ABSTRACT
Glaucoma is a leading cause of blindness worldwide, with a marked increase in prevalence with advancing age. Due to the multifactorial nature of glaucoma pathogenesis, dissecting how ageing impacts upon glaucoma risk requires analysis and synthesis of evidence from a vast literature. While there is a wealth of human clinical studies examining glaucoma pathogenesis and why older patients have increased risk, many aspects of the disease such as adaptations of retinal ganglion cells to stress, autophagy and the role of glial cells in glaucoma, require the use of animal models to study the complex cellular processes and interactions. Additionally, the accelerated nature of ageing in rodents facilitates the longitudinal study of changes that would not be feasible in human clinical studies. This review article examines evidence derived predominantly from rodent models on how the ageing process impacts upon various aspects of glaucoma pathology from the retinal ganglion cells themselves, to supporting cells and tissues such as glial cells, connective tissue and vasculature, in addition to oxidative stress and autophagy. An improved understanding of how ageing modifies these factors may lead to the development of different therapeutic strategies that target specific risk factors or processes involved in glaucoma.
Introduction
Glaucoma is one of the leading causes of visual impairment and blindness, with a global prevalence of 3.54%, projected to increase from 64.3 million in 2013 to 111.8 million individuals affected by 2040.Citation1 The risk of developing glaucoma rises markedly with higher intraocular pressure (IOP) and advancing age.Citation2 Other risk factors include genetics, ethnicity, central corneal thickness and myopia.Citation2 Although how such factors contribute to increased risk remains incomplete, using pre-clinical models, significant advances have been made in understanding the mechanisms underlying retinal ganglion cell (RGC) injury and how ageing influences glaucoma.
It is now understood that neurons and their supporting cells sense and respond to stressors (e.g., metabolic, oxidative, immune and biomechanical) via both acute and sustained cellular changes. Given that older age is a strong risk factor for glaucoma,Citation2 it may be reasonable to suggest that older eyes may have poorer adaptations and a reduced capacity to recover from stress.
Using pre-clinical evidence, this review examines retinal changes observed in both normal ageing and eyes that encounter glaucomatous stress, in particular elevated IOP. How ageing changes RGC susceptibility to injury and recovery from IOP elevation is also explored.
Retinal changes in normal ageing
The onset of age-related changes in the retina and optic nerve often coincides with the increased incidence of glaucoma. Older adults experience a decline in subjective measures of visual function, including reduced visual acuity, dark adaptation and contrast sensitivity.Citation3 Using objective approaches, such as retinal electrophysiology, reduced amplitudes and delayed timing of all cell classes including photoreceptors, bipolar cells and RGCs have been recorded in older adults.Citation4,Citation5 Accompanying these functional changes, structural thinning of the retinal nerve fibre layer, ganglion cell layer, inner plexiform layer, and photoreceptor outer segment layer are seen in older eyes ().Citation6
Figure 1. Schematic of normal ageing changes, including biomechanical, vascular and neuronal changes. Understanding how such changes increase the risk of retinal ganglion cell injury may point to novel treatment approaches. RGC, retinal ganglion cell; RNFL, retinal nerve fibre layer; LC, lamina cribrosa.
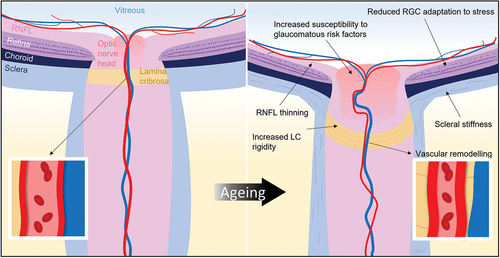
To differentiate normal ageing from disease processes, well-controlled experimental models have provided important insights. Rodent models have become widely used as they are relatively inexpensive and more accessible.Citation7 Adult (3-month-old) and old (2-year-old) mice approximate 20 and 70 years of human life, respectively.Citation8 Due to their life span of approximately 2 years, despite differences between human and rodent eyes (such as rod-dominant retina, glial lamina at the optic nerve head, and lack of macula in rodents), both inducible and genetic rodent glaucoma models are useful to study processes involved in RGC death and can be readily overlaid on ageing to further understanding of complex risk interactions.
Older mice have shown reduced visual acuity, evident by reduced optokinetic responses in 21- to 24-month-old compared to 2- to 3-month-old mice.Citation9 Like humans, older mice also showed reduced photoreceptor (a-wave amplitude) and bipolar cell driven responses (b-wave amplitude) of the electroretinogram, a measure commonly used to quantify rodent retinal function.Citation9 Compared to 2-month-old mice, reductions in photoreceptor and bipolar cell function are evident even by 6 months of age, with further decline at 12 months.Citation10 Interestingly, this functional attenuation was not associated with decline in photoreceptor nuclei,Citation10 even in 24- to 28-month-old mice.Citation11 Similarly, there was a decline in bipolar cell function between 2- and 6-month-old rats, with evidence of further decline (cone b-wave) at 22 months.Citation12
Thinning of total retinal, inner and outer nuclear layer thicknesses continued with ageing in rats between 6 and 22 months.Citation12 It is worth noting that the growth in eye size, particularly increased retinal area between 2 and 6 months of age, may lead to apparent retinal thinning though the total tissue volume is conserved in rodents.Citation11,Citation12 Cell count estimates in the inner and outer nuclear layers did not show any significant differences in rodents between 6 and 22 months of age, suggesting that cells were spread over a larger retinal area.Citation11,Citation12 That age-related functional decline was not explained by cell loss suggests that other cellular processes may contribute to age-related functional attenuation.Citation12 These studies highlight the importance of considering the choice of age ranges for ‘adult’ and ‘old’ animals when seeking to understand age-related changes.
Whilst gross cell numbers appear not to change with age, functional decline may involve reduction in synaptic density, shrinkage of RGC dendritic field area in the inner plexiform layer and reduced amacrine cell density as reported in 24- to 28-month-old compared to 3- to 5-month-old mice.Citation11 Single cell recordings did not show any difference in response from directionally sensitive RGCs between these ages.Citation11 Consistent with this, RGC function as measured using the electroretinogram scotopic threshold response was also relatively preserved in 18-month-old compared to 3-month-old rats.Citation13
The preservation of RGC function with age may reflect inner retinal adaptations to maintain retinal output. RGCs in 12-month-old mice, particularly the ON RGCs, became relatively more complex than those in 3-month-old mice.Citation14 Why ON RGCs might show more prominent age-related changes is unclear, but their higher energy demand compared with OFF RGCs may be a contributor.Citation15 It may also be possible that with normal ageing, RGCs remodel to become smaller but more complex to modify contact with bipolar and amacrine cells. These adaptations allow for RGCs to maintain visual output despite an age-related decline in outer retinal function ().
Figure 2. Retinal ganglion cell (RGC) and glial adaptations with ageing and intraocular pressure (IOP). With age, RGCs show reduced complexity along with evidence of an attenuated capacity for IOP induced morphological changes. Microglia, astrocytes and perhaps Müller cells in older eyes take on a more proinflammatory state and appear to show a slower but more exaggerated response to IOP elevation. RNFL, retinal nerve fibre layer; GCL, ganglion cell layer; IPL, inner plexiform layer; INL, inner nuclear layer; OPL, outer plexiform layer; ONL, outer nuclear layer; IS, photoreceptor inner segment layer; OS, photoreceptor outer segment layer; RPE, retinal pigment epithelium; Br, Bruch’s membrane; Sc, sclera; ONH, optic nerve head.
