Abstract
Nuclear facility discharge pipelines accumulate inorganic and microbial fouling and radioactive contamination, however, research investigating the mechanisms that lead to their accumulation is limited. Using the Sellafield discharge pipeline as a model system, this study utilised modified Robbins devices to investigate the potential interplay between inorganic and biological processes in supporting fouling formation and radionuclide uptake. Initial experiments showed polyelectrolytes (present in pipeline effluents), had minimal effects on fouling formation. Biofilms were, however, found to be the key component promoting fouling, leading to increased uptake of inorganic particulates and metal contaminants (Cs, Sr, Co, Eu and Ru) compared to a non-biofilm control system. Biologically-mediated uptake mechanisms were implicated in Co and Ru accumulation, with a potential bioreduced Ru species identified on the biofilm system. This research emphasised the key role of biofilms in promoting fouling in discharge pipelines, advocating for the use of biocide treatments methods.
Graphical Abstract
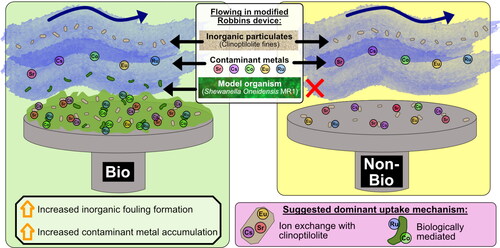
1. Introduction
Fouling can be defined as the accumulation of unwanted deposits at a surface-solution interface. Major fouling phenomena include scaling, where chemical compounds are precipitated onto surfaces and biofouling, where biofilms build up on submerged surfaces. In industrial settings, fouling and scaling are known to accumulate within equipment including pipes, boilers, and cooling towers. This fouling phenomenon can cause pipe blockages, reduction of heat-transfer energy, and enhanced corrosion, resulting in increased production and maintenance costs (Barton et al. Citation2022). Many industries are affected by fouling including oil and gas (Olajire Citation2015), drinking water distribution systems (Liu et al. Citation2016), reverse osmosis desalination (Supekar et al. Citation2018), geothermal systems (Gallup and von Hirtz Citation2015), and nuclear power (Rao et al. Citation2009). It has been predicted that annual expenses caused by fouling amount to 0.8 billion $US in the UK, 3 billion $US in Japan, and 9 billion $US in the US (Macadam and Parsons Citation2004; Li et al. Citation2017). The nuclear industry is afflicted with both inorganic and biological fouling issues. Magnetite fouling of steam generators (Prusek et al. Citation2017; Turner and Khumsa-Ang Citation2017) and biofouling of condenser cooling water systems (Satpathy et al. Citation2010; George et al. Citation2016) are known to cause reductions in overall nuclear power plant efficiency. Fouling induced incidents, including pipe blockages and damage caused by microbiologically influenced corrosion or stress corrosion cracking (Rao and Nair Citation1998; Terachi et al. Citation2005; Rao et al. Citation2009), are implicated in large economic losses. In some nuclear environments, added complexity is experienced where radioactivity is present, for example, biofouling in spent nuclear fuel (SNF) storage ponds can accumulate fission product radionuclides (Sarró et al. Citation2005; Tišáková et al. Citation2013; Foster et al. Citation2020b). Similarly, in primary circuitcoolant systems, 60Co accumulates within corrosion deposit fouling and is responsible for up to 80% of the exposure experienced by reactor operating personnel (Varga et al. Citation2001; Lin Citation2009).
Another key area of radiologically contaminated fouling at nuclear power plants is within the radioactive discharge pipelines (Magnox North Citation2008; Jones Citation2016), used for the authorised discharge of treated, very low level radioactive effluent. In the UK alone there is a substantial legacy of these contaminated pipelines. The pipelines are usually hard to access, running underground over long distances and have long operational timescales, often longer than 50 years. Sellafield is a prominent example, whereby pressure build up in a radioactive discharge pipeline led to the detection of considerable fouling deposits along the length of the underground pipeline (Jones Citation2016). The fouling had accumulated significant levels of radioactivity, with radionuclides detected including 106Ru, 90Sr, 125Sb, 137Cs, 134Cs, 60Co, 144Ce, 154Eu, and 155Eu in order of ascending activity (Bq/g). Challenges associated with these pipelines carry substantial economic ramifications, as the costs related to addressing issues such as maintenance and treatment of the radioactive fouling, the eventual decommissioning of the pipeline and the prevention of future pipeline fouling accumulation and radioactive contamination run into the millions of pounds. An understanding of the mechanism of pipe fouling formation, as well as how the radionuclide contaminants are being sequestered, is important to develop innovative solutions to these challenges.
The studied Sellafield discharge pipeline receives effluents from the Site Ion Exchange Plant (SIXEP), which is used for treatment of the large volumes of neutral to alkaline effluents produced from purges of the network of spent nuclear fuel ponds on site. The main components of SIXEP include a sand bed filter, dosed with a flocculent polyelectrolyte (Magnafloc LT31, 50% Aliphatic polyamine), to remove Mg-rich solids from the ponds with associated radionuclide contaminants, followed by a carbonation tower to reduce the overall alkaline pH to neutral. After this neutralisation, the effluent is treated by the ion exchange beds, made up of the zeolite clinoptilolite (Na6Al6Si30O72·24H2O), for the removal of 137Cs and 90Sr (Owens et al. Citation2015). It is highly likely that minor fractions of the components from SIXEP, such as clinoptilolite fines and polyelectrolyte, will be present in the pipeline effluent. A recovered sample of the Sellafield pipe fouling deposit was analysed with energy-dispersive spectroscopy (EDX) (Jones Citation2016), which determined it to have a similar composition to clinoptilolite (see Figure S1 for images of the solid contamination and clinoptilolite). Several potential mechanisms of Sellafield pipe fouling formation have been considered, including (i) biofilm formation and the entrapment of clinoptilolite fines (Vieira and Melo Citation1995; Nexia Solutions Citation2006), (ii) polyelectrolyte mediated attachment of clinoptilolite fines (Claesson et al. Citation2005; Jones Citation2016), and (iii) chemical precipitation onto the pipe surface (British Nuclear Group Citation2005; Muryanto et al. Citation2014). There are multiple lines of evidence that could support a role for biofilm formation in contributing to the Sellafield pipe fouling. Notably, there is availability of carbon sources for microbial growth, for example, biomass accumulated in microbial blooms that develop in the upstream SNF ponds (Foster et al. Citation2020a). The effluent contains glycol and polyelectrolyte (BNFL. Citation2004; Owens et al. Citation2015; Jones Citation2016), providing additional carbon sources. The fouling deposit was also found to have a high phosphorus content (Jones Citation2016), which is not typically seen in the effluent and hence could be of biological origin. The solid was also determined to have a porous structure and high water content (Jones Citation2016), both properties being consistent with biofilms (Zhang and Bishop Citation1994; Sandt et al. Citation2008). Biofilms are also likely to be present due to their enhanced ability to survive in extreme conditions including the radiation present in the pipe fouling (Yin et al. Citation2019).
