and sessile zebra mussels in western Lake Erie . Oecologia. , 92 : 30 – 39 .
- Makarewicz , J C , Lewis , T W and Bertram , P . 1999 . Phytoplankton composition and biomass in the offshore waters of Lake Erie: Pre- and post-Dreissena introduction (1983-1993) . J Great Lakes Res. , 25 : 135 – 148 .
- May , B and Marsden , J E . 1992 . Genetic identification and implications of another invasive species of dreissenid mussel in the Great Lakes . Can J Fish Aquat Sci. , 49 : 1501 – 1506 .
- Melancon , S S . 1977 . “ A preliminary survey of the macrobenthos of Las Vegas Bay, Lake Mead, Nevada. MS thesis ” . Las Vegas , NV : University of Nevada Las Vegas . Department of Biological Sciences
- Mills , E L , Dermott , R M , Roseman , E F , Dustin , D , Mellina , E , Conn , D B and Spidle , A P . 1993 . Colonization, ecology, and population structure of the quagga mussel (Bivalvia, Dreissenidae) in the lower Great Lakes . Can J Fish Aquat Sci. , 50 : 2305 – 2314 .
- Nalepa , T F and Schloesser , D W . 1993 . Zebra mussels: Biology, Impacts, and Control , Boca Raton , FL : Lewis Publishers .
- Nicholls , K H , Standke , S J and Hopkins , G J . 1999 . “ Effects of dreissenid mussels on nitrogen and phosphorus in north shore waters of Lake Erie. Backhuys Publishers ” . In State of Lake Erie (SOLE)-Past, Present, and Future , Edited by: Munawar , M , Edsall , T and Munawar , I F . 323 – 336 . Leiden , , Netherlands : Backhuys Publishers .
- Pace , M L , Findlay , S E and Fischer , D . 1998 . Effects of an invasive bivalve on the zooplankton community of the Hudson River . Freshw Biol. , 39 : 103 – 116 .
- Peck , S K , Pratt , W , Pollard , J , Paulson , L J and Baepler , D H . 1987 . Benthic invertebrates and crayfish of Lake Mead , Las Vegas , NV : Lake Mead Limnological Research Center, University of Nevada .
- Qualls , T M , Dolan , D M , Reed , T , Zorn , M E and Kennedy , J . 2007 . Analysis of the impacts of the zebra mussel, Dreissena polymorpha, on nutrients, water clarity, and the chlorophyll-phosphorus relationship in lower Green Bay . J Great Lakes Res. , 33 : 617 – 626 .
- Ram , J L and McMahon , R F . 1996 . Introduction: The biology, ecology, and physiology of zebra mussels . Am Zool. , 36 : 239 – 243 .
- Rockwell , D C , Warren , J G , Bertram , P E , Salisbury , D K and Burns , N M . 2005 . The US EPA Lake Erie indicators monitoring program 1983–2002: Trends in phosphorus, silica, and chlorophyll a in the central basin . J Great Lakes Res. , 31 : 23 – 34 .
- Roditi , H A , Caraco , N F , Cole , J J and Strayer , D L . 1996 . Filtration of Hudson River water by the zebra mussel (Dreissena polymorpha) . Estuaries. , 19 : 824 – 832 .
- Starobogatov , Y I and Andreeva , S I . 1994 . “ Distribution and history ” . In Freshwater Zebra Mussel Dreissena polymorpha (Pall.) (Bivalvia, Dreissenidae): Systematics, Ecology, Practical Meaning , Edited by: Starobogatov , Y I . 47 – 55 . Moscow , , Russia : Nauka Press .
- Strayer , D L , Caraco , N F , Cole , J J , Stuart , F and Pace , M L . 1999 . Transformation of freshwater ecosystem by bivalves: A case study of zebra mussels in the Hudson river . Bioscience. , 49 : 19 – 27 .
- Strayer , D L , Powell , J , Ambrose , P , Smith , L C and Pace , M L . 1996 . Arrival, spread, and early dynamics of a zebra mussel (Dreissena polymorpha) population in the Hudson River estuary . Can J Fish Aquat Sci. , 53 : 1143 – 1149 .
- Vanderploeg , H A , Nalepa , T F , Jude , D J , Mills , E L , Holeck , K T , Liebig , J R , Grigorovich , I A and Ojaveer , H . 2002 . Dispersal and emerging ecological impacts of Ponto-Caspian species in the Laurentian Great Lakes . Can J Fish Aquat Sci. , 59 : 1209 – 1228 .
- Wong , W H and Gerstenberger , S . 2008 . A standardized design for quagga mussel monitoring in Lake Mead, Nevada Arizona , Scottsdale , AZ : Colorado River Science and Resource Management Symposium .
- Wong , W H , Levinton , J S , Twining , B S and Fisher , N . 2003 . Assimilation of micro- and mesozooplankton by zebra mussels: A demonstration of the food web link between zooplankton and benthic suspension feeders . Limnol Oceanogr. , 48 : 308 – 312 .
- Zhang , H Y , Culver , A and Boegman , L . 2008 . A two-dimensional ecological model of Lake Erie: Application to estimate dreissenid impacts on large lake plankton populations . Ecol Model. , 214 : 219 – 241 .