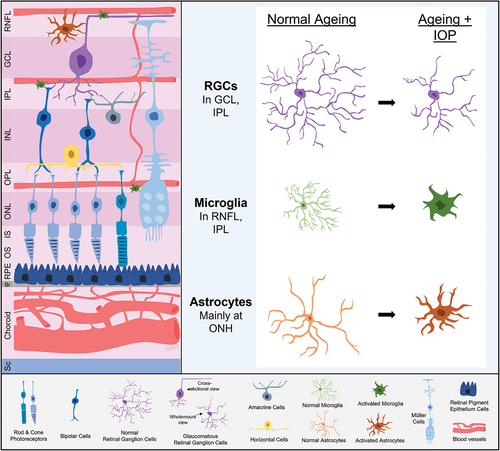
Age-related responses to IOP elevation
Are older eyes more susceptible to IOP elevation?
Rodent models have been useful in quantifying age-related susceptibility to IOP elevation. Acute IOP elevation (60° head-down position, ~5 mmHg increase) resulted in a 65% reduction in RGC function in 10-month-old DBA/2J mice (a model of spontaneous glaucomatous neuropathy), but no change in 3-month-old counterparts.Citation16 It is worth noting that 3-month-old DBA/2J mice had normal baseline IOP, whereas 10-month-old mice showed a spontaneous increase in baseline IOP, thus the RGC dysfunction in the older cohort reflects the effect of an acute IOP elevation overlaid on older age and mild basal chronic IOP elevation. Nonetheless, this finding is consistent with the idea that older eyes are functionally more susceptible to IOP elevation.
In normal rodents, 14-month-old rats showed slower retinal functional recovery from a stepwise IOP elevation from 10 to 100 mmHg compared with 3-month-old rats.Citation17 Similarly, 12-month-old mice showed greater susceptibility to IOP elevation to 50 mmHg, and poorer recovery compared with 3-month-old animals, an effect that was further exacerbated at 18 months of age.Citation14,Citation18
Anatomically, despite similar levels of chronic IOP elevation, older rats showed approximately double the RGC loss (74% in 18-month-old vs 36% in 3-month-old) and three times the magnitude of retinal nerve fibre layer thinning (21% in 9.5-month-old vs 7% in 2-month-old).Citation19,Citation20 Even with shorter periods of IOP elevation, for example acute IOP elevation from 10 to 100 mmHg (10 mmHg steps each lasting 3 minutes), there was greater thinning of the retinal nerve fibre layer in 18-month-old rats compared to 3-month-old rats.Citation21 Greater age-related susceptibility has also been exposed with an acute IOP elevation (50 mmHg for 30 minutes), after which 12-month-old mice showed inner plexiform layer thinning.Citation22 Why RGC function in older eyes is more susceptible and slower to recover from IOP elevation is a topic of interest, which will be discussed below.
Retinal ganglion cell adaptations to IOP elevation
Ganglion cell axons
Axonal transport of mitochondria was similar in mice between 2 and 23 months of age.Citation23 Whether this can be maintained in the presence of chronic IOP elevation is an important determinant of axonal and RGC injury.Citation24 After 2 weeks of chronic IOP elevation (5 to 10 mmHg higher than controls), there was a 60% reduction in anterograde axonal transport in 7- to 9-month-old rats, but not in 3- to 4-month-old rats.Citation25 However, others reported similar anterograde axonal transport disruption between 2- and 9.5-month-old rats after chronic IOP elevation for 3 weeks.Citation20 Age-related deficits may be more profound in rodents of older ages. Comparing 23- to 25-month-old mice to 4-month-old counterparts, there were greater reductions in both anterograde and retrograde transport after 2 weeks of IOP elevation.Citation23
Few studies have examined axonal transport recovery from stress. In a recoverable stress model (50 mmHg for 30 minutes), anterograde transport was similar between 3- and 18-month-old mice at day 7 after injury although RGC function had not recovered in older eyes.Citation18,Citation26 Whilst axonal transport speed may be normal, it may take time to restore the cellular components needed for normal function. A more complete time course of axonal transport recovery from stress would provide novel insights.
Ganglion cell dendrites
It is now well documented that RGC dendrites remodel in glaucoma. Human eyes with advanced glaucoma had smaller RGC dendritic trees and fewer dendritic bifurcations.Citation27 Similar findings have been noted in rodent models of glaucoma.Citation28,Citation29 In general, studies reported reductions in RGC dendritic field size, complexity or length following chronic IOP elevation in 3- to 6-month-old rodents.Citation28,Citation29 Comparing 24-month-old to 3-month-old mice, there was a greater decline in RGC dendritic complexity, with the same level of chronic IOP elevation (9 mmHg higher than controls).Citation30
The extent of remodelling in response to IOP elevation appears to be RGC specific.Citation31 In general, in 1- to 4-month-old mice, OFF RGCs are more likely to undergo earlier dendritic remodelling in response to chronic IOP elevation.Citation31,Citation32 Furthermore, spontaneous activity and light-evoked receptive field size were reduced in OFF-transient RGCs after IOP elevation in young mice.Citation31,Citation32 Consistent with these observations, 3 days after acute mild IOP elevation in 13-month-old mice, reduced spontaneous and light-evoked responses were evident in OFF RGCs, but not in ON RGCs.Citation33
Age-related differences were directly addressed by Lee et al., by examining RGC remodelling 7 days after acute mild IOP elevation, a time point where RGC function had recovered in young but not older mice.Citation14 IOP elevation in 3- to 5-month-old mice resulted in changes to both ON- and OFF-RGCs; ON RGC dendritic fields became smaller and OFF-RGC dendritic fields became less complex.Citation14 In contrast, 12- to 14-month-old mice showed smaller ON-RGC dendritic fields with little change to OFF-RGCs.Citation14 These data suggest that OFF-RGCs dendritic field changes are associated with better functional recovery, and that attenuation of this adaptive response in older eyes is associated with poorer functional recovery from stress. Further studies to examine how these responses are altered with chronic IOP elevation are warranted.
Ganglion cell synapses
Several studies have investigated RGC synaptic changes in response to IOP elevation in young rodents. As with IOP-induced morphological changes, synaptic changes are also RGC type specific, with reduction in synapses more evident in the inner plexiform layer OFF-sublamina in 1- to 4-month-old mice.Citation31,Citation32 In 2-month-old rats, synaptic disconnection occurred 5 days after IOP elevation (~10 mmHg higher than controls), which preceded cell death at 14 days.Citation34 After 8 weeks of IOP elevation (~17 mmHg higher than controls), there was a decrease in inner plexiform layer ribbon synapse density.Citation35 Perhaps in compensation, the total number of synaptic vesicles per synapse was increased along with evidence of immature synapse formation.Citation36 How dynamic synaptic plasticity responds to IOP elevation is as yet unclear. Nevertheless, restoration of synapsesCitation35 or prevention of complement-mediated synapse elimination both via brain-derived neurotrophic factor-dependent pathwaysCitation22 can improve RGC function.