Gaining access to these underground pipelines for collecting samples or manual cleaning is challenging both due to the radiation in the pipeline and as there are limited access points. Subsequently, laboratory investigations with replicated systems are an essential bridge to better understand the mechanisms of fouling formation and treatment. This is the first study building these approaches to further understand the combined role of abiotic and biological processes in pipeline fouling in the Sellafield radioactive effluent systems. The research described here has used Modified Robbins Devices (MRDs), dynamic systems typically used for growing biofilms (Kharazmi et al. Citation1999), as model systems for radioactive discharge pipelines. The MRDs were used in combination with geochemical, mineralogical, surface science, and biological imaging techniques to investigate Sellafield pipeline fouling formation mechanisms (both biological and inorganic), and radionuclide incorporation. Our results showed that biofilm formation enhanced pipe fouling formation and led to the capture of solids (clinoptilolite fines) onto the metal surface used to replicate pipelines. Thus, biofilms were determined to be a key component underpinning fouling formation. The biofouling was also associated with increased accumulation of metal contaminants from solution, likely via biological uptake mechanisms. The significance of biofilms identified in these radioactive discharge pipe fouling model systems is novel and could inform discharge pipeline management and decommissioning strategies, as well as mitigation strategies in new nuclear power plants.
2. Materials and methods
2.1. Batch boundary condition experiments
Samples of the polyelectrolyte (Magnafloc LT31) and clinoptilolite (sourced from the Mud Hills deposit, California, USA) were provided by the National Nuclear Laboratory as products representative of those used on the Sellafield site. Continual washing of the clinoptilolite in SIXEP is likely to produce clinoptilolite fines which would be present in the pipeline effluent. Consequently, the clinoptilolite in the pipe fouling is most likely in the form of fines. Fines can be obtained by labour intensive washing of clinoptilolite grains, however, the easier experimental method of powdering clinoptilolite grains was found, via scanning electron microscopy (SEM), to produce particles of the same size range as the fines (≈10-50 µm), as shown in Figure S2. Thus powdered clinoptilolite was used for these experiments. Powdered clinoptilolite (40 mg) was added to three concentrations (1, 0.1, and 0% v/v) of the Magnafloc LT31 polyelectrolyte in deionised water (DI) water (200 mL). A steel coupon of the same grade as the Sellafield discharge pipeline (UNS S30403) was suspended in the solution from the lid of the bottle and the solutions were left to stir for 2 weeks. The coupons were removed and dried at 40 °C, and the attachment of clinoptilolite to the coupons was quantified using SEM-EDX mapping.
2.2. Microbial culturing
Shewanella oneidensis MR-1 was obtained from the University of Manchester Geomicrobiology Group culture collection. Starter cultures were grown aerobically in tryptic soy broth (Oxoid CM0876) (0.9 g in 30 mL of deionised water (DIW) (ultrapure water, Millipore MQ; 18.2 MΩ cm)) for 4 h (30 °C, 100 rpm) and then transferred into an anoxic minimal medium (Myers and Nealson Citation1988) containing lactate (10 mM) as the electron donor and fumarate (10 mM) as the electron acceptor. The bacteria were grown to mid-exponential phase for 24 hrs incubation at 30 0C, then centrifuged (5000 g, 20 mins) and added to 40 mL of minimal medium to give a final optical density (OD) of 0.2, prior to addition to a Modified Robbins Device (MRD).
2.3. Modified Robbins Device setup
Four bespoke MRDs were built for this research. Each MRD comprised a 36 cm long acrylic block with a channel through the middle of 1.8 by 22.5 cm, and with 5 evenly spaced sample ports with screwed-in sample plugs containing aseptically replaceable coupons of UNS S30403 stainless steel (Figure S3). Flow rate into the devices was controlled with a peristaltic pump (Masterflex L/S® Easy-Load®) II (Cole-Parmer®). Before each experiment the entire experimental apparatus including coupons, tubing, and the MRD, was sterilised with 10% sodium hypochlorite followed by 98% ethanol and then washed with DIW.
2.4. Modified Robbins Device experiments
The MRD used for biofilm growth, post sterilisation and washing, was inoculated with 40 mL (0.2 OD) of late exponential/early stationary phase S. oneidensis culture, grown anaerobically in minimal medium (as detail in section 2.2). S. oneidensis can growth both aerobically and anaerobically but an anaerobic culture was chosen for inoculation in these experiments because the pipe fouling system would likely become anoxic, especially in any biofouling. The culture was pumped around the device in a circulating system (Figure S4) for 8 h. A 33 ml/h flow rate was maintained throughout the experiment. It was then switched to a flow-through system (Figure S4) and minimal medium was pumped through the system for 2 days for biofilm maturation, then a coupon was extracted to analyse with fluorescence microscopy. A solution of clinoptilolite fines in minimal media (0.5 g/L) was then pumped through the biofilm MRD for 2 days in a flow through system. At the same time, after sterilisation and washing, a solution of 0.5 g/L clinoptilolite fines in DIW was pumped through the non-biofilm MRD for 2 days in a flow through system. The coupons were then removed for SEM analysis.
In further experiments, the previously described procedure was repeated but after 2 days of delivery of a clinoptilolite fines solution (0.5 g/L), 200 mL solutions containing SrCl2·6H2O, CsCl, CoCl2·6H2O, EuCl3.6H2O, and RuCl3·xH2O (all 100 μM), in 3-(N-morpholino)propanesulfonic acid (MOPS, 50 mM) with lactate (10 mM), were circulated in the biofilm and non-biofilm MRDs for 8 days (as surrogates for contaminating radionuclides). MOPS was identified as the best buffer for solubilising all metal species and lactate was added as an electron donor to sustain the S. oneidensis growth over the 8 days. Aliquots were taken daily for inductively coupled plasma mass spectrometry (ICP-MS) measurements, and coupons removed for SEM, laser ablation – (ICP-MS) and X-ray photoelectron spectroscopy (XPS) analysis.
2.5. SEM-EDX mapping
After removal of the coupons from the MRD, biofilms were fixed in 2.5% v/v glutaraldehyde in phosphate buffered saline (PBS) overnight, and then washed in PBS diluted to 60% and 30%. The coupons were subsequently dehydrated in 30 min steps of DIW with increasing ethanol concentrations of 25, 30, 40, 50, 60, 70, 80, 90 and 100%. The non-biofilm coupons were dried in air and both the biofilm and non-biofilm coupons were coated with gold and examined via scanning electron microscopy. A FEI XL30 field emission gun (FEG) - environmental SEM instrument equipped with an EDAX Gemini EDX system, was used for imaging and chemical analysis using a 15 kV accelerating voltage in high vacuum mode.
2.6. Fluorescence microscopy
The coupons were rinsed with DIW then stained with the LIVE/DEADTM BacLightTM Bacterial Viability Kit (L-7012) (Molecular Probes, Eugene, Ore.), which is composed of SYTO 9 as a viability marker and propidium iodine (PI) as a membrane-compromised dead cell marker. Each coupon was covered with a 1:1 staining solution of SYTO 9 and PI in DIW. The coupons were incubated for 15 min in the dark at room temperature and were then washed with DIW. The samples were examined by epifluorescence microscopy with a magnification of 100 times immersion oil objective, using 100 times immersion oil [Immersol 518 N, ne = 1.518 (23 °C)] on a Zeiss Axio Imager A1 (Carl Zeiss Microimaging 234 GmbH, Germany) light microscope equip with an Axiocam 506 mono camera with Zen2 imaging software. The filter sets used included 09 (SYTO9™) and 26 (propidium iodide).
