Abstract
This paper describes the modern enzymology in Japanese bioindustries. The invention of Takadiastase by Jokiti Takamine in 1894 has revolutionized the world of industrial enzyme production by fermentation. In 1949, a new γ-amylase (glucan 1,4-α-glucosidase, EC 3.2.1.3) from A. luchuensis (formerly designated as A. awamori), was found by Kitahara. RNase T1 (guanyloribonuclease, EC 3.1.27.3) was discovered by Sato and Egami. Ando discovered Aspergillus nuclease S1 (single-stranded nucleate endonuclease, EC 3.1.30.1). Aspergillopepsin I (EC 3.4.23.18) from A. tubingensis (formerly designated as A. saitoi) activates trypsinogen to trypsin. Shintani et al. demonstrated Asp76 of aspergillopepsin I as the binding site for the basic substrate, trypsinogen. The new oligosaccharide moieties Man10GlcNAc2 and Man11GlcNAc2 were identified with α-1,2-mannosidase (EC 3.2.1.113) from A. tubingensis. A yeast mutant compatible of producing Man5GlcNAc2 human compatible sugar chains on glycoproteins was constructed. The acid activation of protyrosinase from A. oryzae at pH 3.0 was resolved. The hyper-protein production system of glucoamylase was established in a submerged culture.
Graphical abstract
Proposed structures of the new oligosaccharides (Man10GlcNAc237) [A] and Man11GlcNAc238) [B]) of Aspergillus serine carboxypeptidase.
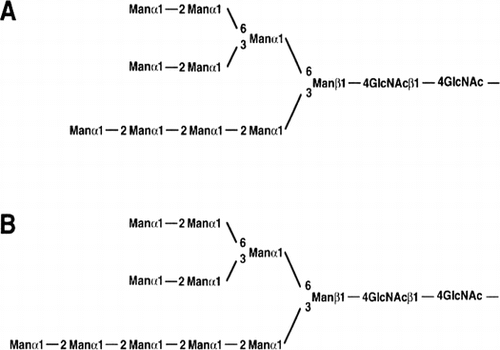
The fungus Aspergillus oryzaeCitation1) is one of the oldest and frequently used micro-organisms in Japanese bioindustries. Over the past 1,000 years, the use of hydrolytic enzymes from fungi has become more prevalent in Japanese fermentation industries. Almost all characteristic flavors and tastes of these traditional fermented foods are introduced by traditional solid fermentation of malted rice and/or soy bean using A. oryzae and A. sojae.Citation2,3) A. luchuensis,Citation4–6) an industrially important black Aspergillus in East Asia, is also used for the production of Japanese traditional spirits, citric acid, and other fermented beverages. The study of enzymes from Aspergillus fungi remains of great importance to the scientific community and to society in Japan.
The invention of Takadiastase by Jokiti Takamine in 1894 has revolutionized the world of industrial enzyme production by fermentation.Citation7,8) Sirou Akabori developed the study of of α-amylase (EC 3.2.1.1) from A. oryzae (Taka-amylase A).Citation9,10) In 1949, a new γ-amylase from A. luchuensis (A. awamori), which is now classified as glucoamylase (glucan 1,4-α-glucosidase, EC 3.2.1.3), was found by Kitahara and Kurushima.Citation11) The raw starch affinity site of glucoamylase I from A. luchuensis (A. awamori var. kawachii) was determined by Hayashida et al.Citation12,13) and Goto et al.Citation14,15)
RNase T1 (guanyloribonuclease, EC 3.1.27.3) was discovered by Sato and Egami.Citation16) AndoCitation17) discovered Aspergillus nuclease S1 (single-stranded nucleate endonuclease, EC 3.1.30.1).
