ABSTRACT
The scarcity of fresh water has forced farmers to use saline water (SW) for irrigation. It is important to understand the response of the soil microbial community and diversity to saline irrigation water. The objective of this study was to determine the effects of irrigation water salinity and nitrogen fertilization rates on soil physicochemical properties, microbial activity, microbial biomass, and microbial functional diversity. The field experiment consisted of a factorial design with three levels of irrigation water salinity (electrical conductivities (ECs) of 0.35, 4.61 or 8.04 dS m−1) and two nitrogen rates (0 and 360 kg N ha−1). The results showed that the 4.61 and 8.04 dS m−1 treatments both reduced soil microbial biomass C (MBC), microbial biomass N (MBN), basal respiration, total phospholipid fatty acid (PLFA), bacterial PLFA, fungal PLFA, and fungal:bacterial ratios. In contrast, the SW treatments increased the MBC:MBN ratio. Nitrogen fertilization increased soil MBC, MBN, basal respiration, total PLFA, bacterial PLFA, and gram-negative bacterial PLFA. In contrast, N fertilization decreased gram-positive bacterial PLFA, fungal PLFA, and fungal:bacterial ratios. Average well color development, Richness, and Shannon's Index were always lowest in the 8.04 dS m−1 treatment. Carbon utilization patterns in the 8.04 dS m−1 treatment were different from those in the 0.35 dS m−1 treatment. In conclusion, five years of irrigation with brackish or SW reduced the soil microbial biomass, activity, and functional diversity, which may cause the deterioration of soil quality. Thus, the high-salinity water (EC > 4.61 dS m−1) is not appropriate as a single irrigation water resource. Proper N fertilizer input may overcome some of the negative effects of salinity on soil microbial.
Introduction
Fresh-water shortage and soil salinity are two serious problems limiting sustainable agricultural development in arid regions. The scarcity of fresh water (FW) has forced farmers to use saline water (SW) for irrigation. Continuous irrigation with SW, however, may result in soil salinization, land degradation, and yield decrease. Efforts are being made to identify management practices that prevent or limit salt accumulation in the root zone when SW is used for irrigation (Pereira et al. Citation2002). However, even with careful management, saline irrigation water can alter the physical, chemical, and biological properties of soil.
The influence of salt as a major stress to soil microorganisms has been the subject of several studies. Some studies show that soil microorganisms have the ability to adapt to or tolerate osmotic stress caused by salinity (Wichern et al. Citation2006; Yuan et al. Citation2007). However, many studies indicate that high salt concentrations in soil solution reduce soil microbial biomass (Setia et al. Citation2010; Elgharably & Marschner Citation2011; Yan & Marschner Citation2012). Increasing soil salinity also has adverse effects on biological processes, such as soil respiration (Ghollarata & Raiesi Citation2007). For example, Setia et al. (Citation2010) reported that saline soil (electrical conductivity (EC) ≥ 5.0 dS m−1) reduced soil respiration by more than 50%. Soil microbial functional diversity is widely used to evaluate soil processes and ecological functions (Harris Citation2003; Nannipieri et al. Citation2003; He et al. Citation2009). Jin et al. (Citation2014) reported that average well color development (AWCD) values decreased significantly with increasing irrigation water salinity, thus indicating that irrigation water salinity changed the C utilization patterns of the soil microorganisms (Jin et al. Citation2014). Shen et al. (Citation2008) also observed that soil salinization significantly decreased soil microbial functional diversity.
Proper N fertilizer management can increase the salt tolerance of crops and reduce the adverse effects of salinity on crop growth, development, and yield (Villa-Castorena et al. Citation2003; Esmaili et al. Citation2008). Nitrogen fertilizer application directly or indirectly affects soil chemical, physical, and biological properties, including the composition and function of the soil microbial community (Marschner et al. Citation2003; Hai et al. Citation2010). Shen et al. (Citation2010) reported that N rates of 270 or 300 kg N ha−1 significantly reduced both AWCD and the functional diversity indices in soil under polytunnel greenhouses used for vegetable production. Sarathchandra et al. (Citation2001) observed that 200 and 400 kg N ha−1 significantly reduced soil microbial functional diversity compared with an unfertilized control. However, Lee and Caporn (Citation1998) reported that low N fertilizer rates (e.g. 40 and 80 kg N ha−1) significantly increased the soil microbial functional diversity.
Soil microbial and biochemical properties (e.g. soil microbial biomass, community structure, functional diversity, and basal soil respiration) are powerful tools for monitoring soil biological quality. However, little is known about the interactive effects of irrigation water salinity and N fertilization on soil microbial and biochemical properties under drip-irrigation conditions. The objectives of this study were to evaluate the effects of irrigation water salinity and N fertilization on (1) soil physicochemical properties, (2) soil microbial biomass and basal respiration, (3) phospholipid fatty acid (PLFA) content, and (4) soil microbial functional diversity. The information obtained from this study will be helpful for evaluating the potential of saline irrigation water in arid areas from an environmental standpoint.