2.7. ICP-MS and LA-ICP-MS
The concentrations of Cs, Sr, Co, Ru, and Eu in the MRD circulating solutions were determined by inductively coupled plasma mass spectrometry (ICP − MS) analysis of acidified (2% HNO3) aliquots using an Agilent 7500CX ICP − MS.
The concentrations of Cs, Sr, Co, Ru, Eu, Si, and Al on the coupon surfaces were also determined with laser-ablation inductively-coupled plasma mass spectrometry (LA-ICP-MS), using a Teledyne Photon Machines Analyte Excite+ 193 nm ArF Excimer laser ablation system equipped with a HelEx II active 2‐volume ablation cell, coupled to an Agilent 8900 ICP‐MS (see Table S2 for a summary of the analytical setup and data processing procedure). Ablation of NIST 612 glass was used to tune the instrument, optimising signal intensities while maintaining low levels of oxide formation (232ThO/232Th < 0.2%) and U/Th ratio close to unity. Fifteen analyses were carried out on each coupon, using a spot size of 50 μm, a fluence of 5 J.cm−2, and a repetition rate of 5 Hz. The spots were chosen at random to avoid bias. Each analysis lasted 40 s and was preceded by 20 s counting time of the gas blank (background). The masses analysed comprised 13C, 27Al, 28Si, 29Si, 31P, 52Cr, 57Fe, 59Co, 88Sr, 101Ru, 133Cs, and 153Eu (see Table S2 for dwell times used). Signal intensities were corrected from background contributions by subtracting the gas blank. The semi-quantitative Trace Elements data reduction scheme of the Iolite 4 software (Woodhead et al. Citation2007; Paton et al. Citation2011) was used for data reduction. Coupon analyses were bracketed by analysing reference glass NIST 612, reference metals NIST SRM 661 and 663, and chips of the iron meteorite Hoba every 15 unknown analysis. NIST SRM 663 was used as primary reference material for 13C, 27Al, 28Si, 29Si, 31P, 52Cr, 57Fe, and 59Co, NIST 612 for 88Sr, 133Cs, and 153Eu, and Hoba for 101Ru. 13C was used instead of 12C to avoid saturating the detector with elevated intensity of 12C. All data obtained on the coupons are given in Table S3, together with their associated 2 standard error uncertainties.
Maps were also acquired to image the distribution of the elements listed above at the surface of the coupons. A 2 μm rectangular spot (with 5 J.cm−2 fluence and 5 Hz repetition rate) was rastered at 2 μm.s−1 for 40 s to produce 80 μm long lines. Stacks of 20 successive horizontal lines were acquired on each samples to produce 80 × 40 μm maps. All element intensities were normalised to the 52Cr intensity (Figure S6).
2.8. XPS
X-ray photoelectron spectroscopy (XPS) measurements of the coupon surfaces were performed using a Kratos Axis Ultra DLD spectrometer with a monochromated Al Kα X-ray source (hv = 1486.6 eV, 10 mA emission at 150 W). A low-energy electron source charge neutraliser was used to remove any differential charging effects under the X-rays, and Binding Energy scale calibration was performed using C − C in the C 1s photoelectron peak at 284.8 eV. Quantified analysis and chemical state identification via peak fitting were performed using the CasaXPS software (www.casaxps.com).
3. Results and discussion
3.1. Influence of polyelectrolyte on clinoptilolite attachment
Clinoptilolite fines and polyelectrolyte will be present in key Sellafield effluents after release from SIXEP (Jones Citation2016). Batch coupon experiments were used to test the hypothesis that the polyelectrolyte flocculation properties (Claesson et al. Citation2005) could facilitate the aggregation and attachment of clinoptilolite fines to the pipeline surface, promoting fouling formation. SEM imaging showed that the clinoptilolite attached to the coupon surfaces at all concentrations of polyelectrolyte (). Furthermore, image EDX mapping analysis of the coupons from 0 and 0.1% v/v concentration of polyelectrolyte gave a very similar clinoptilolite surface area coverage of approximately 20%, however, when the v/v of polyelectrolyte was increased to 1%, the clinoptilolite coverage dropped by more than half to approximately 8% of the coupon surface area (). Thus, these simple batch experiments suggest that increasing polyelectrolyte concentration apparently decreases clinoptilolite fines attachment to a stainless steel surface, suggesting that polyelectrolyte is unlikely to play a large role in the pipe fouling formation. On that basis it was not considered further in this study.
Figure 1. Black and white stitched BSE images (left) of the whole coupon within the red area, clinoptilolite (black) coverage of the steel (white) was quantified using imageJ to give the percentage (below the image). EDX maps (right) from 4 randomly allocated spots on the coupons, grouped the clinoptilolite (green) and steel (pink) phases separately for quantification of clinoptilolite coverage by % surface area (below image).
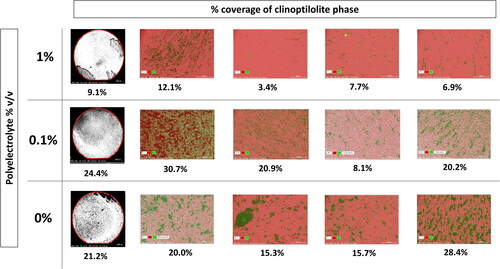
Table 1. Percentages of clinoptilolite attached to stainless steel coupons based on 4 random areas (1-4) and the whole coupon, in solutions of different concentrations of polyelectrolyte in deionised water. Percentages determined via EDX mapping of the phases and ImageJ processing of black and white SEM BSE images of the whole coupon.
3.2. The impact of biofilm on inorganic fouling formation
To investigate the potential role and impact of biofouling in discharge pipelines, MRDs were used to grow bacterial biofilms (Kharazmi et al. Citation1999). A comparison could then be made to non-biofilm MRD systems on the ability to accumulate clinoptilolite fines and metals/radionuclides. S. oneidensis was used as a model organism for biofilm growth because it is a metabolically flexible bacterium, capable of both biofilm formation and a range of interactions with metals (Lloyd Citation2003; Lloyd and Renshaw Citation2005; Sani et al. Citation2008; Zhang et al. Citation2014).
Epifluorescence microscopy was used to confirm the presence of a mature biofilm on the biofilm MRD coupons (). Visual inspection of coupons that had supported biofilm growth in the MRD showed that they clearly attached more clinoptilolite compared to the non-biofilm coated coupon ( and , respectively). This observation was corroborated in the respective BSE images () where the clinoptilolite is visible as darker deposits (due to higher abundances of low-Z elements Si, Al, and O), compared to the steel (Fe) background. The biofilm coupon () had an almost complete coverage of clinoptilolite whereas the non-bio coupon () had limited clinoptilolite coverage. Further investigation of the biofilm coupon via SEM and EDX mapping was used to distinguish the different components of the system via their elements as shown in (another example in Figure S5). Cells within the biofilm could be identified by their organic carbon (), the clinoptilolite by the silicon () and the steel by the iron present (). The microbial biomass and clinoptilolite appeared to be co-located, suggesting that the biofilm entrapped the clinoptilolite fines (also shown in the Figure S5 example). This in turn offers an explanation for the increased coverage of clinoptilolite on the biofilm coupon over the non-biofilm coupon. Biofilms have been found to incorporate suspended solids adding to their mechanical strength and biomass accumulation (Vieira and Melo Citation1995). The enhanced ability displayed here by biofilms to accumulate inorganic particulate in a flowing system suggests the importance of biofilms in supporting the formation of fouling deposits in nuclear discharge pipelines.