A new zinc-binding motif aspzincinCitation18) (HEXXH + D) was found in deuterolysinCitation19) (microbial neutral protease II, EC 3.4.24.39). Four of the 10 glutaminases were involved in glutamate production in soy sauce and the peptideglutaminase reaction was very important for enhancing glutamate.Citation20–23)
This review also includes our results. Perhaps one of the most exciting fields of modern enzymology is the application of the enzyme technology. Shintani et al. demonstrated Asp76 of aspergillopepsin ICitation24–29) (EC 3.4.23.18) from A. tubingensis as the binding site for the basic substrate, trypsinogen, and that a single amino acid mutation (D76S) is sufficient to alter the substrate specificity of the enzyme for trypsinogen.Citation30) Aorsin (EC 3.4.21.-) from A. oryzae has a catalytic triad, Glu86, Asp211, and Ser354, and is not inhibited by pepstatin, a specific inhibitor of pepsin.Citation31) Serine carboxypeptidaseCitation32–36) (acid carboxypeptidase, EC 3.4.16.5) of A. tubingensis released lysine, glutamic acid, and leucine from the anticoagulant decapeptide (SQLQEAPLEK) by a single enzyme.Citation35)
The new oligosaccharide moieties Man10GlcNAc2Citation37) and Man11GlcNAc2Citation38) of serine carboxypeptidase from A. tubingensis were identified with a new α-1,2- mannosidaseCitation39,40) (EC 3.2.1.113) from A. tubingensis. Tatara et al.Citation41) identified catalytic residues of Ca2+-independent α-1,2-mannosidase from A. tubingensis by site-directed mutagenesis. A yeast mutant compatible of producing Man5GlcNAc2 human compatible sugar chains on glycoproteins was constructed by Chiba et al.Citation42)
The acid activation of protyrosinaseCitation43–45) from A. oryzae to tyrosinase (EC 1.14.18.1, EC 1.10.3.1) at pH 3.0 is a unique mechanism, in which the tetrameric protyrosinase is dissociates to dimers. The dimerization of acid-activated tyrosinase involving the intermolecular disulfide bond is essential for the activity.
The hyper-protein production system of glucoamylase was established in a submerged culture.Citation46) The glaB-type glucoamylase was produced under the control of the tyrosinase encoding gene, melO,Citation44) promoter.
This review discusses the catalytic and molecular properties of unique and characteristic enzymes from Aspergillus fungi in Japanese bioindustries.
I. Results obtained using A. oryzae, A. sojae, and A. luchuensis
I.i. The national fungi of Japan
The genome of A. oryzae was sequenced in 2005.Citation1) And, the genome of A. sojae was sequenced in 2011.Citation3) Since Inui reported Aspergillus luchuensis from black koji in Okinawa in 1901, many fungal names associated with koji molds were reported.Citation4–6) Recently, multi-locus sequence typing has been successfully used to taxonomy of black Aspergillus. According to β-tubulin and calmodulin gene sequences, black koji molds can be subdivide in three species, A. luchuensis, A. niger, and A. tubingensis. A. saitoi and A. saitoi var. kagoshimaensis are synonyms of A. tubingensis. A.luchuensis mut. kawachii was suggested particular name for A. kawachii became of their industrial importance.
In 2006, the Brewing Society of Japan authorized the certification of “A. oryzae, A. sojae and A. luchuensis are the national fungi of Japan.”Citation47)
I.ii. Carbohydrases
The year of 1894 saw the true beginnings of modern microbial enzyme technology, with Takadiastase (Table ), a rather crude mixture of hydrolytic enzymes prepared by growing A. oryzae on wheat bran, being marketed in the West for the first time. The major glucosidases of A. oryzae are amylases, α-amylase, and glucoamylase, which break down starch.
Table 1. Characteristic glycosidases obtained from A. oryzae, A. luchuensis, and A. tubingensis.
By 1951 Akabori established the crystallizationCitation9,10) of α-amylase (EC 3.2.1.1) from A. oryzae (Taka-amylase A) (Table ). For Taka-amylase A, the amino acid sequenceCitation48) and the nucleotide sequenceCitation49) were determined, respectively. Studies were soon followed by determination of molecular structure.Citation50,51) Matsuura et al.Citation51) reported that a complete molecular structure model of Taka-amylase A consisting of 478 amino acid residues was built with the aid of amino acid sequence data. On the basis of the difference Fourier analysis and the model fitting study, glutamic acid (Glu-230) and aspartic acid (Asp-297); which are located at the bottom of the cleft, were concluded to be the catalytic residues, serving as the general acid and base, respectively. For additional study, the Institute for Protein Research Osaka University was founded formally on April 1 1958, as a part of Osaka University, and Professor Shiro Akabori (1900–1992) was appointed as its first director. Akabori made an important contribution to Japanese enzymology.