Materials and methods
Experimental conditions and treatments
This field experiment was conducted from 2009 to 2013 at the agricultural experiment station of Shihezi University in Xinjiang Province, northwest of China (44°18′ N, 86°02′ E). The soil type is a calcic aridisol (IUSS classification system). The study area is classified as a temperate arid zone with a continental climate. The mean annual precipitation is 210 mm; nearly 60% of this precipitation is received between April and August. The mean annual potential evaporation is 1660 mm and the groundwater table is about 6 m from the surface.
The experiment consisted of a 3 × 2 factorial design with three salinity levels of irrigation water and two N fertilization rates. The six treatments were arranged in a completely randomized block design with three replications. The irrigation rates and fertilizer application rates were the same for each cropping season. The three irrigation treatments were FW (EC = 0.35 dS m−1), brackish water (BW, EC = 4.61 dS m−1) and SW (EC = 8.04 dS m−1). Both BW and SW were prepared by adding equivalent amounts of NaCl and CaCl2 to FW. Selected properties of the irrigation water are shown in . The N fertilization rates were 0 and 360 kg N ha−1 (referred to as N0 and N360, respectively). Nitrogen fertilizer (urea) was applied through a drip-irrigation system during the growing season. All plots were fertilized with 105 kg P2O5 ha−1 (calcium superphosphate) and 60 kg K2O ha−1 (potassium sulfate) before sowing each year. Local farmers typically apply urea at the rate of 360 kg N ha−1.
Table 1. Chemical characteristic of the three types of irrigation water in this study.
Each plot consisted of two 1.2 × 16 m beds, with 0.6-m bare soil between them. Cotton (Gossypium hirsutum L. cv Xinluzao No. 36) was sown at 0.1 m intervals within each row to obtain a population of 222,000 plants ha−1. All plots were drip-irrigated with 30 mm fresh water at sowing to improve germination and seedling establishment. The drip-irrigation emitters were 0.4 m apart. The plots were irrigated nine times between June and August. The irrigation interval was 7–10 d based on field practices used by local farmers for fresh-water-irrigated soils. The plots were all irrigated on the same dates with equal watering depths. Total precipitation and open pan evaporation were 146 and 1260 mm, respectively, during the 2013 growing season.
Soil sampling
Soil samples were collected on 29 July 2013, five years after the treatments were begun. The samples were collected from the 0–20 cm depth at six random locations in each plot. Visible pieces of crop residue (including roots) were removed by hand. The samples were then bagged and placed in a cooler. Soil cores from the same plot were pooled, sieved through a 2.0-mm mesh screen, and then stored in field-moist condition at 4°C.
Soil chemical analysis
The gravimetric water content of the soil was measured by drying the soil at 105°C for 24 h. Soil pH and EC were measured in 1:2.5 and 1:5 soil:water suspensions, respectively. Soil organic matter (OM) was measured using the K2Cr2O7-H2SO4 oxidation–reduction titration method. Total nitrogen (TN) was measured using the semimicro-Kjedahl digestion method. Soil NO3−–N and NH4+–N were measured colorimetrically with a SmartChem140 Discrete Analyzer (Westco Scientific, Danbury, Connecticut, USA) following extraction of 5 g soil in 50 mL 2 mol L−1 KCl for 1 h on a wrist-action shaker.
Soil microbial biomass C and N
Soil microbial biomass C (MBC) and microbial biomass N (MBN) were determined using the fumigation-extraction method and correction factors (KEC, KEN) of 0.38 and 0.54, respectively. Organic C in the extracts was measured using the dichromate oxidation method (Vance et al. Citation1987). Total N in the extracts was measured with the Kjeldahl method (Brookes et al. Citation1985).
Soil basal respiration
Soil basal respiration was measured according to the method of Alef and Nannipieri (Citation1995) with some modifications. Briefly, 30 g of fresh soil was placed in a 500 mL airtight conical flask with a vial containing 5 mL of 0.5 mol L−1 NaOH. Each treatment was replicated three times. The samples were incubated for 1 d in the dark at 25°C. Soil basal respiration rate was calculated based on cumulative CO2 evolution over a 1 d period.
PLFA analysis
Microbial community structure was determined using PLFA analysis. The PLFAs were extracted from soil samples using a modified Bligh–Dyer procedure (Frostegård et al. Citation1993; Bligh & Dyer Citation1995). Briefly, 5 g freeze-dried soil was shaken for 2 h in a mixture of phosphate buffer, chloroform, and methanol (0.8/1/2, v/v/v). Phospholipids were separated from neutral lipids and the glycolipids were eluted with chloroform, acetone, and methanol, respectively, on a solid phase extraction column. The phospholipids in the methanol fraction were transesterified into fatty acid methyl esters using a 0.2 mol/L methanolic KOH solution. The PLFAs were quantified with a gas chromatograph (Agilent 5890) equipped with a flame ionization detector and an HP-5 fused silica capillary column (30.0 m × 320 um × 0.25 um). Helium was the carrier gas. The initial temperature was 140°C for 4 min, followed by heating rates of 4°C min−1 to 190°C with a 1 min pause, 3°C min−1 to 230°C with a 1 min pause, 2°C min−1 to 250°C with a 2 min pause, and 10°C min−1 to 300°C with a final pause of 1 min. The fatty acid 19:0 was added as an internal standard and the PLFAs were identified using Bacterial Acid Methyl Esters Mix and Supelco 37 Component FAME Mix (Sigma, USA).
