Abstract
Acute kidney injury (AKI) and renal interstitial fibrosis are global clinical syndromes associated with high morbidity and mortality. Renal ischemia-reperfusion (I/R) injury, which commonly occurs during surgery, is one of the major causes of AKI. Nevertheless, an efficient therapeutic approach for AKI and the development of renal interstitial fibrosis is still lacking due to its elusive pathogenetic mechanism. Here, we showed that chitosan oligosaccharide (COS), a natural oligomer polysaccharide degraded from chitosan, significantly attenuates I/R-induced AKI and maintains glomerular filtration function by inhibiting oxidative stress, mitochondrial damage, and excessive endoplasmic reticulum stress both in vitro and in vivo. In addition, long-term administration of COS can also attenuate the proliferation of myofibroblasts, mitigate extra cellular matrix deposition, and thus inhibit the transition of AKI to chronic kidney disease through participating in metabolic and redox biological processes. Our findings provide novel insights into the protective role of COS against acute kidney injury.
Introduction
Acute kidney injury (AKI), defined by the rapid decline in renal excretory function, is a global clinical syndrome associated with high morbidity and mortality [Citation1]. Renal ischemia-reperfusion (I/R) injury commonly occurs during kidney transplantation, partial nephrectomy, and other complicated surgical operations and is one of the major causes of AKI [Citation2,Citation3]. In addition, studies have reported that AKI can contribute to the development of chronic kidney disease (CKD) and eventually end-stage renal disease (ESRD) [Citation4,Citation5], which is characterized by renal fibrosis, one of the leading causes of death worldwide. Currently, the pathological mechanisms underlying the development of AKI and CKD remain poorly understood. Therefore, there is still a lack of available prevention and reliable therapeutic strategies for the treatment of AKI and its progression to CKD. Dialysis and kidney transplantation are commonly used in clinical treatment, but owing to their high long-term costs and side effects, therapeutic strategies with natural nontoxicity, limited adverse reactions, and efficient curative effects are urgently needed for renal I/R injury.
Chitosan oligosaccharide (COS) is composed of β-(1,4)-linked D-glucosamine with a degree of polymerization 2–8, and is a natural oligomer polysaccharide degraded from chitosan [Citation6]. Compared to chitosan, COS shows higher water solubility, higher intestinal absorption efficiency, and lower viscosity [Citation7]. These excellent characteristics are beneficial for clinical applications as therapeutic agents. Recently, studies have shown that COS exhibits satisfactory therapeutic effects on many diseases [Citation6,Citation7], such as obesity [Citation8], diabetes [Citation9], Alzheimer’s disease [Citation10] and nonalcoholic fatty liver disease [Citation11]. Owing to its safety and nontoxic biological characteristics, the renoprotective effect of COS has been widely studied, and the therapeutic potential of COS in multiple kidney diseases has been proven, including diabetic nephropathy [Citation12], renal fibrosis induced by unilateral ureteral obstruction (UUO) [Citation13], and toxic nephropathy induced by glycerol, paraquat, or lipopolysaccharide (LPS) [Citation14–16]. However, the effects of COS on I/R injury-induced AKI and the development of renal interstitial fibrosis have not yet been reported.
In this study, using both in vivo and in vitro experiments, we demonstrated that oral administration of COS significantly attenuated I/R-induced AKI and maintained glomerular filtration function. This protective effect of COS is associated with inhibition of oxidative stress, mitochondrial damage, and excessive endoplasmic reticulum (ER) stress. Moreover, this study showed that long-term administration of COS could alleviate renal interstitial fibrosis induced by renal I/R injury.
Materials and methods
Reagents and antibodies
Antibodies against GRP78, CHOP, and α-SMA were purchased from Cell Signaling Technology (Danvers, MA, USA). Antibody against KIM-1 (kidney injury molecule-1) was purchased from R&D Systems (Minneapolis, MN, USA). Antibodies against GAPDH were purchased from Proteintech (Chicago, IL, USA). Antibodies against ATF-4 were purchased from Bioss (Beijing, China). Antibodies against β-tubulin were purchased from Elabscience (Wuhan, China). Antibody against Fibronectin 1 (FN1) were purchased from ZEN-BIOSCIENCE (Chengdu, China). Secondary antibodies and 4′,6-diamidino-2-phenylindole (DAPI) were purchased from Invitrogen (Carlsbad, CA, USA). N-acetylcysteine (NAC) was purchased from Yeasen (Shanghai, China). tert-Butyl hydroperoxide (TBHP) was purchased from Macklin (Shanghai, China). Fetal bovine serum (FBS) was purchased from Suero fetal bovine ester, Natocor-Industria Biologica (Cordoba, Argentina). Bovine serum albumin (BSA) was purchased from Biosharp (Anhui, China). Triton X-100 was purchased from SolarBio (Beijing, China). Radioimmunoprecipitation assay (RIPA) buffer was purchased from Epizyme (Shanghai, China). BeyoECL Plus reagent was purchased from Beyotime Biotechnology (Shanghai, China).