Whilst it is accepted that RGCs undergo synaptic, dendritic and axonal adaptations in response to IOP elevation, how ageing impedes these adaptations leading to altered functional responses requires further investigations.
Biomechanical changes with age
The sclera, a tough collagenous connective tissue, is the primary load-bearing tissue of the eye. At the optic nerve head, the lamina cribrosa forms a perforated scleral structure spanning the scleral canal. IOP exerts pressure from within the eye anterior to the lamina cribrosa, whereas cerebrospinal fluid exerts pressure on the optic nerve through the sheath surrounding the post-laminar optic nerve. This creates a pressure gradient, known as the translaminar pressure difference. A lower cerebrospinal fluid pressure with age,Citation37 coupled with normal or high IOP, creates an elevated translaminar pressure difference which is thought to place greater biomechanical stress on the lamina cribrosa.
In human donor eyes, multiple studies have shown age-related stiffening of the sclera and lamina cribrosa ().Citation38,Citation39 RGC axons and their supporting capillaries are vulnerable to the additional biomechanical stress encountered as they traverse the lamina cribrosa. Recent advances in enhanced depth spectral-domain optical coherence tomography imaging have allowed measurements of lamina cribrosa deformation and estimation of the strain response to IOP changes in living human eyes.Citation40,Citation41 This lays a foundation for future clinical studies to investigate the age-related changes in glaucoma pathogenesis.
Age-related remodelling of optic nerve head-related connective tissues can magnify IOP-related stress on RGC axons.Citation42 Ageing leads to reduced turnover and altered connective tissue composition, including increased collagen and reduced elastic fibres within the sclera and lamina cribrosa.Citation39,Citation42 Increased IOP compounds age-related connective tissue stiffening. In particular, increasing IOP from 25 to 50 mmHg in human donor eyes leads to stiffer scleral mechanical properties, with higher peak magnitudes of compressive and extensional principal strain (114% and 98% compared to 24% and 35% in compliant sclera, respectively).Citation38 Age-related connective tissue alterations are thought to make tissues less compliant, therefore less capable of absorbing mechanical stress leading to excessive deformation of the lamina cribrosa and optic nerve head tissues,Citation38 increasing the risk of RGC injury.Citation42
Even though the rodent optic nerve head lacks a well-defined collagenous lamina cribrosa, IOP elevation led to increased nasal-temporal directional strains on the astrocyte-dominated glial lamina for both young (2- to 4-month-old) and older (13- to 15-month-old) mouse eyes, but superior-inferior strains only in the younger eyes.Citation43 Similar to humans, slower collagen turnover with age leads to stiffening of scleral tissues.Citation44 In rats, stiffer scleral tissues may contribute to reduced anterior retinal surface deformation in older (18-month-old) compared to younger eyes (3-month-old).Citation21
In the anterior eye, ageing increases pigment and debris deposition in the trabecular meshwork which results in increased outflow resistance.Citation45 Additionally, age-related reduction in the density and size of giant vacuoles and intracellular pores lining Schlemm’s canal leads to impaired endothelial outflow facility in older eyes.Citation46 These factors interfere with aqueous humour drainage, possibly leading to higher IOP and subjecting the RGC axons at the lamina cribrosa to damage by both vascular deficiency and mechanical strain.Citation42 Age-related impairment in aqueous outflow, coupled with scleral tissue stiffening and increased strain at the optic nerve head, may contribute to the higher susceptibility of RGC injury in older eyes.
Vascular changes with age
Poor vascular perfusion of the optic nerve head and supporting structures is thought to contribute to progressive RGC loss.Citation47 Low ocular perfusion pressure (the difference between arterial pressure in the ophthalmic artery and IOP) has been consistently associated with glaucoma.Citation47 Maintaining adequate blood flow, in the face of a constantly variable perfusion pressure (fluctuations in blood pressure or IOP) involves local modulators of vascular tone, via a process known as autoregulation.Citation47 Autoregulation occurs for blood pressures ranging from ~60 to 150 mmHg, where the arteries constrict or dilate at the higher and lower pressures, respectively to maintain blood flow.Citation48
With increasing age, there was a smaller change of retinal vessel density in the parafoveal region when blood pressure was increased, suggesting an age-related impairment in autoregulation.Citation49 Impairment in vascular autoregulation may be improved, as shown in restoration of blood flow instability induced by postural changes after 8 weeks of brimonidine treatment in normal tension glaucoma.Citation50
Ageing leads to degenerative changes to the vascular endothelium, loss of pericytes and vascular smooth muscle cells, and hyalinisation of arterial walls.Citation51 In addition to endothelial cell dropout, ageing also increases vascular endothelial dysfunction due to both increased oxidative stress and chronic low-grade sterile inflammation, termed ‘inflammaging’, leading to overexpression of pro-inflammatory cytokines.Citation52 This can compromise autoregulation, affecting the capacity of the eye to maintain adequate tissue perfusion.Citation52 Furthermore, age-related changes to the biomechanical properties of connective tissue, as previously discussed, also occur in the vascular tree with blood vessel remodelling resulting in increased arterial wall stiffness and thickness ().Citation52
Thickening and hardening of the vessel walls reduce the maximal vasodilatory capacity of vessels, narrowing the range of perfusion pressures over which autoregulation can be effective. In the context of glaucoma, autoregulatory failure can lead to repeated episodes of hypoperfusion and ischaemia, particularly in cases where IOP is unstable, ultimately leading to RGC dysfunction and loss.Citation47
Using optical coherence tomography angiography, age-related reductions in vessel density in the optic nerve head and peripapillary area are evident from the 6th decade of life onwards.Citation53 In glaucoma patients, loss of vessel density appears to be accelerated and is associated with conversion from suspect to manifest glaucoma, retinal nerve fibre layer thinning, as well as visual field loss and progression.Citation54,Citation55
It is important to note that there are some limitations to the current paradigms in clinical practice: (1) measurements vary between instruments and comparisons between devices are challenging; (2) test–retest variabilities limit the differentiation of disease-related changes from normal age-related changes; and (3) variability in diagnostic ability depending on glaucoma severity, e.g., better in advanced glaucoma due to its expansion of structural dynamic range.Citation56 Nonetheless, current evidence suggests that compromise to the ocular microcirculation may be one of the driving factors of increasing prevalence of glaucoma with age.
Although Alnawaiseh et al. did not find a difference in retinal vessel density between 3- to 4-month-old and 18- to 24-month-old mice,Citation57 it has been shown in mice that the intercommunication between blood vessels mediated by pericytes is damaged by IOP elevation, resulting in impaired local regulation to increased metabolic demand induced by flickering light.Citation58 Whether blood vessels and their interconnections in older eyes are more easily damaged by IOP elevation requires further investigation.
As described in this section, current evidence suggests that vascular insufficiency and compromised perfusion of the RGCs and supporting structures are associated with glaucoma development and progression. Age-related alterations in vascular reactivity and vessel density, converge with this increasing risk of glaucoma in cases of vascular insufficiency, culminate in a likely relationship between ageing and glaucoma risk driven by altered ability to sustain adequate tissue perfusion with older age.