Total microbial PLFAs were calculated as the sum of all identified PLFA peaks. Signature PLFAs were used as indicators for specific microbial groups (gram-positive (G+) bacterial: i14:0, i15:0, a15:0, i16:0, i17:0, a17:0, i19:0, a19:0; gram-negative (G−) bacterial: 16:1ω9c, cy17:0, 17:1ω9c, cy19:0; Fungi: 18:1ω9c and 18:3ω6c; Zelles Citation1997; Zak et al. Citation2000; Pankhurst et al. Citation2001; Amir et al. Citation2008; Zornoza et al. Citation2009). The total bacterial PLFA was calculated as the sum of the biomarker PLFAs for G+ bacteria and G− bacteria plus those at 14:0, 15:0, 16:0, and 17:0 (Buchan et al. Citation2012).
Soil microbial functional diversity
Biolog Eco-microplates (Biolog, Hayward, CA, USA) were used to determine the functional diversity of the soil microbial communities (Garland and Mills Citation1991). Shannon, Evenness, Richness, and Simpson indices were calculated according to the methods of Zak et al. (Citation1994) and Zhang et al. (Citation2008). In brief, 5 g of fresh soil was added to 50 mL of phosphate-buffered saline (PBS) solution in sterile 150 mL centrifuge tubes. The PBS solution was prepared by mixing 6.8 g NaCl, 0.24 g KH2PO4, and 0.896 g Na2HPO4·7H2O in about 800 mL of water. The pH of the PBS solution was adjusted to 8.0 by adding 0.1 mol L−1 NaOH. Finally, the solution was diluted with water to bring the volume to 1 L. The solutions in the centrifuge tubes were shaken for 30 min at 220 rpm. Ten-fold dilutions (10−1 and 10−3 dilutions) were prepared and then 150 μL aliquots of each soil suspension were inoculated into Biolog microtiter plates with 96 wells. The plates included 31 C sources as well as one well with no C source. The plates were incubated at 25°C. Carbon utilization by microorganisms caused color development in the wells. The absorbance of this color was measured at 590 nm after incubation for 0, 12, 24, 36, 48, 60, 72, 84, 96, 108, and 120 h and recorded using Microlog 4.01 software (Biolog, Hayward, CA, USA).
The AWCD values at 72 h were used to calculate (1) Richness index (R), (2) Shannon index (H’), (3) Shannon–Wiener evenness index (E), and (4) Simpson index (D) for the species richness of the community. The following equations were used for the calculations:
where Ci is the optical density value measured at 590 nm in substrate i in the Eco Plates, and R is the value measured in the control well.
Richness (S) (i.e. the number of oxidized C sources) was calculated using a transformed value of > 1 as the threshold for positive response. The pattern of substrate utilization of each plate was expressed using transformed data calculated by dividing the raw difference value for each well by the AWCD of the plate: (Ci−R)/∑ (Ci−R)/31. Transformed values >1 indicate that color responses are positive:
where pi is the ratio between the activity of each substrate and the sum of the activities of all substrates, Pi =(Ci−R)/∑(Ci−R).
where H′ is Shannon diversity index and S is substrate richness.
where pi is the ratio between the activity of each substrate and the sum of the activities of all substrates, Pi=(Ci−R)/∑(Ci−R).
Statistical analysis
The data were analyzed using SPSS statistical software (v. 11.0) with a two-way ANOVA at a significance level of 0.05. Irrigation water salinity and N fertilization rate were the independent variables. Treatment means were compared using Tukey's test at P = 0.05 when significant (P < 0.05) differences were detected among the irrigation water salinity or N fertilization rate treatments. Principal component analyses (PCA) of both the PLFA signatures and the Eco-plate data (72 h) were performed using Canoco 4.5 software based on the identified PLFAs regimentation and substrate utilization data.
Results
Soil physicochemical properties
The effect of water salinity, N rate and their interaction on selected soil properties are summarized in . Soil water content, salinity, and NH4–N increased as irrigation water salinity increased. In contrast, soil pH, TN, OM, and NO3–N decreased as irrigation water salinity increased. Averaged across all three water salinities, N fertilization increased soil salinity, NO3–N, NH4–N, TN, and OM, but decreased soil water content and pH.
Table 2. Soil chemical properties as affected by irrigation water salinity and N fertilization.