Figure 2. Epifluorescence microscopy micrographs of one biofilm coupon with LIVE/DEADTM BacLightTM staining, with living bacteria in green and dead bacteria in red. The coupon was extracted after 3 days, after inoculation and biofilm maturation and prior to exposure to clinoptilolite fines or metals in solution.
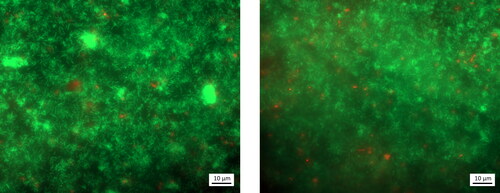
Figure 3. Images of coupons removed from the biofilm (a) and non-biofilm MRD (b) after exposure to clinoptilolite fines (without exposure to any metal solutions), and corresponding SEM BSE images of a biofilm (c) and non-biofilm coupon (d), on which steel is seen as the lighter grey and clinoptilolite as the darker grey.
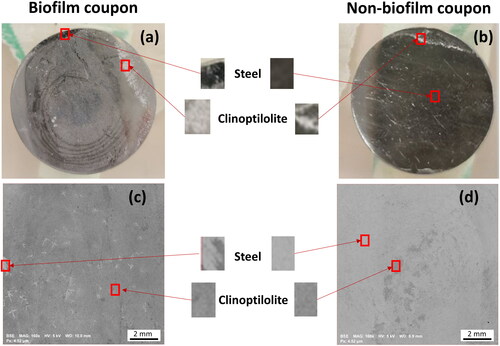
3.3. Investigating uptake of metal contaminants to pipe fouling
The biofilm- and clinoptilolite-replicated fouling systems, created with the MRDs in section 3.2, were used to investigate the accumulation and speciation of common metal contaminants that would be present in the Sellafield effluents. Solution ICP-MS analysis showed that the uptake of Cs and Sr from solution was similar, with similar removal rates, in the biofilm and non-biofilm MRD systems (). For the biofilm MRD, the concentrations in solution were reduced by 93% for Cs and 70% for Sr, while for the non-biofilm MRD, concentrations in solution dropped by 94% and 78%, respectively (Table S1). The primary uptake mechanism for Cs and Sr is likely to be ion exchange in the clinoptilolite (Dyer et al. Citation2006), as clinoptilolite is used specifically in the SIXEP plant for its ability to selectively remove 137Cs and 90Sr from effluents purged from SNF ponds (Owens et al. Citation2015). The Cs(I) and Sr(II) cations bind to oxygen atoms of the 3D aluminosilicate framework within clinoptilolite, compensating for the overall net negative charge of the framework (Palmer and Gunter Citation2001). Based on the previous experiment (section 3.2), the increased attachment of clinoptilolite on the biofilm coupon should correspond to greater Cs and Sr removal in the biofilm over non-biofilm systems. However, as there was not a large difference in percentage removal between the biofilm and non-biofilm system for both Cs and Sr (Table S1), it could be suggested that there was there was maximum uptake to the clinoptilolite in both systems and the increased clinoptilolite on the biofilm coupon could be in excess. Moreover, biofilms can act as diffusion barriers (Chen and Stewart Citation1996; Anderson et al. Citation2006) and so could prevent access for the metals to the entrapped clinoptilolite.
Figure 5. Concentration (µM) from ICP-MS of solutions containing Cs (a), Sr (b), Ru (c), Co (d), and Eu (e) in the biofilm and non-biofilm circulating MRD systems over a period of 8 days.
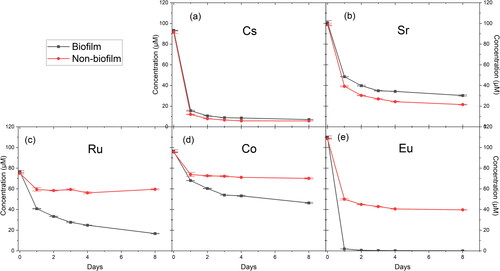
Interestingly, considerably higher uptake levels for Co, Ru and Eu were seen in the biofilm system (52, 78, and 100% removal, respectively), compared to the non-biofilm system (27, 21, and 63% removal, respectively) (). There is evidence in the literature of Ru (Kabiri-Tadi and Faghihian Citation2011), Co (Mamba et al. Citation2009), and Eu (Levenets et al. Citation2020) uptake into clinoptilolite, however, the substantially increased uptake in the biofilm system over the non-biofilm indicates that there was a biologically-mediated mechanism of enhanced removal for these metal species. Additionally, the concentrations of Ru, Co and Eu in the non-biofilm system are shown to plateau after day 1, whereas the concentrations of Ru and Co in the biofilm system maintain a steady decrease over the 8 days. This difference could be due to continual uptake within the biofilm or sustained biomineralisation processes. Further support includes the LIVE/DEAD staining of the biofilm coupon in which shows a dense biofilm of green alive cells, which could be capable of metal uptake.
LA-ICP-MS spot sample analysis of the coupon surfaces (in μg.g−1) showed overall elevated levels of 19 times more Si and 20 times more Al on the biofilm-coated compared to non-biofilm coupon (). This indicates there was more clinoptilolite attached to the surface of the biofilm coupon, which is consistent with the SEM observations. Higher concentrations of all the metals apart from Eu were detected on the biofilm coupon compared to the non-biofilm coupon. Another coupon from the same biofilm and non-biofilm MRDs was analysed as a replicate and showed very similar results (Table S4). The spot data was also corroborated by LA-ICP-MS maps (80 × 40 µm) which were taken of the biofilm and non-biofilm coupon surfaces (). Increased intensity was seen in the biofilm coupon maps over the non-biofilm coupon maps for the Si and Al from the clinoptilolite and all of the metals apart from Eu. The increased removal of Eu in the non-biofilm system is inconsistent with the removal seen from solution ICP-MS analyses of 100% removal for the biofilm system compared to 63% for the non-biofilm system. However, the absolute Eu abundance from the LA-ICP-MS spot results () showed the increase on the non-biofilm surface (129 ± 66 μg.g−1 of Eu) compared to the biofilm coupon (155 ± 182 μg.g−1 of Eu) is small. These results could suggest that the Eu uptake is controlled by ion exchange with the clinoptilolite as it is present in both the biofilm and non-biofilm systems. The Eu sorption coefficient to clinoptilolite is relatively high at 72.7%, and this is twice as high as synthetic zeolites (Ks (NaA) − 36.8%, Ks (NaX) − 25.2%) (Levenets et al. Citation2020). Biofilms have also been found to be capable of accumulating Eu via mechanisms including biosorption and biomineralisation (Ozaki et al. Citation2004; Gangappa et al. Citation2017; Maleke et al. Citation2019; Jena et al. Citation2021), however, the LA-ICP-MS evidence suggests that these are not the dominant mechanism at play in these systems. Alternatively, the increased Eu on the non-biofilm coupon could be sorbed to the steel surface (Dehghani et al. Citation2020), as more steel was uncovered on the non-biofilm coupon because of decreased clinoptilolite and lack of biofilm coverage. This is also supported by the LA-ICP-MS maps () which showed Eu intensity without co-location of any Si or Al intensity from clinoptilolite.