Oxidative stress with age
Ageing is also associated with reduced energy availability and increased oxidative stress.Citation59 Age-related impairment of the body’s antioxidant defence mechanisms leads to a greater susceptibility to oxidative damage.Citation59 RGCs house a high density of mitochondria throughout their long axons to regulate ion gradients for generating action potentials. Mitochondria maintain oxidative energy metabolism and regulate intracellular calcium homoeostasis.Citation60
Age-related mitochondrial dysfunction in RGCs is thought to contribute to glaucomatous neurodegeneration.Citation61 In DBA/2J mice, increased mitochondrial stress markers are seen in the ganglion cell layer along with antioxidant and metabolic substrates.Citation62 Mitochondrial changes in RGCs may be one of the earlier markers of glaucoma onset, as they have been reported to coincide with early attenuations in electroretinogram response.Citation62 Mitochondrial DNA mutations, that can occur with ageing, increase the chance of free radical production, contributing to RGC vulnerability to IOP injury.Citation63
Autophagy facilitates the degradation and recycling of defective intracellular components and toxic foreign substances, a process highly critical for cell survival.Citation64 In states of extreme metabolic stress, autophagy can facilitate cell death. Age-related failure of such systems can impair cellular capacity to cope with elevated IOP.Citation61
IOP elevation is known to trigger autophagy in trabecular meshwork cells and retinal neurons.Citation65 Experimental activation of autophagy returns conflicting results,Citation66,Citation67 which likely reflects a complex interplay between a range of cell maintenance processes including the proteasome and cargo-specific autophagy processes. Moreover, studies of autophagy in rodent models of glaucoma have largely employed young animals, thus the impact of ageing is not well understood.Citation65
Using a transgenic mouse strain with intrinsically fluorescent autophagy markers (microtubule-associated proteins light chain 3, LC3), autophagy was found to significantly reduce with age in the outer retina.Citation68 Following acute IOP elevation (50 mmHg for 30 minutes), alongside poorer functional recovery at 3 days after injury, a reduced capacity to upregulate LC3 expression was observed in the inner retina of older eyes, unlike younger eyes.Citation68 This suggests that ageing may impair both basal and stress-induced autophagic responses in the eye.
In a chronic IOP elevation model (injection of hypertonic saline in the limbal vein), increased LC3 immunofluorescence is associated with greater RGC and axonal loss in older mouse eyes (18-month-old) compared with younger eyes (4-month-old).Citation69 Increased LC3 staining may be indicative of reduced autophagic flux and impairment of lysosome acidification as it is trafficked back to the cell body. Such an impairment of lysosome activity could arise from age and/or IOP induced axonal transport which is required for lysosome transport.Citation70
Other studies have examined the role of autophagy in ageing and its age-related RGC susceptibility to injury using Ambra1+/gt mice, a transgenic mouse model of subtle autophagy deficiency.Citation71 There were impaired oxidative stress responses and altered mitochondrial dynamics in 12- to 14-month-old autophagy deficient mouse eyes compared with younger eyes (5- to 6-month-old).Citation71 Importantly, these older mice showed decreased RGC survival following optic nerve crush.Citation71 Current evidence suggests that age-related impairment in autophagy may increase RGC susceptibility to IOP elevation in older eyes. With a growing interest towards the topic of autophagy and age-related neurodegenerative diseases, further investigation of its role in glaucomatous injury may be valuable.
Glial cell changes with age
Interactions between RGCs and retinal macroglia, including Müller cells and astrocytes, and microglia, are also crucial in regulating stress responses within the retina.
Microglia
Microglia, the resident population of immune cells, survey their surrounding environment and monitor neural activity through various neurotransmitter receptors.Citation72 They are also involved in defence against invading pathogens, including release of inflammatory mediators, clearance of debris and waste products and critically, refinement and maintenance of synaptic architecture.Citation72 Microglia are a long-lived population of cells (~4 years in humans), and uniquely retain their proliferative capacity to self-renew through cell division in the mature retina.Citation73 However, this makes them susceptible to senescence, as accumulation of DNA damage and telomere attrition eventually render the cell incapable of division and potentially less efficient at performing their normal, homoeostatic housekeeping duties.Citation73
With age, microglia showed increased expression of major histocompatibility complex class II, increased expression of components of the complement cascade (particularly C3), increased expression of pro-inflammatory cytokines, and altered transcriptomic profiles more similar to microglia in pathological states.Citation74,Citation75 Their morphology also becomes more rounded and their processes retract with reduced process motility.Citation76 Some rodent studies reported age-related upregulation of phagocytic activity, whereas others showed impaired phagocytosis.Citation77,Citation78 Either case may detrimentally affect neuronal health as upregulation of phagocytic behaviour may lead to aberrant destruction of functional neurons, whereas deficient phagocytic activity may lead to the accumulation of debris, waste products and noxious protein aggregates in the tissue. From this evidence, microglia in healthy ageing drift towards a more pro-inflammatory phenotype, with reduced surveillance capabilities.
Furthermore, ageing may also alter how microglia respond to injury. Older (18- to 24-month-old) microglia have been shown to polarise their processes and migrate towards the site of a focal laser injury slower than 2- to 3-month-old mice, as well as being slower to de-aggregate following this injury.Citation79 Together these findings suggest that with ageing, microglia are slower to respond to injury, and their responses may be disproportionate and aberrantly sustained, which may also damage neighbouring tissue.
In the context of glaucoma, microglia have been found to be more numerous and activated in human donor tissues and rodent glaucoma models, with increased expression of pro-inflammatory cytokines (e.g., TNFα) and complement components (e.g., C1q).Citation80,Citation81 In both acute and chronic rodent models of IOP elevation, microglia in 15-month-old mouse retinae show higher levels of activation than in 2- to 3-month-old eyes.Citation77,Citation82 However, it is less clear whether increased microglial activity is in response to RGC pathology, or whether it plays an active role in RGC loss. In DBA/2J mice, microgliosis at the optic nerve head preceded neurodegeneration by up to 7 months, and the degree of microgliosis was predictive of neurodegeneration severity.Citation83 Additionally, transgenic knockout of C1q or pharmacological inhibition of C1 in DBA/2J mice led to reduced RGC neurodegeneration.Citation80,Citation84
Pharmacological inhibition of microglial activation has also shown to attenuate RGC loss in rodent RGC injury models in rats treated with either optic nerve transection or translimbal laser photocoagulation.Citation85 These studies suggest that suppression of microglial activity is of potential therapeutic benefit in glaucoma. The reality is more nuanced, as the complex and dynamic nature of microglial behaviour in neurodegenerative diseases is not fully understood. There needs to be a deeper investigation into which aspects of microglial neurobiology are beneficial or detrimental in neurodegenerative diseases.