Soil microbial biomass and basal respiration
Irrigation water salinity and N fertilization both had significant main effects on soil MBC, MBN, MBC/MBN, and basal respiration (). Soil MBC, MBN, and basal respiration decreased as irrigation water salinity increased. Averaged across both N fertilization rates, irrigation with BW or SW reduced soil MBC by 20–36%, MBN by 39–68%, and basal respiration by 15–28%. In contrast, the MBC/MBN ratio increased by 21–80% as irrigation water salinity increased. Averaged across all three water salinities, N fertilization increased MBC by 40%, MBN by 110%, and basal respiration by 43%. Nitrogen fertilization reduced the MBC/MBN ratio by 24%.
Table 3. Soil microbial biomass C (MBC), microbial biomass N (MBN), and basal respiration as affected by irrigation water salinity and N fertilization.
Microbial community structure assessed by the PLFAs
Total PLFA concentrations can be used to indicate the total biomass of soil microbial communities. In the N0 plots, total PLFA, bacterial PLFA, fungal PLFA, and the fungal:bacterial PLFA ratio decreased significantly as irrigation water salinity increased (). The effects of irrigation water salinity varied depending on the type of PLFAs. The G+ bacterial PLFA was also greatest in the FW treatment; the differences between the BW and SW treatments were not significant. There was no significant difference in G− bacterial PLFA between the FW and BW treatments. In the N360 plots, total PLFA, bacterial PLFA, G− bacterial PLFA fungal PLFA, and the fungal:bacterial PLFA ratio were generally greatest in the FW treatment; however, the differences among the treatments were not always significant. The G+ bacterial PLFA was greatest in the BW treatment. Averaged across all three water salinities, total PLFA, bacterial PLFA, and G− bacterial PLFA were 32%, 31%, and 32% greater, respectively, in the N360 treatments than in the N0 treatments. In contrast, G+ bacterial PLFA, fungal PLFA, and the fungal:bacterial PLFA ratio were 14%, 58%, and 114% greater, respectively, in the N0 treatment than in the N360 treatment.
Table 4. Relative abundances of microbial groups based on PLFA (ug kg−1) analysis as affected by irrigation water salinity and N fertilization
The PLFA data were subject to PCA to examine if patterns of species combinations could clearly separate the soil samples according to the different treatments (). The first two principal components (PC1 and PC2) together explained 99.8% of the total variation in the data. This indicated that soil microbial community composition varied significantly among different irrigation water salinity and N rate treatments. There were four clusters: (1) FWN0 (2) BWN0; (3) SWN0; (4) FWN360, BWN360, and SWN360.
Figure 1. PCA of PLFA profiles as affected by irrigation water salinity and N fertilization. PC1 described 94.3% and PC2 5.0% of variation in the data. Abbreviations: FWN0 stand for water salinity of 0.35 dS m−1 with unfertilized treatment; FWN360 stand for water salinity of 0.35 dS m−1 with fertilized treatment; BWN0 stand for water salinity of 4.61 dS m−1 with unfertilized treatment; BWN360 stand for water salinity of 4.61 dS m−1 with fertilized treatment; SWN0 stand for water salinity of 8.04 dS m−1 with unfertilized treatment; SWN360 stand for water salinity of 8.04 dS m−1 with fertilized treatment. Each symbol represents the average score of three replicates.
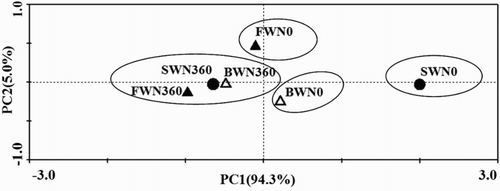
Microbial functional diversity
AWCD has commonly been used to reflect C utilization patterns of rapidly growing bacterial communities and to quantify soil microbial metabolic properties. The AWCD values in this study displayed a sigmoidal pattern in all treatment combinations (). Differences among treatments became greater with incubation time. During the first 24 h, the AWCD values were similar regardless of treatment. The AWCD values increased rapidly (log phase) between 24 and 96 h. Thereafter, growth was steady (stationary phase) until the end of the incubation at 120 h. In the unfertilized plots (N0), the AWCD values were higher in the BW treatment than in the FW treatment during the first 84 h of the incubation. The AWCD values were lowest in the SW treatment. In the fertilized plots (N360), the AWCD values were highest in the FW treatment, followed by the FW treatment and then the SW treatment.
Figure 2. AWCD during 120-h incubations. Values are the mean of three replicates. Error bars represent standard deviations. The symbols FW, BW, and SW represent irrigation water with EC of 0.35, 4.61, and 8.04 dS m−1, respectively. The symbols N0 and N360 represent the 0 and 360 kg N ha−1 treatments, respectively.
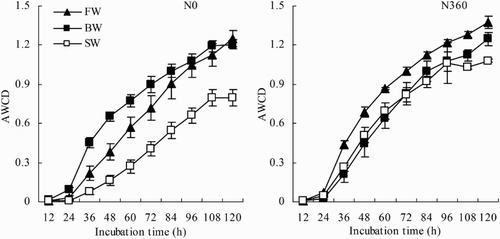
The effects of irrigation water salinity and N fertilization on C source utilization are shown in . In the FW and BW treatments, the most utilized compounds were carbohydrates, amines, amino acids, and polymers. The least used compounds were phenolic acids and carboxylic acid. In the SW treatments, the most utilized compounds were carbohydrates, amino acids, and polymers. The least used compounds were amines, carboxylic acid, and phenolic acid.