Figure 6. LA-ICP-MS elemental maps (80 x 40 µm) of Co, Cs, Eu, Ru, Sr, Al and Si on the surface of the biofilm and non-biofilm coupons. The intensities are normalised to the Cr detected as it was consistent across the biofilm and non-biofilm systems.
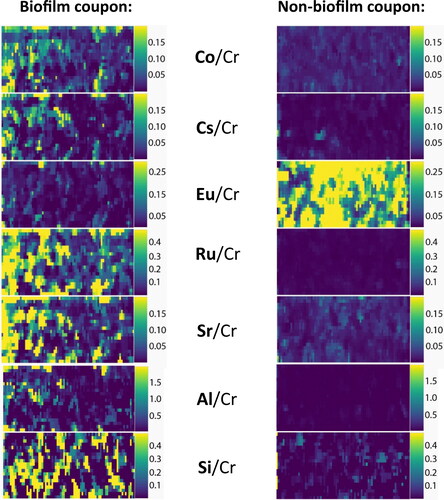
Table 2. Average qualitative concentrations (μg.g−1) of elements detected on the surface of the biofilm and non-biofilm MRD system coupons via LA-ICP-MS (15 spots averaged per sample, with standard deviations shown, all data to 3 significant figures).
The increased concentrations of Co and Ru on the biofilm coupon are in agreement with the solution ICP-MS data, providing further evidence for biofilms being the main uptake mechanism for these radionuclides within the Sellafield pipe fouling. This biological uptake mechanism is also supported by the LA-ICP-MS maps which showed some areas of Co and Ru intensity which do not co-locate with Si or Al of clinoptilolite and thus are likely associated with the biofilm which has entrapped the clinoptilolite. These findings highlight the importance of considering biofilms and microbial biomass in decommissioning scenarios, as well as the potential need for biocides in decommissioning and contamination prevention strategies.
Biofilms form when microorganisms adhere to a surface, first with weak Van der Waals forces then via the production of an adhesive extracellular polymeric substance (EPS) matrix, consisting of polymeric substances such as proteins and polysaccharides (Bixler and Bhushan Citation2012). Radionuclides and metal contaminants can be incorporated into biofilms primarily via two mechanisms; bioaccumulation, which is metabolism dependant, and biosorption, which is metabolism independent (van Hullebusch et al. Citation2003; Sulaymon et al. Citation2014; Van Hullebusch and Pechaud Citation2015; Shamim Citation2018). Co may be taken up into the cell as an essential micronutrient (Waldron and Robinson Citation2009; Veyrier and Cellier Citation2014), however, bioaccumulation can also occur for Co without a clear metabolic function (Gao et al. Citation2017; Maleke et al. Citation2019). Biosorption is caused by metals binding to functional groups of the cell wall or EPS, e.g. amine, carboxyl, hydroxyl, phosphate, and sulfate (Shamim Citation2018). Biofilms are capable of biosorbing Co (Liu et al. Citation2001; Haack and Warren Citation2003), and Ru (Gibson et al. Citation1986; Colica et al. Citation2012; Kora et al. Citation2016; Southam et al. Citation2017). Subsequently, there are feasible biologically-mediated mechanisms that could be increasing Co and Ru uptake in biofilm coupons over non-biofilm coupons. Biofouling in radioactive discharge pipelines could, therefore, be contributing to radioactive contamination.
To further explore the fate of the metals in the coupon experiments, the speciation of the metals within the biofilm and non-biofilm systems was investigated using XPS. Of the metals investigated (Sr, Cs, Co, Eu, and Ru), XPS was able to detect only Co and Ru. A very limited amount of Co was detected on the biofilm coupon surface, seen as a small peak above the background in . In contrast, no Co was detected on the surface of the non-biofilm coupon (). Ru was also detected via XPS on both the biofilm and non-biofilm coupons ( and ; binding energies in Table S5). This is in agreement with the LA-ICP-MS spot data ( and Table S4) where Ru was the highest concentration metal for both the biofilm and non-biofilm coupon, followed by Co, which had a higher concentration on the biofilm over non-biofilm coupon. Although comparatively the LA-ICP-MS was able to detect all of the metals, this is not unexpected as it is a higher sensitivity instrument with lower detection limits than XPS.
Figure 7. Co 2p3/2 XPS region for the biofilm (a) and non-biofilm (b) coupons, and Ru 3p3/2 XPS region for the biofilm (c) and non-biofilm (d) coupons.
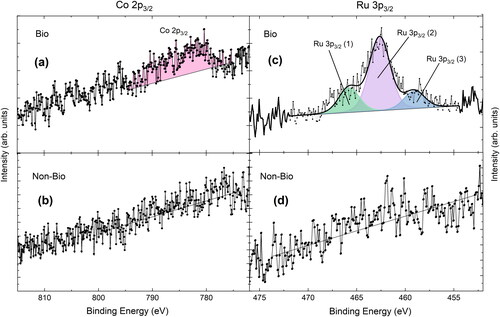
The XPS data showed the biofilm coupon sample had higher atomic percentages of C-O and C = O compared to non-biofilm coupon (Table S6). This is likely due to the presence of C=O/amide and C-O/amine interactions from cells on the biofilm coupon, noted in the literature for S. oneidensis (Neal et al. Citation2002) and other bacteria (Van Der Mei et al. Citation2000; Gomez-Bolivar et al. Citation2019). Two of the Ru species identified on the biofilm and non-biofilm coupon are likely to be RuCl3 (at 283.0 eV and 282.9 respectively) and the hydrolysis product Ru(OH)3 (both at 281.4 eV) (Morgan Citation2015; Gomez-Bolivar et al. Citation2019; Omajali et al. Citation2019). On the biofilm coupon, a third Ru species at a lower binding energy was required for a sufficient fit of the XPS data. The presence of three Ru species is also clearly seen in the biofilm Ru 3p peak shown in (but not in the corresponding non-biofilm coupon; ). It is suggested that the third Ru species on the biofilm coupon is a bioreduced species of Ru. Indeed, S. oneidensis MR-1 is capable of bioreduction of a wide range of metals (Lloyd Citation2003), the reduction is electrochemically feasible (Ru(III) + e− → Ru(II) E°/V = 0.25 V and Ru(II) + 2e− → Ru(0) E°/V = 0.455 V; (Antelman and Harris Citation1982)), and Ru bioreduction (mainly in Ru and Ru/Pd nanoparticles) has been reported previously (Sano et al. Citation2016; Gomez-Bolivar et al. Citation2019; Omajali et al. Citation2019; Gupta and Mishra Citation2020). There are examples of both Ru(II) and Ru(0) forming from Ru(III) in reducing and bioreducing systems (Miyazaki et al. Citation2001; Sano et al. Citation2016; Gomez-Bolivar et al. Citation2019; Omajali et al. Citation2019; Gupta and Mishra Citation2020) and the Ru species noted here (280.0 eV) is within the binding energy range of both (see Table S7). We also note that no other expected element or species (also verified using survey spectra) would present spectral features at this binding energy. Fitting of the Ru 3d peak, as overlapping C 1s, was performed using careful constraint of the peak fit with the well-known spin-orbit splitting of Ru 3d of ∼3.2 eV and the expected binding energy positions of C 1s species (Hrbek et al. Citation1995).