Preparation of COS
COS was prepared and purified in our laboratory as previously reported [Citation17]. In brief, chitosan was incubated with chitosanase (made in house) at pH 5.5 for 3 h at 45 °C. Chitosanase was then inactivated and removed by sedimentation with trichloroacetic acid. The pH of the hydrolysate was adjusted to neutral with a sodium hydroxide solution. After spray drying, desalting, and ethanol precipitation, purified COS was subjected to infrared spectroscopy (IR) analysis.
Animals
Male C57BL/6J mice (8–10 weeks old) were purchased from the Vital River Laboratory Animal Technology Co., Ltd. (Beijing, China). Mice were housed in a specific pathogen-free (SPF) animal room (12-h light-dark cycle, 20–24 degrees’ Celsius temperature and 40–70% humidity) and provided with unrestricted access to a standard diet and distilled water.
Replication of renal I/R injury models
To replicate AKI and subsequent AKI in CKD models, unilateral I/R injury with contralateral nephrectomy was performed using an established protocol. Briefly, C57BL/6J mice were randomly separated into five groups: (i) sham, (ii) I/R, (iii) I/R-COS100, (iv) I/R-COS200, and (v) I/R-NAC. C57BL/6J mice were anesthetized with an intraperitoneal injection of 25 mg/kg pentobarbital sodium. The mice were then placed prone on a homeothermic table to maintain body temperature during the operation. Abdominal skin and muscle were cut along the midline of the mice using fine scissors, and the renal artery was carefully exposed from the renal pedicle. In the Sham group, the abdominal skin and muscle were opened, and the left kidney was exposed without renal I/R and contralateral nephrectomy. In the I/R group, mice were subjected to renal artery clamping for 35 min (45 min for the AKI to CKD model) in the left kidney with right nephrectomy. After the surgery, the muscle layer and skin were closed using absorbable sutures. For I/R-COS100 group, based on the operation of I/R group, COS (100 mg/kg) was administrated daily by gavage starting from 3 days before the renal I/R injury operation until mice were sacrificed. In the I/R-COS200 group, the concentration of COS was changed to 200 mg/kg. For the I/R-NAC group, a single intraperitoneal injection of 100 mg/kg NAC, an inhibitor of reactive oxygen species (ROS), was administered based on the operation of the I/R group. Mice models were housed in a SPF facility until sacrifice at 2 or 60 days after surgery. Kidneys and serum were harvested and stored at −80 °C refrigerator or fixed in 10% neutral formalin, followed by paraffin embedding until further analysis. To assess the renal function of each group, serum creatinine (Cr) was measured on day 2 by the hospital medicine biochemical laboratory (the Affiliated Hospital of Qingdao University). All animal experimental protocols were approved by the Institutional Animal Ethics and Use Committee of the Qingdao University.
Cell culture and in vitro experiments
TCMK-1, a mouse renal tubule epithelial cell line, was maintained in DMEM medium plus 10% FBS and cultured at 37 °C, 95% air, and 5% CO2. Cultured TCMK-1 cells were randomly divided into four groups: (i) Control group: TCMK-1 cells were cultured in DMEM supplemented with 1% FBS for 24 h for starvation treatment and then cultured in DMEM supplemented with 10% FBS for an additional 24 h. (ii) TBHP group: After starvation, TCMK-1 cells were cultured in DMEM supplemented with 10% FBS and 150 µmol/L t-BHP for an additional 24 h. (iii) TBHP + NAC group: 5 mmol/L NAC was added to the culture medium during starvation treatment, followed by 150 µmol/L t-BHP for an additional 24 h. (iv) TBHP + COS group: 200 ug/ml was added to the culture medium during starvation treatment, followed by 150 µmol/L t-BHP for an additional 24 h. All in vitro experiments with TCMK-1 cells were performed in triplicates.
Renal histopathological assessment
For the renal histopathological study, kidney tissues from each group were fixed in 10% neutral buffered formalin solution and embedded in paraffin. Slides (5 μm) were used for hematoxylin and eosin (H&E) and Masson trichrome staining, respectively. Images of each slide were obtained using a light microscope (Nikon ECLPSE 80i, Japan) and assessed blindly by a single pathologist. The renal tubular injury score was analyzed using hematoxylin and eosin staining. The 5 point scoring system described in our previous study was used to assess renal tubular damage [Citation18]. Pathologists performed blinded readings of the images of all the allocation groups.
Immunohistochemistry
For immunohistochemical staining, paraffin-embedded sections (5 μm) were dewaxed and rehydrated using gradient ethanol in water. The slides were incubated in citrate buffer (pH 6.0) to activate antigenicity using a microwave method and exposed to H2O2 solution for 15 min to block endogenous peroxidase. slides were then incubated with primary antibodies against KIM-1 (1:300), GRP78 (1:600), CHOP (1:100), and α-SMA (1:100) at 4 °C overnight, separately. After incubation with the corresponding secondary antibodies for 1 h at room temperature, the slides were stained with diaminobenzidine tetrahydrochloride (DAB), followed by counterstaining with hematoxylin to identify the nuclei. Images of the slide samples after immunohistochemical staining were obtained using a light microscope (Nikon ECLPSE 80i, Japan).