Figure 3. Carbon utilization profiles (72 h) as affected by irrigation water salinity and N fertilization. The symbols FW, BW, and SW represent irrigation water with EC of 0.35, 4.61, and 8.04 dS m−1, respectively. The symbols N0 and N360 represent the 0 and 360 kg N ha−1 treatments, respectively. Additional abbreviations: CH, carbohydrates; AM, amines; AA, amino acids; PM, polymers; CA, carboxylic acid; and PA, phenolic acid.
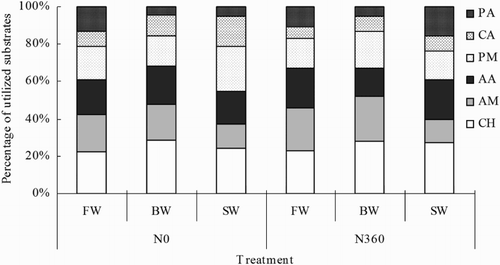
PCA of the optical density values measured at 72 h of incubation could clearly separate the soil samples according to the different treatments (). The first two principal components (PC1 and PC2) explained 91.7% of the variation. This indicated that there were significantly different patterns of potential carbon utilization and microbial communities differing in functional diversity among different irrigation water salinity and N rate treatments. There were four clusters: (1) FWN0 and SWN360; (2) FWN360; (3) SWN0; and (4) BWN0 and BWN360.
Figure 4. PCA of carbon utilization profiles (72 h) as affected by irrigation water salinity and N fertilization. PC1 described 76.9% and PC2 14.8% of variation in the data. Abbreviations: FWN0 stand for water salinity of 0.35 dS m−1 with unfertilized treatment; FWN360 stand for water salinity of 0.35 dS m−1 with fertilized treatment; BWN0 stand for water salinity of 4.61 dS m−1 with unfertilized treatment; BWN360 stand for water salinity of 4.61 dS m−1 with fertilized treatment; SWN0 stand for water salinity of 8.04 dS m−1 with unfertilized treatment; SWN360 stand for water salinity of 8.04 dS m−1 with fertilized treatment. Each symbol represents the average score of three replicates.
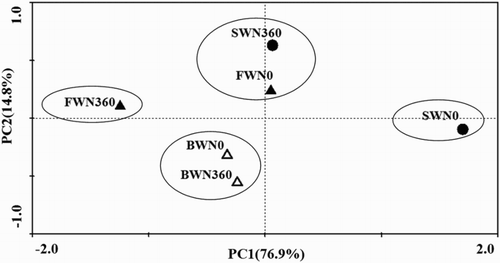
Richness, Shannon, Evenness, and Simpson indices were calculated to describe the diversity of C source utilization. Richness was significantly affected by irrigation water salinity, but not by N rate or by water salinity × N rate interaction (). Richness values were generally highest in the FW treatments. However, the differences between the FW and BW treatments were not significant. The Shannon and Simpson indices were all significantly affected by irrigation water salinity, N fertilization, and their interaction. The Shannon index was generally highest in the FW treatments and lowest in the SW treatments. The Simpson index was generally highest in the SW treatments. The Evenness index was significantly affected by N rate and by water salinity × N rate interaction. Averaged across all three water salinities, the Shannon and Evenness indexes were greater in the N360 treatment than in the N0 treatment. In contrast, the Simpson index was greatest in the N0 treatment.
Table 5. Diversity indices of microbial C source utilization as affected by irrigation water salinity and N fertilization.
Discussion
SW irrigation for five years significantly altered the physicochemical properties of this arid soil (). Many previous reports indicate that irrigation with saline or BW increases soil salt concentrations (Kang et al. Citation2010; Pang et al. Citation2010). Soil water contents generally increase under SW irrigation because saline conditions reduce plant water uptake (Romero-Aranda et al. Citation2001; Malash et al. Citation2008). Soil OM contents may decrease because plant growth declines, resulting in a decrease in organic inputs to soil (Rietz & Haynes, Citation2003). Soil NH4+–N concentrations can be greater in salt-affected soil than in normal soil because nitrification is reduced by high salt concentrations (Santoro et al, Citation2008; Bernhard & Bollmann Citation2010).
As determined by PLFA, SW irrigation reduced total microbial biomass, bacterial biomass, fungal biomass, and the fungal:bacterial ratio (). Several researchers have reported that soil microbial biomass and basal respiration decrease as soil salinity increases because of osmotic stress (Egamberdieva et al. Citation2010; Setia et al. Citation2011; Iwai et al. Citation2012; Baumann & Marschner, Citation2013; Mavi & Marschner Citation2013; Li et al., Citation2014). Mamilov et al. (Citation2004) reported that the slower transformation of organic substrates in saline soils is due to reduced microbial biomass and restricted microbial metabolism. Chowdhury et al. (Citation2011) suggested that the fungal:bacterial ratio decreased with decreasing soil salinity because fungi are more sensitive to salinity than bacteria. The change in the fungal:bacterial ratio may influence soil OM decomposition. Fungi are able to decompose recalcitrant biomolecules such as lignin, whereas bacteria rapidly decompose more easily decomposable material (Swift et al. Citation1979).