4. Conclusion
In this study, we investigated the factors that contribute to the fouling of Sellafield discharge pipelines. The role of inorganic and biological elements of the pipeline systems were tested, including the potential of clinoptilolite fines, polyelectrolyte, and microbial biofilms in promoting fouling formation. Biofilms were found to have a significant impact by promoting the attachment of clinoptilolite fines by ∼20 times, in contrast, polyelectrolytes were deemed unimportant, leading to a decrease in clinoptilolite fines attachment with increasing polyelectrolyte concentrations. Biofilms directly entrapped clinoptilolite, and played a major role in fouling formation in the MRD flow-through systems. Metal/radionuclide retention in the fouling system was found to be linked to both the clinoptilolite and the microbial component. For Cs, Sr, and potentially Eu, ion exchange with clinoptilolite was likely to be the dominant mechanism of uptake in both the biofilm and non-biofilm systems. In contrast, biologically-mediated uptake mechanisms were deemed to be important for Co and Ru accumulation. This was indicated by the increased and continual removal of Co and Ru from solution and the accumulation of these metals in the biofilm-coated system, yet more work is required to confirm and define the specific mechanisms. Of particular note here was the identification of a bioreduced species of Ru detected via XPS on the biofilm coated system, adding to the accumulating literature on Ru bioreduction/interactions with microbial cells (Gibson et al. Citation1986; Sano et al. Citation2016; Southam et al. Citation2017; Gomez-Bolivar et al. Citation2019; Omajali et al. Citation2019). This work highlights the importance of limiting biomass accumulation in these pipeline systems, to minimise the propagation of fouling and the accumulation of inorganic particulates and associated radionuclides. As a result, strategies to control future biofouling can include limiting the nutrients in the effluent, while the use of biocides could also be considered. This would also mitigate the risk of microbiologically influenced corrosion which is associated with biofouling.
Although this research demonstrates an important use of surrogate experimental systems to decipher key fouling formation mechanisms and impacts, real pipeline systems are clearly more complex. For example, there will be a wide range of different effluent sources passing through the pipelines throughout the long operating periods for major nuclear facilities. In this study we used a simplified monoculture system, but real-world fouling systems will be made up of more complex microbial communities, exhibiting a wider range of processes including biomineralisation reactions (Lloyd and Macaskie Citation2000) that may impact on the fate of metals. Clearly there is a need for detailed chemical, biological and radiological characterisation of a wide range of real world field samples.
Supplemental Material
Download PDF (1.4 MB)Acknowledgements
We also acknowledge access to the EPSRC NNUF RADER Facility (EP/T011300/1) for analyses performed in this work, and The University of Manchester and the Science and Technology Facilities Council (#ST/S002170/1) for funding the LA-ICP-MS facility. We acknowledge the significant contribution of the work from Dr Robert Jones’s thesis (Jones Citation2016) in informing this research.
Disclosure statement
The authors report there are no competing interests to declare.
Additional information
Funding
References
- Anderson C, Pedersen K, Jakobsson AM. 2006. Autoradiographic comparisons of radionuclide adsorption between subsurface anaerobic biofilms and granitic host rocks. Geomicrobiol J. 23:15–29. doi:10.1080/01490450500399946.
- Antelman MS, Harris FJ. 1982. Electrochemical series. The encyclopedia of chemical electrode potentials, p. 1–81. Boston (MA): Springer. doi:10.1007/978-1-4613-3374-6_1.
- Barton F, Shaw S, Morris K, Graham J, Lloyd JR. 2022. Impact and control of fouling in radioactive environments. Prog Nucl Energy. 148:104215. Elsevier. doi:10.1016/j.pnucene.2022.104215.
- Bixler GD, Bhushan B. 2012. Biofouling: lessons from nature. Philos Trans A Math Phys Eng Sci. 370:2381–2417. doi:10.1098/rsta.2011.0502.
- BNFL. 2004. SIXEP sea discharge line solids – initial analysis and results.
- British Nuclear Group. 2005. SIXEP Sea line solids : review of mechanisms of formation.
- Chen X, Stewart PS. 1996. Chlorine penetration into artificial biofilm is limited by a reaction-diffusion interaction. Environ Sci Technol. 30:2078–2083. doi:10.1021/es9509184.
- Claesson PM, Poptoshev E, Blomberg E, Dedinaite A. 2005. Polyelectrolyte-mediated surface interactions. Adv Colloid Interface Sci. 114-115:173–187. doi:10.1016/j.cis.2004.09.008.
- Colica G, Caparrotta S, De Philippis R. 2012. Selective biosorption and recovery of Ruthenium from industrial effluents with Rhodopseudomonas palustris strains. Appl Microbiol Biotechnol. 95:381–387. doi:10.1007/s00253-012-4053-9.
- Dehghani A, Mostafatabar AH, Bahlakeh G, Ramezanzadeh B. 2020. A detailed study on the synergistic corrosion inhibition impact of the Quercetin molecules and trivalent europium salt on mild steel; electrochemical/surface studies, DFT modeling, and MC/MD computer simulation. J Mol Liq. Elsevier B.V., 316:113914. doi:10.1016/j.molliq.2020.113914.
- Dyer A, Chimedtsogzol A, Campbell L, Williams C. 2006. Uptake of caesium and strontium radioisotopes by natural zeolites from Mongolia. Microporous Mesoporous Mater. 95:172–175. doi:10.1016/j.micromeso.2006.05.013.
- Foster L, Boothman C, Ruiz-Lopez S, Boshoff G, Jenkinson P, Sigee D, Pittman JK, Morris K, Lloyd JR. 2020. Microbial bloom formation in a high pH spent nuclear fuel pond. Sci Total Environ. 720:137515. doi:10.1016/j.scitotenv.2020.137515.
- Foster L, Muhamadali H, Boothman C, Sigee D, Pittman JK, Goodacre R, Morris K, Lloyd JR. 2020. Radiation tolerance of Pseudanabaena catenata, a Cyanobacterium relevant to the first generation Magnox storage pond. Front Microbiol. 11:515. doi:10.3389/fmicb.2020.00515.
- Gallup DL, von Hirtz P. 2015. Control of silica-based scales in cooling and geothermal systems, mineral scales and deposits: scientific and technological approaches. Amsterdam, Netherlands: Elsevier B.V. doi:10.1016/B978-0-444-63228-9.00022-X.
- Gangappa R, Farrier A, Macaskie LE. 2017. Eu3+ sequestration by biogenic nano-hydroxyapatite synthesized at neutral and alkaline pH. Geomicrobiol J. Taylor & Francis, 34:753–759. doi:10.1080/01490451.2016.1261966.
- Gao R, Wang Y, Zhang Y, Tong J, Dai W. 2017. Cobalt(II) bioaccumulation and distribution in Rhodopseudomonas palustris. Biotechnol Biotechnol Equip. Taylor & Francis, 31:527–534. doi:10.1080/13102818.2017.1292148.
- George RP, Kamachi Mudali U, Raj B. 2016. Characterizing biofilms for biofouling and microbial corrosion control in cooling water systems. ACMM. 63:477–489. doi:10.1108/ACMM-07-2014-1401.
- Gibson JF, Poole RK, Hughes MN, Rees JF. 1986. Ruthenium nitrosyl complexes: toxicity to Escherichia coli and yeasts and uptake by marine bacteria. Arch Environ Contam Toxicol. 15:519–523. doi:10.1007/BF01056564.