Astrocytes
The role of astrocytes in the optic nerve head is also of crucial importance to RGC health. Astrocytes, like microglia, are involved in neurotransmitter homoeostasis, expression of immune modulating factors including components of the complement system, synapse maintenance, extracellular ion homoeostasis and extracellular matrix composition.Citation86 In glaucoma, their roles include maintenance of the blood-retinal-barrier and regulation of ocular blood flow via neurovascular coupling and in connective tissue remodelling in the optic nerve head.Citation87,Citation88 Astrocytes are also present in the retinal nerve fibre layer, however activation of retinal astrocytes in the retina appears to be regional and mostly present in the later stages of glaucomatous pathology, whereas astrocyte behaviour in the optic nerve head seems to be more closely linked with RGC health in glaucoma.Citation89 This review will principally focus on the optic nerve head astrocytes.
Astrocytes are affected by non-pathological ageing, and this altered state may favour neuronal injury rather than survival. Unlike microglia, astrocytes do not proliferate beyond development under normal circumstances, however they can regain their proliferative capacity under pathological circumstances.Citation90 With ageing, astrocytes have shorter and thicker processes, and become pro-inflammatory.Citation91 Whilst there is evidence that astrocytes are involved in blood neural barrier alteration in neurodegenerative diseases, whether this also occurs in normal ageing in the eye is yet to be determined.Citation91 Compromise to the blood-retinal-barrier as a result of astrocyte ageing may be detrimental to RGC health as circulating peripheral immune cells gain access to an immune privileged eye. These sites represent areas of compromise to the blood-retinal-barrier, where location and frequency of occurrence of optic disc haemorrhages are associated with glaucoma progression.Citation92 Whether the associated progression is linked to ischaemia or immune cell infiltration into the retina, or both is unclear.
In the DBA/2J mouse model, at around 6 months of age, prior to axon loss, astrocyte processes retract and redistribute towards the outer portion of the nerve before later redistributing into areas of axonal loss forming a gliotic scar by around 11 months of age.Citation93 Liddelow et al. identified a neurotoxic astrocyte phenotype capable of inducing RGC death via interaction with microglia, mediated by microglial secretion of IL-1α, TNF and C1q.Citation94 This neurotoxic subtype of astrocytes has also been reported to have impaired phagocytic capabilities and increased C3 expression, as well as reduced expression of trophic factors.Citation95 Whether this type of astrocytes alter the age-related RGC responses to IOP elevation is unknown.
Müller cells
Müller cells, the most abundant glial cell type in the vertebrate retina, have a radial organisation and extensive processes that allow contact with all retinal cell types.Citation96 In a rat model of glaucoma, increased IOP was shown to be associated with increased transient receptor potential vanilloid-type 4 (TRPV4) expression in Müller cells.Citation97 Pharmacological activation of TRPV4 channel induced Müller cell gliosis and release of cytotoxic TNFα, a potential pathway to induce RGC death.Citation97 Additionally, mechanical stimulation of Müller cells can prompt adenosine tri-phosphate release, which can lead to Müller cell gliosis via activation of P2Y receptors on Müller cell membranes, and RGC death via calcium influx due to P2×7 receptor activation on RGCs.Citation98 Interestingly, in 13-month-old mice, genetic ablation of P2×7receptor did not rescue RGC dysfunction following an acute IOP elevation, suggesting older Müller cells respond differently to injury.Citation33
Two studies using RNA sequencing in Müller cells found common upregulated pathways in 18-month-old mice without injury, compared to 2-month-old mice with excitotoxicity injury,Citation99 and an over-representation of pathways associated with neuroinflammation and fibrosis in 24-month-old mice compared to 4-month-old mice.Citation100 These results suggest that Müller cell physiology in ageing may mirror the drift towards a pro-inflammatory state that favours neuronal death rather than survival as seen in microglia and astrocytes, however there is not yet a strong evidence base in the literature to support this idea. The regenerative potential of Müller cells in lower vertebrates, such as zebrafish, and how this may be translated into mammalian retina is a key area of interest but has yet to be explored in mouse models of glaucoma or normal ageing.
As outlined in this section, it appears that ageing can play a role in tipping the balance towards neuronal injury rather than survival, with glial cells potentially drifting to a more pro-inflammatory phenotype. In many cases, glial behaviour is altered in glaucoma, however it is often unclear whether these altered glial states are an attempt to preserve RGC function, or an aberrant state that is actively involved in propagating RGC injury. How the neuroprotective potential of these cell classes can be harnessed in new therapies for glaucoma is a rapidly advancing area of glaucoma research.
Conclusions
This review outlines factors altered with increasing age that are important in glaucoma pathogenesis, such as RGC adaptations, biomechanical and vascular changes, oxidative stress, and glial cells (Müller cells, astrocytes and microglia) in response to IOP elevation. However, other aspects have not been covered by the scope of this review such as age-related changes to various classes of receptors on RGC membranes, in addition to myriad systemic co-morbidities that increase in prevalence with ageing and can alter glaucoma risk. Furthermore, many of the factors mentioned interact with one another, such as the relationship between deficient autophagy, oxidative stress and axonal transport, which then in turn can influence vascular function. How all these factors intersect, and how ageing may alter these relationships is complex and yet to be fully understood.
Ageing and elevated IOP remain the main risk factors for glaucoma. Whilst ageing itself is a relentless process, dissecting the ageing process into various components such as those outlined in this review, may highlight potential aspects of ageing that could be mitigated with the development of new therapies.
Disclosure statement
No potential conflict of interest was reported by the author(s).
Additional information
Funding
References
- Tham YC, Li X, Wong TY et al. Global prevalence of glaucoma and projections of glaucoma burden through 2040: a systematic review and meta-analysis. Ophthalmology 2014; 121: 2081–2090. doi:10.1016/j.ophtha.2014.05.013.
- Quigley HA. Glaucoma. Lancet 2011; 377: 1367–1377. doi:10.1016/S0140-6736(10)61423-7.
- Owsley C. Aging and vision. Vision Res 2011; 51: 1610–1622. doi:10.1016/j.visres.2010.10.020.
- Birch DG, Anderson JL. Standardized full-field electroretinography. Normal values and their variation with age. Arch Ophthalmol 1992; 110: 1571–1576. doi:10.1001/archopht.1992.01080230071024.
- Lek JJ, Nguyen BN, McKendrick AM et al. An electrophysiological comparison of contrast response functions in younger and older adults, and those with glaucoma. Invest Ophthalmol Vis Sci 2019; 60: 442–450. doi:10.1167/iovs.17-23522.
- Demirkaya N, van Dijk HW, van Schuppen SM et al. Effect of age on individual retinal layer thickness in normal eyes as measured with spectral-domain optical coherence tomography. Invest Ophthalmol Vis Sci 2013; 54: 4934–4940. doi:10.1167/iovs.13-11913.
- Biswas S, Wan KH. Review of rodent hypertensive glaucoma models. Acta Ophthalmol 2019; 97: e331–e340. doi:10.1111/aos.13983.
- Flurkey K, Currer JM, Harrison DE. Chapter 20 - mouse models in aging research. In: Fox J, Davisson M, Quimby F, Barthold S, Newcomer C, Smith A, editors. The mouse in biomedical research 2nd ed. In American College of Laboratory Animal Medicine. Cambridge, MA: Academic Press; 2007. p. 637–672.
- Sugita Y, Yamamoto H, Maeda Y et al. Influence of aging on the retina and visual motion processing for optokinetic responses in mice. Front Neurosci 2020; 14: 586013. doi:10.3389/fnins.2020.586013.