Long-term irrigation with SW reduced the diversity of the soil microbial community. The AWCD, Richness, and Shannon's Index were lowest and the Simpson index was highest in the SW treatment ( and ). SW irrigation also altered substrate utilization patterns, with carbohydrates, amines, amino acids, and polymers being the most utilized C sources in the FW and BW treatments (). In the SW treatment, carbohydrates, amino acids, and polymers were the most utilized C sources.
Nitrogen fertilization may increase microbial activity (Conde et al. Citation2005), reduce it (Craine et al. Citation2007; Ramirez et al. Citation2010), or have no effect on it (Hobbie & Vitousek Citation2000). In this arid soil, N fertilization for five years increased microbial activity and reduced the negative influence of SW irrigation on soil microbial biomass and community diversity in this arid soil ( and ). Zhang et al. (Citation2008) also observed that N fertilization significantly increased AWCD values. The main explanation is that N fertilization increased cotton growth and organic inputs to the soil. Changes in AWCD values due to N application suggest that soil microorganisms vary in their response to irrigation water salinity and N rate. These changes were attributed to the C source utilization of soil microbial communities are more sensitive to irrigation water salinity when in conjunction with N addition.
In conclusion, the results indicated that microbial community and diversity had changed significantly after five years of irrigation with brackish or SW. The new community was dominated by fewer and relatively common microbial species. The changes were generally less dramatic when N fertilizer was applied.
Disclosure statement
No potential conflict of interest was reported by the authors.
Additional information
Funding
References
- Alef K, Nannipieri P. 1995. Methods in applied soil microbiology and biochemistry. London: Academic; p. 214–219.
- Amir S, Merlina G, Pinelli E, Winterton P, Revel JC, Hafidi M. 2008. Microbial community dynamics during composting of sewage sludge and straw studied through phospholipid and neutral lipid analysis. J Hazardous Mat. 159:593–601. doi: 10.1016/j.jhazmat.2008.02.062
- Baumann K, Marschner P. 2013. Effects of salinity on microbial tolerance to drying and rewetting. Biogeochem. 112:71–80. doi: 10.1007/s10533-011-9672-1
- Bernhard AE, Bollmann A. 2010. Estuarine nitrifiers: new players, patterns and processes. Estuarine, Coastal Shelf Sci. 88:1–11. doi: 10.1016/j.ecss.2010.01.023
- Bligh EG, Dyer WJ. 1995. A rapid method of total lipid extraction and purification. Can J Biochem Physiol. 37:911–917. doi: 10.1139/o59-099
- Brookes PC, Landman A, Pruden G, Jenkinson DS. 1985. Chloroform fumigation and the release of soil nitrogen: a rapid direct extraction method to measure microbial biomass nitrogen in soil. Soil Biol Biochem. 17:837–842. doi: 10.1016/0038-0717(85)90144-0
- Buchan D, Moeskops B, Ameloot N, De Neve S, Sleutel S. 2012. Selective sterilisation of undisturbed soil cores by gamma irradiation: effects on free-living nematodes, microbial community and nitrogen dynamics. Soil Biol Biochem. 47:10–13. doi: 10.1016/j.soilbio.2011.12.014
- Chowdhury N, Marschner P, Burns R. 2011. Response of microbial activity and community structure to decreasing soil osmotic and matric potential. Plant Soil. 344:241–254. doi: 10.1007/s11104-011-0743-9
- Conde E, Cardenas M, Ponce-Mendoza A, Luna-Guido ML, Cruz-Mondragón C, Dendooven L. 2005. The impacts of inorganic nitrogen application on mineralization of 14C-labelled maize and glucose, and on priming effect in saline alkaline soil. Soil Biol Biochem. 37:681–691. doi: 10.1016/j.soilbio.2004.08.026
- Craine JM, Morrow C, Fierer N. 2007. Microbial nitrogen limitation increases decomposition. Ecology. 88:2105–2113. doi: 10.1890/06-1847.1
- Egamberdieva D, Renella G, Wirth S, Islam R. 2010. Secondary salinity effects on soil microbial biomass. Biol Fertility Soils. 46:445–449. doi: 10.1007/s00374-010-0452-1
- Elgharably A, Marschner P. 2011. Microbial activity and biomass and N and P availability in a saline sandy loam amended with inorganic N and lupin residues. Eur J Soil Biol. 47:310–315. doi: 10.1016/j.ejsobi.2011.07.005
- Esmaili E, Kapourchal SA, Malakouti MJ, Homaee M. 2008. Interactive effect of salinity and two nitrogen fertilizers on growth and composition of sorghum. Plant, Soil Environ. 54: 537–546.