Immunofluorescence
For immunofluorescence staining, TCMK-1 cells were cultured in 24-well slides and fixed with 4% paraformaldehyde for 10 min. Slides were blocked with 5% BSA and 0.5% Triton X-100 for 2 h at room temperature and then incubated with primary antibody against GRP78 (1:200) at 4 °C overnight. After washing with PBS, the slides were incubated with the corresponding fluorescent secondary antibodies. DAPI was used to identify the nuclei. Images were captured using a fluorescence microscope (Nikon).
Western blot analysis
For the analysis of in vivo samples, total kidney tissues (containing both cortex and medulla) were homogenized, and total proteins were extracted using RIPA lysis buffer. For the analysis of in vitro samples, cultured TCMK-1 cells were collected and total proteins were extracted using cell lysis buffer. Protein concentrations were measured using a BCA protein assay kit (Beyotime Biotechnology, Shanghai, China). Equal amounts of total protein were separated by Sodium Dodecyl Sulfate Polyacrylamide gel electrophoresis (SDS-PAGE) and transferred to a polyvinylidene fluoride (PVDF) membrane. Primary antibodies against GRP78 (1:1000), CHOP (1:1000), and ATF4 (1:1000) were used to detect the degree of ER stress. Antibodies against GAPDH were used as loading controls. After reacting with the primary antibodies and horseradish peroxidase (HRP)-conjugated secondary antibodies, the protein bands were visualized using the ChemiDicTMXRS + Imaging System (Bio-Rad Laboratories).
Detection of ROS
ROS generated in TCMK-1 cells was measured using a Reactive Oxygen Species Assay Kit (Beyotime Biotechnology, Shanghai, China) according to the manufacturer’s protocol. In brief, TCMK-1 cells were incubated with DCFH-DA at 37 °C for 20 min and washed in PBS for three times. DAPI was co-stained to identify the nuclei. Images were captured using a fluorescence microscope (Nikon).
Determination of mitochondrial membrane potential (MMP)
The MMP of TCMK-1 cells was detected using a JC-1 Mitochondrial Membrane Potential Assay Kit (YEASEN, Shanghai, China) according to the manufacturer’s protocol. In brief, TCMK-1 cells were incubated with JC-1 solution at 37 °C for 20 min and then washed with 1 × JC-1 staining buffer. Images were captured using a fluorescence microscope (Nikon). The red-to-green fluorescence ratio was analyzed using ImageJ software.
Statistical analysis
Data from independent experiments are shown as the mean ± SEM Prism was applied for statistical analysis. Statistical significance was calculated by one-way analysis of variance (ANOVA). *p<0.05, considered statistically significant.
Results
COS alleviates I/R-induced renal dysfunction and histological damage
To study the protective effect of COS on acute kidney injury induced by ischemia-reperfusion, we investigated renal condition from the perspective of histopathology. H&E staining was used to assess changes in renal histological morphology two days after reperfusion (). No significant pathological damage was detected in the kidneys of rats in the sham group. However, the kidneys of the I/R group showed typical characteristics of AKI, such as swelling of renal tubular epithelial cells, intraluminal necrotic cellular debris, lumen obstruction, and formation of proteinaceous casts. In contrast, the administration of NAC strikingly attenuated renal pathological damage. N-acetylcysteine (NAC), an inhibitor of Reactive Oxygen Species (ROS), was used as a positive control in this study to demonstrate the protective effect of NAC on AKI. More importantly, COS treatment (both 100 mg/kg and 200 mg/kg) showed histological results similar to those of the NAC group, which both exhibited reversal of pathological changes caused by I/R injury. The renal tubular injury score of each group was then analyzed based on H&E staining to provide a clear and straightforward demonstration of the renal pathological changes (). It was striking that there was almost no statistical difference between the scores of the COS groups (both 100 mg/kg and 200 mg/kg) and the sham group, indicating that administration of COS could significantly attenuate the pathological damage of kidneys 2 days after reperfusion.
Figure 1. COS administration protects against AKI induced by renal I/R injury. (A) H&E staining was used to assess renal histological morphology at 2 days after reperfusion. Arrows show intraluminal necrotic cells. Scale bar represents 50 μm. (B) Tubular injury scores were quantified and analyzed based on H&E staining. Values are mean ± SEM, results are representative of five animals in each group. (C) Concentrations of serum Cr were measured at 2 days after reperfusion (mean ± SEM, n = 8). (D) Immunohistochemical staining of KIM-1. Scale bar represents 50 μm.