- Li C, Cheng M, Yang H et al. Age-related changes in the mouse outer retina. Optom Vis Sci 2001; 78: 425–430. doi:10.1097/00006324-200106000-00015.
- Samuel MA, Zhang Y, Meister M et al. Age-related alterations in neurons of the mouse retina. J Neurosci 2011; 31: 16033–16044. doi:10.1523/JNEUROSCI.3580-11.2011.
- Nadal-Nicolas FM, Vidal-Sanz M, Agudo-Barriuso M. The aging rat retina: from function to anatomy. Neurobiol Aging 2018; 61: 146–168. doi:10.1016/j.neurobiolaging.2017.09.021.
- Charng J, Nguyen CT, Bui BV et al. Age-related retinal function changes in albino and pigmented rats. Invest Ophthalmol Vis Sci 2011; 52: 8891–8899. doi:10.1167/iovs.11-7602.
- Lee PY, Zhao D, Wong VHY et al. The effect of aging on retinal function and retinal ganglion cell morphology following intraocular pressure elevation. Front Aging Neurosci 2022; 14: 859265. doi:10.3389/fnagi.2022.859265.
- Williams PA, Morgan JE, Votruba M. Opa1 deficiency in a mouse model of dominant optic atrophy leads to retinal ganglion cell dendropathy. Brain 2010; 133: 2942–2951. doi:10.1093/brain/awq218.
- Nagaraju M, Saleh M, Porciatti V. IOP-dependent retinal ganglion cell dysfunction in glaucomatous DBA/2J mice. Invest Ophthalmol Vis Sci 2007; 48: 4573–4579. doi:10.1167/iovs.07-0582.
- Lim JK, Nguyen CT, He Z et al. The effect of ageing on ocular blood flow, oxygen tension and retinal function during and after intraocular pressure elevation. PloS One 2014; 9: e98393. doi:10.1371/journal.pone.0098393.
- Kong YX, van Bergen N, Bui BV et al. Impact of aging and diet restriction on retinal function during and after acute intraocular pressure injury. Neurobiol Aging 2012; 33: 1126 e1115–1125. doi:10.1016/j.neurobiolaging.2011.11.026.
- Levkovitch-Verbin H, Vander S, Makarovsky D et al. Increase in retinal ganglion cells’ susceptibility to elevated intraocular pressure and impairment of their endogenous neuroprotective mechanism by age. Mol Vis2013; 19: 2011–2022.
- Abbott CJ, Choe TE, Burgoyne CF et al. Comparison of retinal nerve fiber layer thickness in vivo and axonal transport after chronic intraocular pressure elevation in young versus older rats. PloS One 2014; 9: e114546. doi:10.1371/journal.pone.0114546.
- Zhao D, Nguyen CTO, He Z et al. Age-related changes in the response of retinal structure, function and blood flow to pressure modification in rats. Sci Rep 2018; 8: 2947. doi:10.1038/s41598-018-21203-5.
- Chrysostomou V, Galic S, van Wijngaarden P et al. Exercise reverses age-related vulnerability of the retina to injury by preventing complement-mediated synapse elimination via a BDNF-dependent pathway. Aging Cell 2016; 15: 1082–1091. doi:10.1111/acel.12512.
- Takihara Y, Inatani M, Eto K et al. In vivo imaging of axonal transport of mitochondria in the diseased and aged mammalian CNS. Proc Natl Acad Sci U S A 2015; 112: 10515–10520. doi:10.1073/pnas.1509879112.
- Chidlow G, Ebneter A, Wood JP et al. The optic nerve head is the site of axonal transport disruption, axonal cytoskeleton damage and putative axonal regeneration failure in a rat model of glaucoma. Acta Neuropathol 2011; 121: 737–751. doi:10.1007/s00401-011-0807-1.
- Crish SD, Sappington RM, Inman DM et al. Distal axonopathy with structural persistence in glaucomatous neurodegeneration. Proc Natl Acad Sci U S A 2010; 107: 5196–5201. doi:10.1073/pnas.0913141107.
- Fahy ET, Chrysostomou V, Abbott CJ et al. Axonal transport along retinal ganglion cells is grossly intact during reduced function post-injury. Exp Eye Res 2016; 146: 289–292. doi:10.1016/j.exer.2016.03.001.
- Pavlidis M, Stupp T, Naskar R et al. Retinal ganglion cells resistant to advanced glaucoma: a postmortem study of human retinas with the carbocyanine dye DiI. Invest Ophthalmol Vis Sci 2003; 44: 5196–5205. doi:10.1167/iovs.03-0614.
- Ahmed FA, Chaudhary P, Sharma SC. Effects of increased intraocular pressure on rat retinal ganglion cells. Int J Dev Neurosci 2001; 19: 209–218. doi:10.1016/S0736-5748(00)00073-3.
- Tribble JR, Williams PA, Caterson B et al. Digestion of the glycosaminoglycan extracellular matrix by chondroitinase ABC supports retinal ganglion cell dendritic preservation in a rodent model of experimental glaucoma. Mol Brain 2018; 11: 69. doi:10.1186/s13041-018-0412-5.
- Di Pierdomenico J, Henderson DCM, Giammaria S et al. Age and intraocular pressure in murine experimental glaucoma. Prog Retin Eye Res 2022; 88: 101021. doi:10.1016/j.preteyeres.2021.101021.
- Della Santina L, Inman DM, Lupien CB et al. Differential progression of structural and functional alterations in distinct retinal ganglion cell types in a mouse model of glaucoma. J Neurosci 2013; 33: 17444–17457. doi:10.1523/JNEUROSCI.5461-12.2013.
- Ou Y, Jo RE, Ullian EM et al. Selective vulnerability of specific retinal ganglion cell types and synapses after transient ocular hypertension. J Neurosci 2016; 36: 9240–9252. doi:10.1523/JNEUROSCI.0940-16.2016.
- Wang AYM, Wong VHY, Lee PY et al. Retinal ganglion cell dysfunction in mice following acute intraocular pressure is exacerbated by P2X7 receptor knockout. Sci Rep 2021; 11: 4184. doi:10.1038/s41598-021-83669-0.
- Fu QL, Li X, Shi J et al. Synaptic degeneration of retinal ganglion cells in a rat ocular hypertension glaucoma model. Cell Mol Neurobiol 2009; 29: 575–581. doi:10.1007/s10571-009-9349-7.
- Park HL, Kim SW, Kim JH et al. Increased levels of synaptic proteins involved in synaptic plasticity after chronic intraocular pressure elevation and modulation by brain-derived neurotrophic factor in a glaucoma animal model. Dis Model Mech 2019; 12. doi:10.1242/dmm.037184.
- Park HY, Kim JH, Park CK. Alterations of the synapse of the inner retinal layers after chronic intraocular pressure elevation in glaucoma animal model. Mol Brain 2014; 7: 53. doi:10.1186/s13041-014-0053-2.
- Knier CG, Fleischman D, Hodge DO et al. Three-decade evaluation of cerebrospinal fluid pressure in open-angle glaucoma at a tertiary care center. J Ophthalmol 2020; 2020: 7487329. doi:10.1155/2020/7487329.