- Gomez-Bolivar J, Mikheenko IP, Orozco RL, Sharma S, Banerjee D, Walker M, Hand RA, Merroun ML, Macaskie LE. 2019. Synthesis of Pd/Ru bimetallic nanoparticles by Escherichia coli and potential as a catalyst for upgrading 5-hydroxymethyl furfural into liquid fuel precursors. Front Microbiol. 10:1276. doi:10.3389/fmicb.2019.01276.
- Gupta PK, Mishra L. 2020. Ecofriendly ruthenium-containing nanomaterials: synthesis, characterization, electrochemistry, bioactivity and catalysis. Nanoscale Adv. 2:1774–1791. Royal Society of Chemistry, doi:10.1039/d0na00051e.
- Haack EA, Warren LA. 2003. Biofilm hydrous manganese oxyhydroxides and metal dynamics in acid rock drainage. Environ Sci Technol. 37:4138–4147. doi:10.1021/es026274z.
- Hrbek J, van Campen DG, Malik IJ. 1995. The early stages of ruthenium oxidation. Journal of Vacuum Science & Technology A: vacuum, Surfaces, and Films. 13:1409–1412. doi:10.1116/1.579573.
- Jena A, Pradhan S, Mishra S, Sahoo NK. 2021. Evaluation of europium biosorption using Deinococcus radiodurans. Environ Process. 8:251–265. doi:10.1007/s40710-020-00479-8.
- Jones R. 2016. Formation and attachment of solids within a pipeline emanating from a nuclear efflunet treatment plant [thesis]. Manchester (UK): University of Manchester.
- Kabiri-Tadi M, Faghihian H. 2011. Removal of ruthenium from aqueous solution by clinoptilolite. Clays Clay Miner. 59:34–41. doi:10.1346/CCMN.2011.0590106.
- Kharazmi A, Giwercman B, Hoiby N. 1999. Robbins device in biofilm research. Methods Enzymol. 310:207–215. doi:10.1016/s0076-6879(99)10018-1.
- Kora AJ, Bhaskarapillai A, Toleti SR. 2016. Exopolymer produced by Pseudomonas aeruginosa: a super sorbent for ruthenium. Separation Science and Technology (Philadelphia). Taylor & Francis, 51:1–6. doi:10.1080/01496395.2016.1166133.
- Levenets VV, Lonin AY, Omelnik OP, Shchur AO. 2020. Studies of the features of the sorption of an europium by natural and synthetic zeolites for using it in the nuclear energy. Probl Atomic Sci Technol. 125:121–126. doi:10.46813/2020-125-121.
- Li J, Tang M, Ye Z, Chen L, Zhou Y. 2017. Scale formation and control in oil and gas fields: a review. J Dispersion Sci Technol. 38:661–670. Taylor & Francis. doi:10.1080/01932691.2016.1185953.
- Lin CC. 2009. A review of corrosion product transport and radiation field buildup in boiling water reactors. Prog Nucl Energy. 51:207–224. Elsevier. doi:10.1016/j.pnucene.2008.05.005.
- Liu S, Gunawan C, Barraud N, Rice SA, Harry EJ, Amal R. 2016. Understanding, monitoring, and controlling biofilm growth in drinking water distribution systems. Environ Sci Technol. 50:8954–8976. doi:10.1021/acs.est.6b00835.
- Liu Y, Lam MC, Fang HHP. 2001. Adsorption of heavy metals by EPS of activated sludge. Water Sci Technol. 43:59–66. doi:10.2166/wst.2001.0340.
- Lloyd JR. 2003. Microbial reduction of metals and radionuclides. FEMS Microbiol Rev. 27:411–425. doi:10.1016/S0168-6445(03)00044-5.
- Lloyd JR, Macaskie LE. 2000. Bioremediation of radionuclide‐containing wastewaters. In: Lovley DR, editors. Environmental microbe–metal interactions. Washington (DC): American Society for Microbiology; pp. 277–327.
- Lloyd JR, Renshaw JC. 2005. Bioremediation of radioactive waste: radionuclide-microbe interactions in laboratory and field-scale studies. Curr Opin Biotechnol. 16:254–260. doi:10.1016/j.copbio.2005.04.012.
- Macadam J, Parsons SA. 2004. Calcium carbonate scale control, effect of material and inhibitors. Water Sci Technol. 49:153–159. doi:10.2166/wst.2004.0112.
- Magnox North. 2008. Information in support of applications by Magnox Electric Limited under the Radioactive Substances Act 1993 To Dispose And Discharge Radioactive Wastes From Chapelcross Site Hefin Griffiths Robert Millard Contents P.
- Maleke M, Valverde A, Vermeulen JG, Cason E, Gomez-Arias A, Moloantoa K, Coetsee-Hugo L, Swart H, Van Heerden E, Castillo J. 2019. Biomineralization and bioaccumulation of europium by a thermophilic metal resistant bacterium. Front Microbiol. 10:81. doi:10.3389/fmicb.2019.00081.
- Mamba BB, Nyembe DW, Mulaba-Bafubiandi AF. 2009. Removal of copper and cobalt from aqueous solutions using natural clinoptilolite. Water SA. 35:307–314. doi:10.4314/wsa.v35i3.76768.
- Miyazaki A, Balint I, Aika KI, Nakano Y. 2001. Preparation of Ru nanoparticles supported on γ-Al2O3 and its novel catalytic activity for ammonia synthesis. J Catal. 204:364–371. doi:10.1006/jcat.2001.3418.
- Morgan DJ. 2015. Resolving ruthenium: XPS studies of common ruthenium materials. Surface & Interface Analysis. 47:1072–1079. doi:10.1002/sia.5852.
- Muryanto, S., Bayuseno, A.P., Ma’mun, H., Usamah, M., Jotho, (2014) ‘Calcium carbonate scale formation in pipes: effect of flow rates, temperature, and malic acid as additives on the mass and morphology of the scale. Proc Chem. 9:69–76. Elsevier. doi:10.1016/j.proche.2014.05.009.
- Myers CR, Nealson KH. 1988. Bacterial manganese reduction and growth with manganese oxide as the sole electron acceptor. American Association for the Advancement of Science Stable. http://www.jstor.org/stable/1701057. Science, 240(4857): 1319–1321.
- Neal AL, Lowe K, Daulton TL, Jones-Meehan J, Little BJ. 2002. Oxidation state of chromium associated with cell surfaces of Shewanella oneidensis during chromate reduction. Appl Surf Sci. 202:150–159. doi:10.1016/S0169-4332(02)00550-0.
- Nexia Solutions. 2006. Sea discharge line solids analysis and biocide investigations summary report.
- Olajire AA. 2015. A review of oilfield scale management technology for oil and gas production. J Petrol Sci Eng. 135:723–737. doi:10.1016/j.petrol.2015.09.011.
- Omajali JB, Gomez-Bolivar J, Mikheenko IP, Sharma S, Kayode B, Al-Duri B, Banerjee D, Walker M, Merroun ML, Macaskie LE. 2019. Novel catalytically active Pd/Ru bimetallic nanoparticles synthesized by Bacillus benzeovorans. Sci Rep. 9:4715. Springer US. doi:10.1038/s41598-019-40312-3.
- Owens S, Higgins-Bos M, Bankhead M, Austin J. 2015. Using chemical and process modelling to design, understand and improve an effluent treatment plant. NNL Sci. 3:1–13.