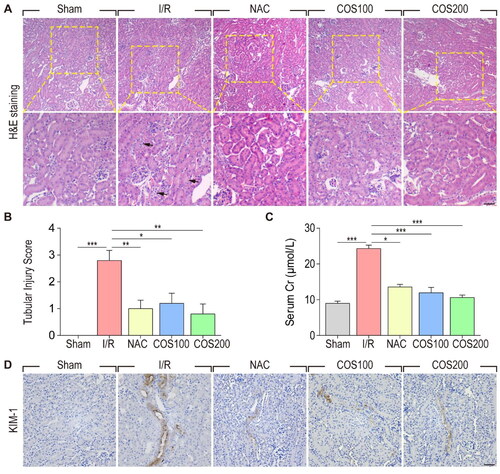
Furthermore, we measured serum Cr concentrations in each group 2 days after reperfusion to investigate the protective role of COS administration on renal function (). As expected, the concentrations of Cr were significantly increased in the I/R group compared to the sham group, whereas NAC injection remarkably reduced serum Cr concentrations after I/R injury. Importantly, COS treatment had an effect similar to that of NAC, indicating that COS could effectively attenuate I/R-induced renal dysfunction. To investigate the protective effect of COS administration on I/R-induced AKI, KIM-1 was detected by immunohistochemical staining. As a biomarker for renal proximal tubule injury, the number of KIM-1 positive tubular cells markedly increased in the kidneys of the I/R group. Nevertheless, administration of both NAC and COS remarkably reduced the number of KIM-1 positive tubular cells. Together, these results strongly demonstrated that oral administration of COS could effectively protect renal filtration function and maintain renal morphological integrity against I/R injury-induced AKI.
COS treatment inhibits maladaptive ER stress in vivo
Accumulating evidence suggests an interrelationship between ER stress and oxidative stress and their important roles in the pathogenesis of multiple human diseases [Citation19,Citation20]. Therefore, we examined whether the protective effect of COS against I/R injury was associated with the regulation of ER stress. Expression of GRP78 and CHOP was analyzed by immunohistochemical staining and western blotting using antibodies against GRP78 and CHOP, which are important ER stress effector proteins. As shown by immunohistochemical staining, the expression of GRP78 was dramatically increased in tubular epithelial cells after reperfusion, whereas NAC treatment reduced the level of expression compared to that in the I/R group. COS administration (both 100 mg/kg and 200 mg/kg) showed a similar effect as NAC treatment after renal I/R injury (). Likewise, results from immunohistochemical staining showed that COS administration also inhibited the expression of CHOP compared with the I/R group (). Western blotting made this same point a little more visible (). The expression of GRP78 and CHOP markedly increased after reperfusion, whereas COS administration significantly reduced the expression of GRP78 and CHOP. Similar results were obtained with qPCR analysis of CHOP mRNA, suggesting the inhibitory role of COS on ER stress ().
Figure 2. COS administration inhibits ER stress caused by I/R injury. (A) Immunohistochemical staining of GRP78. Scale bar represents 50 μm. (B) Immunohistochemical staining of CHOP. Scale bar represents 50 μm. (C) The expression of GRP78 and CHOP were analyzed with Western blotting at 2 days after reperfusion. β-tubulin was used as loading control (mean ± SEM, n = 8). (D) mRNA expression of CHOP relative to GAPDH (mean ± SEM, *p < 0.05, n = 8).
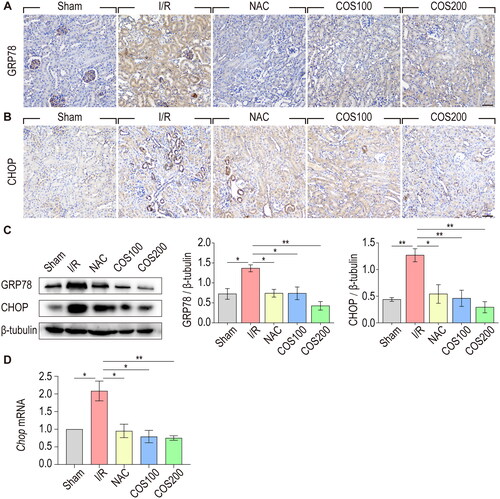
COS treatment inhibits maladaptive ER stress and mitochondrial oxidative damage in vitro
To further verify the relationship between the protective effect of COS and ER stress, we utilized tBHP-induced TCMK-1 cells as an oxidative stress damage cell model. Immunofluorescence staining showed that the expression of GRP78 in TCMK-1 cells significantly increased after TBHP treatment, whereas NAC or COS treatment effectively inhibited the production of GRP78 (). In addition, western blot analysis showed that GRP78, CHOP, and ATF-4, all of which are important ER stress effector proteins, were dramatically increased in TCMK-1 cells after TBHP treatment, whereas NAC and COS administration significantly reduced the production of these proteins (). Together, these results suggest that the protective effect of COS against renal I/R injury is associated with inhibition of maladaptive ER stress.
Figure 3. COS administration inhibits ER stress in TCMK-1 cells treated with TBHP. (A) Immunofluorescent staining of GRP78 in TCMK-1 cells. Scale bar represents 20 μm. (B) The expression of GRP78, CHOP and ATF-4 in TCMK-1 cells were analyzed with Western blotting. β-tubulin was used as loading control (mean ± SEM, n = 8).