- Frostegård Å, Tunlid A, Bååth E. 1993. Phospholipid fatty acid composition, biomass, and activity of microbial communities from two soil types experimentally exposed to different heavy metals. Appl Environ Microbiol. 59:3605–3617.
- Garland JL, Mills AL. 1991. Classification and characterization of heterotrophic microbial communities on the basis of patterns of community-level sole-carbon-source utilization. Appl Environ Microb. 57:2351–2359.
- Ghollarata M, Raiesi F. 2007. The adverse effects of soil salinization on the growth of Trifolium alexandrinum L. and associated microbial and biochemical properties in a soil from Iran. Soil Biol Biochem. 39:1699–1702. doi: 10.1016/j.soilbio.2007.01.024
- Hai L, Li XG, Li FM, Suo DR, Guggenberger G. 2010. Long-term fertilization and manuring effects on physically-separated soil organic matter pools under a wheat–wheat–maize cropping system in an arid region of China. Soil Biol Biochem. 42:253–259. doi: 10.1016/j.soilbio.2009.10.023
- Harris JA. 2003. Measurements of the soil microbial community for estimating the success of restoration. Eur J Soil Sci. 54:801–808. doi: 10.1046/j.1351-0754.2003.0559.x
- He JZ, Ge Y, Xu ZH, Chen CR. 2009. Linking soil bacterial diversity to ecosystem multifunctional using backward-elimination boosted trees analysis. J Soils Sediments. 9:547–554. doi: 10.1007/s11368-009-0120-y
- Hobbie SE, Vitousek PM. 2000. Nutrient limitation of decomposition in Hawaiian forests. Ecology. 81:1867–1877. doi: 10.1890/0012-9658(2000)081[1867:NLODIH]2.0.CO;2
- Iwai CB, Oo AN, Topark-ngarm B. 2012. Soil property and microbial activity in natural salt affected soils in an alternating wet–dry tropical climate. Geoderma. 189:144–152. doi: 10.1016/j.geoderma.2012.05.001
- Jin ZZ, Lei JQ, Li SY, Xu XW. 2014. Characteristics of sandy soil microbial metabolisms in the forests drip irrigation with saline water. J Desert Res. 34:363–370. (In Chinese)
- Kang Y, Chen M, Wan S. 2010. Effects of drip irrigation with saline water on waxy maize (Zea mays L. var. ceratina Kulesh) in North China Plain. Agri Water Manage. 97:1303–1309. doi: 10.1016/j.agwat.2010.03.006
- Lee JA, Caporn SJM. 1998. Ecological effects of atmospheric reactive nitrogen deposition on semi-natural terrestrial ecosystems. New Phytologist. 139:127–134. doi: 10.1046/j.1469-8137.1998.00165.x
- Li X, Jiao Y, Yang MD. 2014. Diversity of soil microbial communities under different soil salinity levels analyzing by PLFA. Advanced Mat Res. 955:314–320.
- Malash NM, Flowers TJ, Ragab R. 2008. Effect of irrigation methods, management and salinity of irrigation water on tomato yield, soil moisture and salinity distribution. Irr Sci. 26:313–323. doi: 10.1007/s00271-007-0095-7
- Mamilov A, Dilly OM, Mamilov S, Inubushi K. 2004. Microbial eco-physiology of degrading Aral Sea wetlands: consequences for C-cycling. Soil Sci Plant Nutr. 50:839–842. doi: 10.1080/00380768.2004.10408544
- Marschner P, Kandeler E, Marschner B. 2003. Structure and function of the soil microbial community in a long-term fertilizer experiment. Soil Biol Biochem. 35:453–461. doi: 10.1016/S0038-0717(02)00297-3
- Mavi MS, Marschner P. 2013. Salinity affects the response of soil microbial activity and biomass to addition of carbon and nitrogen. Soil Res. 51: 68–75. doi: 10.1071/SR12191
- Nannipieri P, Ascher J, Ceccherini M, Landi L, Pietramellara G, Renella G. 2003. Microbial diversity and soil functions. Eur J Soil Sci. 54:655–670. doi: 10.1046/j.1351-0754.2003.0556.x
- Pang HC, Li YY, Yang JS, Liang YS. 2010. Effect of brackish water irrigation and straw mulching on soil salinity and crop yields under monsoonal climatic conditions. Agri Water Manage. 97:1971–1977. doi: 10.1016/j.agwat.2009.08.020
- Pankhurst CE, Yu S, Hawke BG, Harch BD. 2001. Capacity of fatty acid profiles and substrate utilization patterns to describe differences in soil microbial communities associated with increased salinity or alkalinity at three locations in South Australia. Biol Fertility Soils. 33:204–217. doi: 10.1007/s003740000309
- Pereira LS, Oweis T, Zairi A. 2002. Irrigation management under water scarcity. Agri Water Manage. 57:175–206. doi: 10.1016/S0378-3774(02)00075-6