- Eilaghi A, Flanagan JG, Simmons CA et al. Effects of scleral stiffness properties on optic nerve head biomechanics. Ann Biomed Eng 2010; 38: 1586–1592. doi:10.1007/s10439-009-9879-7.
- Coudrillier B, Pijanka J, Jefferys J et al. Collagen structure and mechanical properties of the human sclera: analysis for the effects of age. J Biomech Eng 2015; 137: 041006. doi:10.1115/1.4029430.
- Wanichwecharungruang B, Kongthaworn A, Wagner D et al. Comparative study of lamina cribrosa thickness between primary angle-closure and primary open-angle glaucoma. Clin Ophthalmol 2021; 15: 697–705. doi:10.2147/OPTH.S296115.
- Czerpak CA, Kashaf MS, Zimmerman BK et al. The strain response to intraocular pressure decrease in the lamina cribrosa of patients with glaucoma. Ophthalmol Glaucoma 2023; 6: 11–22. doi:10.1016/j.ogla.2022.07.005.
- Liu B, McNally S, Kilpatrick JI et al. Aging and ocular tissue stiffness in glaucoma. Surv Ophthalmol 2018; 63: 56–74. doi:10.1016/j.survophthal.2017.06.007.
- Nguyen C, Midgett D, Kimball E et al. Age-related changes in quantitative strain of mouse astrocytic lamina cribrosa and peripapillary sclera using confocal microscopy in an explant model. Invest Ophthalmol Visual Sci 2018; 59: 5157–5166. doi:10.1167/iovs.18-25111.
- Ihanamäki T, Salminen H, Säämänen AM et al. Age-dependent changes in the expression of matrix components in the mouse eye. Exp Eye Res 2001; 72: 423–431. doi:10.1006/exer.2000.0972.
- Li Y, Wolf NS. Effects of age and long-term caloric restriction on the aqueous collecting channel in the mouse eye. J Glaucoma 1997; 6: 18–22. doi:10.1097/00061198-199702000-00005.
- Boldea RC, Roy S, Mermoud A. Ageing of Schlemm’s canal in nonglaucomatous subjects. Int Ophthalmol 2001; 24: 67–77. doi:10.1023/A:1016361426238.
- Flammer J, Orgul S, Costa VP et al. The impact of ocular blood flow in glaucoma. Prog Retin Eye Res 2002; 21: 359–393. doi:10.1016/S1350-9462(02)00008-3.
- Pires PW, Dams Ramos CM, Matin N et al. The effects of hypertension on the cerebral circulation. Am J Physiol Heart Circ Physiol 2013; 304: H1598–1614. doi:10.1152/ajpheart.00490.2012.
- Zong Y, Xu H, Yu J et al. Retinal vascular autoregulation during phase IV of the valsalva maneuver: an optical coherence tomography angiography study in healthy Chinese adults. Front Physiol 2017; 8: 553. doi:10.3389/fphys.2017.00553.
- Feke GT, Bex PJ, Taylor CP et al. Effect of brimonidine on retinal vascular autoregulation and short-term visual function in normal tension glaucoma. Am J Ophthalmol 2014; 158: 105–112 e101. doi:10.1016/j.ajo.2014.03.015.
- Nag TC, Maurya M, Roy TS. Age-related changes of the human retinal vessels: possible involvement of lipid peroxidation. Ann Anat 2019; 226: 35–47. doi:10.1016/j.aanat.2019.06.007.
- Ungvari Z, Tarantini S, Donato AJ et al. Mechanisms of vascular aging. Circ Res 2018; 123: 849–867. doi:10.1161/CIRCRESAHA.118.311378.
- Jo YH, Sung KR, Shin JW. Effects of age on peripapillary and macular vessel density determined using optical coherence tomography angiography in healthy eyes. Invest Ophthalmol Vis Sci 2019; 60: 3492–3498. doi:10.1167/iovs.19-26848.
- Nishida T, Moghimi S, Wu JH et al. Association of initial optical coherence tomography angiography vessel density loss with faster visual field loss in glaucoma. JAMA Ophthalmol 2022; 140: 319–326. doi:10.1001/jamaophthalmol.2021.6433.
- Lommatzsch C, Rothaus K, Koch JM et al. Vessel density in OCT angiography permits differentiation between normal and glaucomatous optic nerve heads. Int J Ophthalmol 2018; 11: 835–843. doi:10.18240/ijo.2018.05.20.
- Moghimi S, Hou H, Rao H et al. Optical coherence tomography angiography and glaucoma: a brief review. Asia Pac J Ophthalmol (Phila) 2019; 8: 115–125.
- Alnawaiseh M, Brand C, Bormann E et al. Quantitative analysis of retinal perfusion in mice using optical coherence tomography angiography. Exp Eye Res 2017; 164: 151–156. doi:10.1016/j.exer.2017.09.003.
- Alarcon-Martinez L, Shiga Y, Villafranca-Baughman D et al. Pericyte dysfunction and loss of interpericyte tunneling nanotubes promote neurovascular deficits in glaucoma. Proc Natl Acad Sci U S A 2022; 119: 119. doi:10.1073/pnas.2110329119.
- Cáceres-Vélez PR, Hui F, Hercus J et al. Restoring the oxidative balance in age-related diseases - an approach in glaucoma. Ageing Res Rev 2022; 75: 101572. doi:10.1016/j.arr.2022.101572.
- Liu H, Prokosch V. Energy metabolism in the inner retina in health and glaucoma. Int J Mol Sci 2021; 22: 22. doi:10.3390/ijms22073689.
- Lin WJ, Kuang HY. Oxidative stress induces autophagy in response to multiple noxious stimuli in retinal ganglion cells. Autophagy 2014; 10: 1692–1701. doi:10.4161/auto.36076.
- Williams PA, Harder JM, Foxworth NE et al. Vitamin B(3) modulates mitochondrial vulnerability and prevents glaucoma in aged mice. Sci 2017; 355: 756–760. doi:10.1126/science.aal0092.
- Kong YX, Van Bergen N, Trounce IA et al. Increase in mitochondrial DNA mutations impairs retinal function and renders the retina vulnerable to injury. Aging Cell 2011; 10: 572–583. doi:10.1111/j.1474-9726.2011.00690.x.
- Kaden TR, Li W. Autophagy, mitochondrial dynamics and retinal diseases. Asia Pac J Ophthalmol (Phila) 2013; 2: 341–348. doi:10.1097/APO.1090b1013e31829d31823e31833.
- Adornetto A, Parisi V, Morrone LA et al. The role of autophagy in glaucomatous optic neuropathy. Front Cell Dev Biol 2020; 8: 121. doi:10.3389/fcell.2020.00121.
- Russo R, Varano GP, Adornetto A et al. Rapamycin and fasting sustain autophagy response activated by ischemia/reperfusion injury and promote retinal ganglion cell survival. Cell Death Disease 2018; 9: 981. doi:10.1038/s41419-018-1044-5.
- Produit-Zengaffinen N, Pournaras CJ, Schorderet DF. Autophagy induction does not protect retina against apoptosis in ischemia/reperfusion model. Adv Exp Med Biol 2014; 801: 677–683.