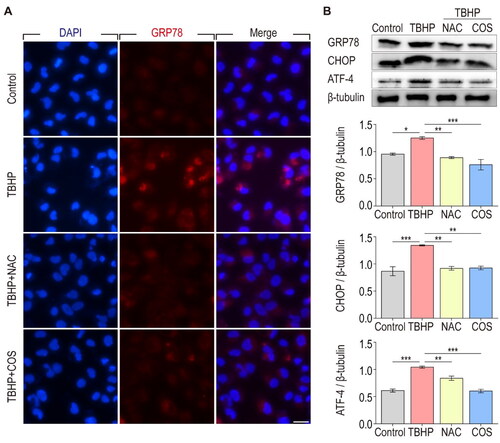
It is well documented that ER stress is related to oxidative stress, and these two factors play important roles in the pathogenesis of multiple human diseases, such as renal disease [Citation19,Citation20]. We then examined whether the protective effect of COS against I/R injury is associated with the regulation of oxidative stress. Our previous work demonstrated that excessive ROS production is implicated in the pathogenesis of oxidative stress and triggers mitochondrial damage [Citation3]. In vitro oxidative stress damage cell model revealed that TBHP treatment increased ROS production in TCMK-1 cells, whereas NAC and COS dramatically decreased the overproduction of ROS (). Next, we carried out JC-1 Assay to measure MMP (Mitochondrial Membrane Potential) in TCMK-1 cells. We found that the relative fluorescence intensity of red to green was much lower in TBHP-treated TCMK-1 cells than in the control TCMK-1 cells. However, the relative fluorescence intensity of red to green was found to be higher in TBHP + NAC group’s cells compared with it in TBHP group. Importantly, COS treatment showed a similar effect to NAC in maintaining the MMP of TCMK-1 cells (). These data strongly indicated that COS treatment could alleviate oxidative damage by reducing ROS overproduction and maintaining MMP.
Figure 4. COS administration alleviates oxidative damage of TCMK-1 cells induced by TBHP. (A) Fluorescence microscopy detection of ROS generation by DCFH-DA. Scale bar represents 50 μm. (B) ROS levels in TCMK-1 cells were analyzed (mean ± SEM, n = 8). (C) MMP was detected using a JC-1 MMP assay Kit and images was captured. (D) The red to green fluorescence ratio was analyzed by image J (mean ± SEM, n = 8).
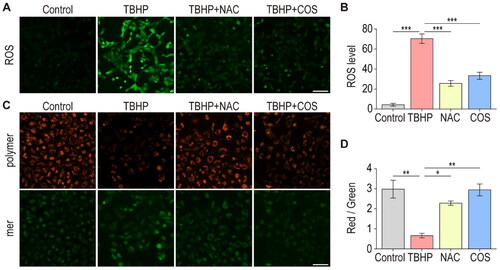
COS alleviates I/R induced renal interstitial fibrosis
To investigate whether COS administration could attenuate the transition from AKI to CKD, H&E staining was used to examine renal morphological changes in each group 2 months after reperfusion (). Large areas of scar tissue, circled with yellow dotted lines, were observed in the kidneys of the I/R group. Notably, COS administration showed a better protective effect on renal morphology than valsartan at 2 months after reperfusion. Valsartan is an angiotensin II receptor antagonist that serves as a first-line treatment for hypertension, particularly CKD. Masson staining was used to evaluate the effect of COS administration on renal interstitial fibrosis caused by I/R injury (). As expected, massive extra cellular matrix (ECM) deposition was observed around the renal tubules in the kidneys of the I/R group. In contrast, both valsartan and COS administration strikingly attenuated ECM deposition, as shown by Masson staining. To visualize such collagen deposition, we performed IHC analysis of Collagen I, the most abundant protein in the ECM. In sham group, Collagen I mainly located in blood vessel wall, whereas in I/R group, Collagen I were markedly induced, and found in tubular basement membrane. Intriguingly, in COS or valsartan group, Collagen I were almost not detectable in in tubular basement membrane. Similar results were obtained using qPCR analysis of Collagen I at mRNA level (). Further, we checked another ECM component—fibronectin. qPCR analysis showed that fibronectin was induced in I/R group, while COS treatment could block its expression significantly.
Figure 5. COS administration attenuates renal interstitial fibrosis at 2 months after renal I/R injury. (A) Renal morphology was examined with H&E staining. Areas bordered by yellow dotted line shows the pathological changes in kidney. Scale bar represents 50 μm. (B) Renal interstitial fibrosis was detected using Masson trichome staining. Arrows show extracellular matrix deposition around renal tubules. Scale bar represents 50 μm. (C) Immunohistochemical staining of α-SMA. Scale bar represents 50 μm. (D) IHC analysis of Collagen I in kidney tissues. Scale bar represents 50 μm. (E-F) mRNA expression of Collagen I and Fibronectin relative to GAPDH (mean ± SEM, *p < 0.05, n = 8).