- Ramirez KS, Craine JM, Fierer N. 2010. Nitrogen fertilization inhibits soil microbial respiration regardless of the form of nitrogen applied. Soil Biol Biochem. 42:2336–2338. doi: 10.1016/j.soilbio.2010.08.032
- Rietz DN, Haynes RJ. 2003. Effects of irrigation-induced salinity and sodicity on soil microbial activity. Soil Biol Biochem. 35:845–854. doi: 10.1016/S0038-0717(03)00125-1
- Romero-Aranda R, Soria T, Cuartero J. 2001. Tomato plant-water uptake and plant-water relationships under saline growth conditions. Plant Sci. 160:265–272. doi: 10.1016/S0168-9452(00)00388-5
- Santoro AE, Francis CA, De Sieyes NR, Boehm AB. 2008. Shifts in the relative abundance of ammonia-oxidizing bacteria and archaea across physicochemical gradients in a subterranean estuary. Environ Microbiol. 10:1068–1079. doi: 10.1111/j.1462-2920.2007.01547.x
- Sarathchandra SU, Ghani A, Yeates GW, Burch G, Cox NR 2001. Effect of nitrogen and phosphate fertilisers on microbial and nematode diversity in pasture soils. Soil Biol Biochem. 33:953–964. doi: 10.1016/S0038-0717(00)00245-5
- Setia R, Marschner P, Baldock J, Chittleborough D. 2010. Is CO2 evolution in saline soils affected by an osmotic effect and calcium carbonate? Biol Fertility Soils. 46:781–792. doi: 10.1007/s00374-010-0479-3
- Setia R, Marschner P, Baldock JS, Chittleborough DJ, Verma V. 2011. Relationships between carbon dioxide emission and soil properties in salt affected landscapes. Soil Biol Biochem. 43:667–674. doi: 10.1016/j.soilbio.2010.12.004
- Shen W, Lin X, Gao N, Zhang H, Yin R, Shi W, Duan Z. 2008. Land use intensification affects soil microbial populations, functional diversity and related suppressiveness of cucumber Fusarium wilt in China's Yangtze River Delta. Plant Soil. 306:117–127. doi: 10.1007/s11104-007-9472-5
- Shen W, Lin X, Shi W, Min J, Gao N, Zhang H, Yin R, He X. 2010. Higher rates of nitrogen fertilization decrease soil enzyme activities, microbial functional diversity and nitrification capacity in a Chinese polytunnel greenhouse vegetable land. Plant Soil. 337:137–150. doi: 10.1007/s11104-010-0511-2
- Swift MJ, Heal OW, Anderson JM. 1979. Decomposition in terrestrial ecosystems. Oxford: Blackwell Scientific Publications.
- Vance ED, Brookes PC, Jenkinson DS. 1987. An extraction method for measuring soil microbial biomass C. Soil Biol Biochem. 19:703–707. doi: 10.1016/0038-0717(87)90052-6
- Villa-Castorena M, Ulery AL, Catnlan-Valencia EA, Remmenga MD. 2003. Salinity and nitrogen rate effects on the growth and yield of Chile pepper plants. Soil Sci Soc Am J. 67:1781–1789. doi: 10.2136/sssaj2003.1781
- Wichern J, Wichern F, Joergensen RG. 2006. Impact of salinity on soil microbial communities and the decomposition of maize in acidic soils. Geoderma. 137:100–108. doi: 10.1016/j.geoderma.2006.08.001
- Yan N, Marschner P. 2012. Response of microbial activity and biomass to increasing salinity depends on the final salinity, not the original salinity. Soil Bio Biochem. 53:50–55. doi: 10.1016/j.soilbio.2012.04.028
- Yuan BC, Li ZZ, Liu H, Gao M, Zhang YY. 2007. Microbial biomass and activity in salt affected soils under arid conditions. Appl Soil Ecol. 35:319–328. doi: 10.1016/j.apsoil.2006.07.004
- Zak JC, Willig MR, Moorhead DL, Wildman HG. 1994. Functional diversity of microbial communities: a quantitative approach. Soil Biol Biochem. 26:1101–1108. doi: 10.1016/0038-0717(94)90131-7
- Zak DR, Pregitzer KS, Curtis PS, Holmes WE. 2000. Atmospheric CO2 and the composition and function of soil microbial communities. Ecol Appl. 10:47–59.
- Zelles L. 1997. Phospholipid fatty acid profiles in selected members of soil microbial communities. Chemosphere. 35:275–294. doi: 10.1016/S0045-6535(97)00155-0
- Zhang N, Wan S, Li LH, Bi J, Zhao MM, Ma KP. 2008. Impacts of urea N addition on soil microbial community in a semi-arid temperate steppe in northern China. Plant Soil. 311:19–28. doi: 10.1007/s11104-008-9650-0
- Zornoza R, Guerrero C, Mataix-Solera J, Scow KM, Arcenegui V, MataixBeneyto J. 2009. Changes in soil microbial community structure following the abandonment of agricultural terraces in mountainous areas of Eastern Spain. Appl Soil Ecol. 42:315–323. doi: 10.1016/j.apsoil.2009.05.011