- Afiat BC, Zhao D, Wong VHY et al. Age-related deficits in retinal autophagy following intraocular pressure elevation in autophagy reporter mouse model. Neurobiol Aging 2023; 131: 74–87. doi:10.1016/j.neurobiolaging.2023.07.009.
- Nettesheim A, Dixon A, Shim MS et al. Autophagy in the aging and experimental ocular hypertensive mouse model. Invest Ophthalmol Visual Sci 2020; 61: 31–31. doi:10.1167/iovs.61.10.31.
- Ferguson SM. Axonal transport and maturation of lysosomes. Curr Opin Neurobiol 2018; 51: 45–51. doi:10.1016/j.conb.2018.02.020.
- Bell K, Rosignol I, Sierra-Filardi E et al. Age related retinal ganglion cell susceptibility in context of autophagy deficiency. Cell Death Discovery 2020; 6: 21. doi:10.1038/s41420-020-0257-4.
- Xu Y, Jin MZ, Yang ZY et al. Microglia in neurodegenerative diseases. Neural Regen Res 2021; 16: 270–280. doi:10.4103/1673-5374.290881.
- Yoo HJ, Kwon MS. Aged microglia in neurodegenerative diseases: Microglia lifespan and culture methods. Front Aging Neurosci 2021; 13: 766267. doi:10.3389/fnagi.2021.766267.
- Candlish M, Hefendehl JK. Microglia phenotypes converge in aging and neurodegenerative disease. Front Neurol 2021; 12: 660720. doi:10.3389/fneur.2021.660720.
- Rutar M, Valter K, Natoli R et al. Synthesis and propagation of complement C3 by microglia/monocytes in the aging retina. PloS One 2014; 9: e93343. doi:10.1371/journal.pone.0093343.
- Hanslik KL, Marino KM, Ulland TK. Modulation of glial function in health, aging, and neurodegenerative disease. Front Cell Neurosci 2021; 15: 718324. doi:10.3389/fncel.2021.718324.
- Meng S, Wen D, Xiao J et al. Age of rats affects the degree of retinal neuroinflammatory response induced by high acute intraocular pressure. Dis Markers 2022; 2022: 9404977. doi:10.1155/2022/9404977.
- Li W. Phagocyte dysfunction, tissue aging and degeneration. Ageing Res Rev 2013; 12: 1005–1012. doi:10.1016/j.arr.2013.05.006.
- Damani MR, Zhao L, Fontainhas AM et al. Age-related alterations in the dynamic behavior of microglia. Aging Cell 2011; 10: 263–276. doi:10.1111/j.1474-9726.2010.00660.x.
- Howell GR, Macalinao DG, Sousa GL et al. Molecular clustering identifies complement and endothelin induction as early events in a mouse model of glaucoma. J Clin Invest 2011; 121: 1429–1444. doi:10.1172/JCI44646.
- Yuan L, Neufeld AH. Activated microglia in the human glaucomatous optic nerve head. J Neurosci Res 2001; 64: 523–532. doi:10.1002/jnr.1104.
- Ramirez AI, Fernandez-Albarral JA, Hoz R et al. Microglial changes in the early aging stage in a healthy retina and an experimental glaucoma model. Prog Brain Res2020; 256: 125–149.
- Bosco A, Romero CO, Breen KT et al. Neurodegeneration severity can be predicted from early microglia alterations monitored in vivo in a mouse model of chronic glaucoma. Dis Model Mech 2015; 8: 443–455. doi:10.1242/dmm.018788.
- Williams PA, Tribble JR, Pepper KW et al. Inhibition of the classical pathway of the complement cascade prevents early dendritic and synaptic degeneration in glaucoma. Mol Neurodegener 2016; 11: 26. doi:10.1186/s13024-016-0091-6.
- Bosco A, Inman DM, Steele MR et al. Reduced retina microglial activation and improved optic nerve integrity with minocycline treatment in the DBA/2J mouse model of glaucoma. Invest Ophthalmol Vis Sci 2008; 49: 1437–1446. doi:10.1167/iovs.07-1337.
- Hasel P, Liddelow SA. Astrocytes. Curr Biol 2021; 31: R326–R327. doi:10.1016/j.cub.2021.01.056.
- Schneider M, Fuchshofer R. The role of astrocytes in optic nerve head fibrosis in glaucoma. Exp Eye Res 2016; 142: 49–55. doi:10.1016/j.exer.2015.08.014.
- Prada D, Harris A, Guidoboni G et al. Autoregulation and neurovascular coupling in the optic nerve head. Surv Ophthalmol 2016; 61: 164–186. doi:10.1016/j.survophthal.2015.10.004.
- Tang Y, Chen Y, Chen D. The heterogeneity of astrocytes in glaucoma. Front Neuroanat 2022; 16: 995369. doi:10.3389/fnana.2022.995369.
- Guizzetti M, Kavanagh TJ, Costa LG. Measurements of astrocyte proliferation. Methods Mol Biol 2011; 758: 349–359.
- Palmer AL, Ousman SS. Astrocytes and aging. Front Aging Neurosci 2018; 10: 337. doi:10.3389/fnagi.2018.00337.
- An D, House P, Barry C et al. Recurrent optic Disc Hemorrhage and its association with visual field deterioration in glaucoma. Ophthalmol Glaucoma 2020; 3: 443–452. doi:10.1016/j.ogla.2020.06.004.
- Cooper ML, Crish SD, Inman DM et al. Early astrocyte redistribution in the optic nerve precedes axonopathy in the DBA/2J mouse model of glaucoma. Exp Eye Res 2016; 150: 22–33. doi:10.1016/j.exer.2015.11.016.
- Liddelow SA, Guttenplan KA, Clarke LE et al. Neurotoxic reactive astrocytes are induced by activated microglia. Nature 2017; 541: 481–487. doi:10.1038/nature21029.
- Quillen S, Schaub J, Quigley H et al. Astrocyte responses to experimental glaucoma in mouse optic nerve head. PloS One 2020; 15: e0238104. doi:10.1371/journal.pone.0238104.
- Newman E, Reichenbach A. The Muller cell: a functional element of the retina. Trends Neurosci 1996; 19: 307–312. doi:10.1016/0166-2236(96)10040-0.
- Li Q, Cheng Y, Zhang S et al. TRPV4-induced Muller cell gliosis and TNF-alpha elevation-mediated retinal ganglion cell apoptosis in glaucomatous rats via JAK2/STAT3/NF-kappaB pathway. J Neuroinflammation 2021; 18: 271. doi:10.1186/s12974-021-02315-8.
- Shinozaki Y, Koizumi S. Potential roles of astrocytes and Muller cells in the pathogenesis of glaucoma. J Pharmacol Sci 2021; 145: 262–267. doi:10.1016/j.jphs.2020.12.009.
- Lin S, Guo J, Chen S. Transcriptome and DNA methylome signatures associated with retinal Muller glia development, injury response, and aging. Invest Ophthalmol Vis Sci 2019; 60: 4436–4450. doi:10.1167/iovs.19-27361.
- Chucair-Elliott AJ, Ocanas SR, Pham K et al. Translatomic response of retinal Muller glia to acute and chronic stress. Neurobiol Dis 2022; 175: 105931. doi:10.1016/j.nbd.2022.105931.