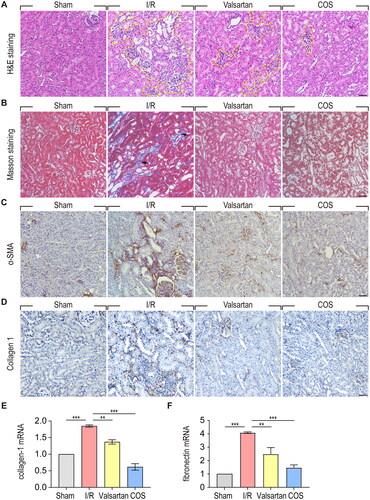
To further validate the protective effect of COS against renal interstitial fibrosis induced by I/R injury, we performed α-SMA immunohistochemical staining to examine myofibroblast proliferation (). In the kidneys of the sham group, α-SMA was mainly expressed in the smooth muscle cells of vessels. In the kidneys of the I/R group, α-SMA was significantly increased and was mainly located on the renal tubule basement membrane, which underwent atrophy. In contrast, COS and valsartan administration markedly inhibited myofibroblast proliferation. Taken together, these results indicate that COS administration could effectively attenuate the proliferation of myofibroblasts, mitigate ECM deposition, and thus inhibit the transition of AKI to CKD.
COS responsive genes/signaling pathways involve in TBHP-induced TCMK-1 cells injury
To obtain molecular insights into the protective effects of COS, we performed RNA-seq to investigate the related signaling pathways and important genes involved in this protective process. Compared to the control group, 746 genes were upregulated and 996 genes were downregulated in the TBHP group. However, after COS administration, 37 upregulated genes were reversed, and 13 downregulated genes were reversed significantly. Next, we performed gene ontology (GO) function analysis on the protein-coding differentially expressed genes (DEGs) that reversed renal injury after COS protection. As shown by the GO analysis histogram, genes Pam16, Dhrs13, and Sdhaf4 related to mitochondria, and genes Aldh1a1, Entpd4 related to apoptosis and metabolism, were found to be possible genes involved in COS regulation.
In order to further clarify the protective mechanism of COS against I/R-induced kidney injury, we used qPCR to validate these identified DEGs in TBHP-induced TCMK-1 cells. These genes verified above, such as Pam16, Trem2 and Esrra, were significantly upregulated in TBHP group and downregulated in COS group, which was consistent with RNA-Seq data (). Taken together, we concluded that COS may play a role in renal protection by participating in metabolic and redox biological processes.
Figure 6. RNA-seq analysis Identifies gene expression profile regulated by COS. (A) Heatmap shows differential gene expression profiles, with upregulation in red, downregulation in blue. (B) Gene ontology (GO) function analysis histogram. BP is marked by dark cyan, CC is marked by sienna and MF is marked by steel blue. The bar chart was constructed through the bioinformatics platform. (C) mRNA expression of Pam16, Trem2 and Esrra relative to GAPDH (mean ± SEM, *p < 0.05, n = 8).
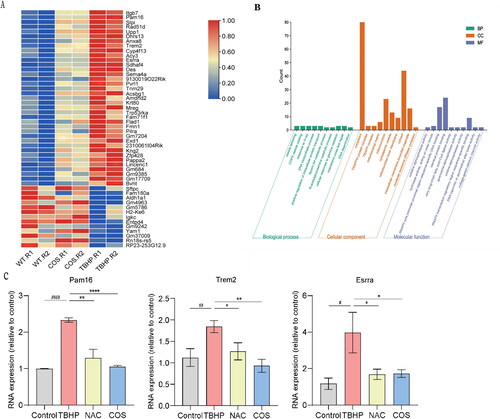
Discussion
Although great progress has been made in the development of therapeutic approaches to treat AKI, it is essential to look for the prevention of health substances with good curative effects and without any adverse side effects. Moreover, the large number of people suffering from severe renal diseases makes it necessary to develop effective drugs to treat or prevent renal diseases. Here, we demonstrated the renoprotective effects of COS via both in vivo and in vitro experiments. COS administered by oral gavage can significantly alleviate renal tubular injury and maintain glomerular filtration. This protective effect of COS is associated with inhibition of oxidative stress, mitochondrial damage, and ER stress. Moreover, this study also shows that long-term administration of COS can attenuate the proliferation of myofibroblasts, mitigate ECM deposition, and thus alleviate renal interstitial fibrosis induced by renal I/R injury.
The endoplasmic reticulum (ER) is an organelle with functions including protein synthesis, folding, maturation and assembly [Citation21]. Protein folding is oxygen-dependent; therefore, hypoxia-ischemia can trigger ER stress, a maladaptive unfolded protein response (UPR) pathway, which plays a key role in determining the fate of damaged cells [Citation22,Citation23], and thus may participate in the pathogenesis of multiple human diseases. To clarify whether the protective effect of COS treatment was associated with ER stress, we measured the expression of ER stress effector proteins. Our results from both in vivo and in vitro experiments revealed that the renoprotective effect of COS is associated with the alleviation of excessive ER stress.