- Ozaki T, Gillow JB, Kimura T, Ohnuki T, Yoshida Z, Francis AJ. 2004. Sorption behavior of europium(III) and curium(III) on the cell surfaces of microorganisms. Radiochim Acta. 92:741–748. doi:10.1524/ract.92.9.741.55006.
- Palmer JL, Gunter ME. 2001. The effects of time, temperature, and concentration on Sr2+ exchange in clinoptilolite in aqueous solutions. Am Mineral. 86:431–437. doi:10.2138/am-2001-0406.
- Paton C, Hellstrom J, Paul B, Woodhead J, Hergt J. 2011. Iolite: freeware for the visualisation and processing of mass spectrometric data. J Anal Spectrom. 26:2508–2518. doi:10.1039/c1ja10172b.
- Prusek T, Oukacine F, Hervouet C. 2017. A Methodology to simulate the impact of tube fouling on steam generator performance with a thermal-hydraulic code. Heat Transfer Eng. 38:721–729. Taylor & Francis. doi:10.1080/01457632.2016.1206413.
- Rao TS, Kora AJ, Chandramohan P, Panigrahi BS, Narasimhan SV. 2009. Biofouling and microbial corrosion problem in the thermo-fluid heat exchanger and cooling water system of a nuclear test reactor. Biofouling. 25:581–591. doi:10.1080/08927010903016543.
- Rao TS, Nair KVK. 1998. Microbiologically influenced stress corrosion cracking failure of admiralty brass condenser tubes in a nuclear power plant cooled by freshwater. Corros Sci. 40:1821–1836. doi:10.1016/S0010-938X(98)00079-1.
- Sandt C, Smith Palmer T, Pink J, Pink D. 2008. Quantification of local water and biomass in wild type PA01 biofilms by confocal Raman microspectroscopy. J Microbiol Methods. 75:148–152. doi:10.1016/j.mimet.2008.05.012.
- Sani RK, Peyton BM, Dohnalkova A. 2008. Comparison of uranium(VI) removal by Shewanella oneidensis MR-1 in flow and batch reactors. Water Res. 42:2993–3002. doi:10.1016/j.watres.2008.04.003.
- Sano N, Nakanishi Y, Sugiura K, Yamanaka H, Tamon H, Saito N, Konishi Y. 2016. Synthesis of bimetallic Pt–Ru nanoparticles by bioreduction using Shewanella algae for application to direct methanol fuel cell. J Chem Eng Japan / JCEJ. 49:488–492. doi:10.1252/jcej.15we077.
- Sarró MI, García AM, Moreno DA. 2005. Biofilm formation in spent nuclear fuel pools and bioremediation of radioactive water. Int Microbiol. 8:223–230. doi: im2305032.[pii].
- Satpathy KK, Kumar A, Sahu G, Biswas S, M S. 2010. Biofouling and its control in seawater cooled power plant cooling water system – a review, nuclear power. Rijeka: Sciyo. doi:10.5772/9912.
- Shamim S. 2018. Biosorption of heavy metals. Intech. Available at: https://www.intechopen.com/books/advanced-biometric-technologies/liveness-detection-in-biometrics.
- Southam HM, Butler JA, Chapman JA, Poole RK. 2017. The microbiology of ruthenium complexes. In: Poole RK, editors. Advances in microbial physiology. 1st ed., Vol. 71. London (UK): Elsevier. doi:10.1016/bs.ampbs.2017.03.001.
- Sulaymon AH, Abbas SH, Ismail IM, Mostafa TM, Sulaymon AH. 2014. Biosorption of heavy metals: a review. Available at: https://www.researchgate.net/publication/266795209.
- Supekar OD, Brown JJ, Greenberg AR, Gopinath JT, Bright VM. 2018. Real-time detection of reverse-osmosis membrane scaling via Raman spectroscopy. Ind Eng Chem Res. 57:16021–16026. doi:10.1021/acs.iecr.8b01272.
- Terachi T, Fujii K, Arioka K. 2005. Microstructural characterization of SCC crack tip and oxide film for SUS 316 stainless steel in simulated PWR primary water at 320 °C. J Nucl Sci Technol. 42:225–232. doi:10.1080/18811248.2005.9726383.
- Tišáková L, Pipíška M, Godány A, Horník M, Vidová B, Augustín J. 2013. Bioaccumulation of 137Cs and 60Co by bacteria isolated from spent nuclear fuel pools. J Radioanal Nucl Chem. 295:737–748. doi:10.1007/s10967-012-1932-6.
- Turner CW, Khumsa-Ang K. 2017. Corrosion product transport and fouling in nuclear steam generators. In: Steam generators for nuclear power plants. Cambridge (UK): Woodhead Publishing. Chapter 9; p. 215–271.doi:10.1016/B978-0-08-100894-2.00011-X.
- Van Der Mei HC, De Vries J, Busscher HJ. 2000. X-ray photoelectron spectroscopy for the study of microbial cell surfaces. Surf Sci Rep. 39:1–24. doi:10.1016/S0167-5729(00)00003-0.
- Van Hullebusch ED, Pechaud Y. 2015. Role of natural and engineered biofilms composition in toxic inorganic contaminants immobilisation. Microbiology for minerals, metals, materials and the environment. p. 281–306. New York (NY): CRC Press. doi:10.1201/b18124-15.
- van Hullebusch ED, Zandvoort MH, Lens PNL. 2003. Metal immobilisation by biofilms: mechanisms and analytical tools. Rev Environ Sci Biotechnol. 2:9–33. doi:10.1023/B:RESB.0000022995.48330.55.
- Varga K, Hirschberg G, Németh Z, Myburg G, Schunk J, Tilky P. 2001. Accumulation of radioactive corrosion products on steel surfaces of VVER-type nuclear reactors. II. 60Co. J Nucl Mater. 298:231–238. doi:10.1016/S0022-3115(01)00658-4.
- Veyrier FJ, Cellier MF. 2014. Metal economy in host-microbe interactions. Front Cell Infect Microbiol. 4:190. doi:10.3389/fcimb.2014.00190.
- Vieira MJ, Melo LF. 1995. Effect of clay particles on the behaviour of biofilms formed by pseudomonas fluorescens. Water Sci Technol. 32:45–52. doi:10.2166/wst.1995.0260.
- Waldron KJ, Robinson NJ. 2009. How do bacterial cells ensure that metalloproteins get the correct metal? Nat Rev Microbiol. 7:25–35. doi:10.1038/nrmicro2057.
- Woodhead JD, Hellstrom J, Hergt JM, Greig A, Maas R. 2007. Isotopic and elemental imaging of geological materials by laser ablation inductively coupled plasma-mass spectrometry. Geostand Geoanalyt Res. doi:10.1111/j.1751-908X.2007.00104.x.
- Yin W, Wang Y, Liu L, He J. 2019. Biofilms: the microbial “protective clothing" in extreme environments. Int J Mol Sci. 20:3423.
- Zhang TC, Bishop PL. 1994. Density, porosity, and pore structure of biofilms. Water Res. 28:2267–2277. doi:10.1016/0043-1354(94)90042-6.
- Zhang Y, Ng CK, Cohen Y, Cao B. 2014. Cell growth and protein expression of Shewanella oneidensis in biofilms and hydrogel-entrapped cultures. Mol Biosyst. 10:1035–1042. doi:10.1039/c3mb70520j.