Recently, an increasing number of studies on kidney diseases have reported a relationship between oxidative stress, mitochondrial dysfunction, and ER stress [Citation24–26]. Reperfusion of blood flow can exacerbate the overproduction of ROS in the mitochondria, which can aggravate mitochondrial damage and cellular oxidative stress [Citation2,Citation3]. The oxidative stress caused by ROS overproduction can exacerbate ER stress, which can produce more ROS, resulting in the aggravation of oxidative stress damage and mitochondrial dysfunction. These mechanisms play key roles in the pathogenesis of multiple human diseases. MMP plays crucial roles in the maintenance of mitochondrial oxidative phosphorylation and production of adenosine triphosphate (ATP). Therefore, ROS generation and MMP decline can be considered early indicators of I/R injury [Citation27]. To determine whether the protective effect of COS is associated with alleviating mitochondrial damage and reducing ROS generation, we measured the production of ROS and MMP in oxidatively damaged TCMK-1 cells. Our results demonstrated that COS treatment could reduce the overproduction of ROS and thus protect the mitochondria against oxidative damage.
Previous studies have reported that oxidative stress plays a crucial role in the development of renal interstitial fibrosis after I/R injury [Citation28]. ER stress is involved in the development of renal fibrosis [Citation29]. The organelle crosstalk between the mitochondria and the ER can serve as a potential target for the treatment of renal I/R injury. To determine whether COS treatment could inhibit the transition from AKI to CKD, we examined renal histological morphology two months after I/R injury. It was found that long-term administration of COS by oral gavage strikingly alleviated the development of renal interstitial fibrosis caused by I/R injury by attenuating myofibroblast proliferation and mitigating ECM deposition. This study verified the antioxidant properties of COS and suggested its therapeutic potential for the treatment of I/R-induced AKI and CKD development.
Conclusions
In conclusion, our data indicate that COS can attenuate acute kidney injury and renal interstitial fibrosis induced by ischemia-reperfusion, thereby enhancing renal function. It offers a new perspective for the prevention and treatment of acute kidney injury, and provides novel insights into the protective role of COS in renal diseases.
Ethical approval
Our study has been approved by the Ethics Committee of the Affiliated Hospital of Qingdao University (Ethical approval no. QYFY WZLL27937).
Acknowledgments
The authors thank the participants and staff of this study for their time and commitment.
Disclosure statement
No potential conflict of interest was reported by the authors.
Data availability statement
The data that support the findings of this study are available upon request from the corresponding author.
Additional information
Funding
References
- Kellum JA, Romagnani P, Ashuntantang G, et al. Acute kidney injury. Nat Rev Dis Primers. 2021;7(1):1. doi: 10.1038/s41572-021-00284-z.
- Eltzschig HK, Eckle T. Ischemia and reperfusion–from mechanism to translation. Nat Med. 2011;17(11):1391–11. doi: 10.1038/nm.2507.
- Tan X, Zhang L, Jiang Y, et al. Postconditioning ameliorates mitochondrial DNA damage and deletion after renal ischemic injury. Nephrol Dial Transplant. 2013;28(11):2754–2765. doi: 10.1093/ndt/gft278.
- Chawla LS, Eggers PW, Star RA, et al. Acute kidney injury and chronic kidney disease as interconnected syndromes. N Engl J Med. 2014;371(1):58–66. doi: 10.1056/NEJMra1214243.
- Ishani A, Xue JL, Himmelfarb J, et al. Acute kidney injury increases risk of ESRD among elderly. J Am Soc Nephrol. 2009;20(1):223–228. doi: 10.1681/ASN.2007080837.
- Naveed M, Phil L, Sohail M, et al. Chitosan oligosaccharide (COS): an overview. Int J Biol Macromol. 2019;129:827–843. doi: 10.1016/j.ijbiomac.2019.01.192.
- Muanprasat C, Chatsudthipong V. Chitosan oligosaccharide: biological activities and potential therapeutic applications. Pharmacol Ther. 2017;170:80–97. doi: 10.1016/j.pharmthera.2016.10.013.
- Deng X, Ye Z, Cao H, et al. Chitosan oligosaccharide ameliorated obesity by reducing endoplasmic reticulum stress in diet-induced obese rats. Food Funct. 2020;11(7):6285–6296. doi: 10.1039/d0fo01107j.
- Zheng J, Yuan X, Cheng G, et al. Chitosan oligosaccharides improve the disturbance in glucose metabolism and reverse the dysbiosis of gut microbiota in diabetic mice. Carbohydr Polym. 2018;190:77–86. doi: 10.1016/j.carbpol.2018.02.058.
- Jia S, Lu Z, Gao Z, et al. Chitosan oligosaccharides alleviate cognitive deficits in an amyloid-beta1-42-induced rat model of alzheimer’s disease. Int J Biol Macromol. 2016;83:416–425. doi: 10.1016/j.ijbiomac.2015.11.011.
- Qian M, et al. Chitosan oligosaccharide ameliorates nonalcoholic fatty liver disease (NAFLD) in Diet-Induced obese mice. Mar Drugs. 2019;17(7):391–404. doi: 10.3390/md17070391.
- Sutthasupha P, Promsan S, Thongnak L, et al. Chitosan oligosaccharide mitigates kidney injury in prediabetic rats by improving intestinal barrier and renal autophagy. Carbohydr Polym. 2022;288:119405. doi: 10.1016/j.carbpol.2022.119405.
- Wu J, Xu Y, Geng Z, et al. Chitosan oligosaccharide alleviates renal fibrosis through reducing oxidative stress damage and regulating TGF-beta1/smads pathway. Sci Rep. 2022;12(1):19160. doi: 10.1038/s41598-022-20719-1.
- Yoon HJ, Moon ME, Park HS, et al. Effects of chitosan oligosaccharide (COS) on the glycerol-induced acute renal failure in vitro and in vivo. Food Chem Toxicol. 2008;46(2):710–716. doi: 10.1016/j.fct.2007.09.111.
- Yoon SP, Han MS, Kim JW, et al. Protective effects of chitosan oligosaccharide on paraquat-induced nephrotoxicity in rats. Food Chem Toxicol. 2011;49(8):1828–1833. doi: 10.1016/j.fct.2011.04.036.
- Wang D-W, Li S-J, Tan X-Y, et al. Engineering of stepwise-targeting chitosan oligosaccharide conjugate for the treatment of acute kidney injury. Carbohydr Polym. 2021;256:117556. doi: 10.1016/j.carbpol.2020.117556.
- Zhang P, Liu W, Peng Y, et al. Toll like receptor 4 (TLR4) mediates the stimulating activities of chitosan oligosaccharide on macrophages. Int Immunopharmacol. 2014;23(1):254–261. doi: 10.1016/j.intimp.2014.09.007.
- Tan X, Yu L, Yang R, et al. Fibroblast growth factor 10 attenuates renal damage by regulating endoplasmic reticulum stress after Ischemia-Reperfusion injury. Front Pharmacol. 2020;11:39. doi: 10.3389/fphar.2020.00039.
- Zeeshan HMA, Lee GH, Kim H-R, et al. Endoplasmic reticulum stress and associated ROS. Int J Mol Sci. 2016;17(3):327. doi: 10.3390/ijms17030327.
- Malhotra JD, Kaufman RJ. Endoplasmic reticulum stress and oxidative stress: a vicious cycle or a double-edged sword? Antioxid Redox Signal. 2007;9(12):2277–2293. doi: 10.1089/ars.2007.1782.
- Walter P, Ron D. The unfolded protein response: from stress pathway to homeostatic regulation. Science. 2011;334(6059):1081–1086. doi: 10.1126/science.1209038.
- Yan M, Shu S, Guo C, et al. Endoplasmic reticulum stress in ischemic and nephrotoxic acute kidney injury. Ann Med. 2018;50(5):381–390. doi: 10.1080/07853890.2018.1489142.
- Cybulsky AV. Endoplasmic reticulum stress, the unfolded protein response and autophagy in kidney diseases. Nat Rev Nephrol. 2017;13(11):681–696. doi: 10.1038/nrneph.2017.129.
- Maekawa H, Inagi R. Pathophysiological role of organelle stress/crosstalk in AKI-to-CKD transition. Semin Nephrol. 2019;39(6):581–588. doi: 10.1016/j.semnephrol.2019.10.007.
- Jiang X-S, Xiang X-Y, Chen X-M, et al. Inhibition of soluble epoxide hydrolase attenuates renal tubular mitochondrial dysfunction and ER stress by restoring autophagic flux in diabetic nephropathy. Cell Death Dis. 2020;11(5):385. doi: 10.1038/s41419-020-2594-x.
- Liu X, Zhang R, Huang L, et al. Excessive oxidative stress contributes to increased acute ER stress kidney injury in aged mice. Oxid Med Cell Longev. 2019;2019:2746521. doi: 10.1155/2019/2746521.
- Quarrie R, Lee DS, Steinbaugh G, et al. Ischemic preconditioning preserves mitochondrial membrane potential and limits reactive oxygen species production. J Surg Res. 2012;178(1):8–17. doi: 10.1016/j.jss.2012.05.090.
- Zhang S, Tan X, Chen Y, et al. Postconditioning protects renal fibrosis by attenuating oxidative stress-induced mitochondrial injury. Nephrol Dial Transplant. 2017;32(10):1628–1636. doi: 10.1093/ndt/gfw469.
- Chiang C-K, Hsu S-P, Wu C-T, et al. Endoplasmic reticulum stress implicated in the development of renal fibrosis. Mol Med. 2011;17(11–12):1295–1305. doi: 10.2119/molmed.2011.00131.