Aspergillus oryzae produces large quantities of a glucoamylase (α-1,4-glucan glucanohydrolase, EC 3.2.1.3) (Table ) in solid-state cultures. Nucleotide sequence of an alternative glucoamylase-encoding gene (glaB) expressed in solid-state culture of A. oryzae was reported by Hata et al.Citation52) A cDNA for A. oryzae glucoamylase was cloned.Citation53) The complete nucleotide sequence of the cDNA contained an open reading frame encoding 612 amino acid residues. Comparative studies with other fungal glucoamylases showed homologies of 67% with A. niger and 30% with Rhizopus oryzae of the deduced amino acid sequences. In the five conserved regions reported in other fungal glucoamylases, the levels of homologies between those regions of A. oryzae and A. niger enzymes were much higher (78–94%). A. oryzae glucoamylase contained no peptide region abundant in threonine and serine residues (TS-region), like that proposed to absorb onto raw starch in A. luchuensis mut. kawachii (A. awamori var. kawachii) glucoamylase.Citation53)
Two extracellular glucoamylases (EC 3.2.1.3) of A. oryzae were purified from solid-state culture (S-GA) and from submerged culture (L-GA). The two glucoamylases have different molecular masses, 65 kDa for L-GA; 63–99 kDa for S-GA, and different isoelectric points; 4.2 for L-GA; 3.9 for S-GA. Almost all of the enzymatic characteristics of the two glucoamylases were similar, except for thermal stability, initial reaction velocity on pullulan and Km value with soluble starch. Although L-GA could digest raw starch, S-GA demonstrated little activity with raw starch. Peptide mapping and amino acid composition showed that L-GA must be encoded by the glaA gene previously cloned as the glucoamylase-encoding gene from A. oryzae, but S-GA had a different primary structure than the deduced glaA product. Introduction of multiple copies of the glaA gene to A. oryzae caused on elevation of glucoamylase productivity of transformant in submerged culture but not in solid-state culture. These results suggested that the two forms of glucoamylases aries from different genes rather than result from proteolytic processing after polypeptide synthesis of a single protein.Citation54)
The A. oryzae glucoamylase gene glaB is expressed specifically and strongly only during solid-state condition. To elucidate the basis for specificity, the glaB promoter was analyzed by electrophoretic gel mobility shift assay. Two protein-binding elements were estimated to be -382 to -353 and -332 to -313, respectively. To confirm that these regions contained cis-elements, deletion analysis of the promoter was undertaken using β-glucuronidase as a reporter. The results of deletion analysis were consistent with the electrophoretic gel mobility shift assay. The promoter missing the -332–-313 element was not induced by low water activity stress during solid-state condition.Citation55)
Regarding the amylase system of A. luchuensis (A. awamori), the following facts are described by Ueda.Citation56) The dextrogenic amylase has extremely weak action on raw starch, independent of the presence or absence of maltase activity, as compared with the saccharogenic amylase fraction. The saccharogenic and dextrogenic amylase fractions interact each other to promote digestion of raw starch up to about three-times sum of the activity of each fraction. The accelerating-interaction effect solely depends upon the debranching activity of saccharogenic amylase fraction. Raw starch digestion by glucoamylase (EC 3.2.1.3) from Aspergillus is a useful method to economize oil, because the Japanese Government urged the nation to economize oil.
The raw starch affinity site of glucoamylase I from A. luchuensis mut. kawachii (A. awamori var. kawachii) was determined by Hayashida et al.Citation12,13) The enzymatically inactive but raw starch-absorbable glycopeptidase-I (Gp-I), which was obtained from raw starch-absorbable, raw starch-digesting glucoamylase I (GAI) of A. luchuensis mut kawachii as a result of proteolysis with subtilisin and proved to contain the raw starch affinity site essential for raw starch digestion, was found to promote the digestion rate of raw corn starch with GAI, maximally to the extent of 2.5 times. The raw starch absorbability of Gp-I was decreased by the partial removal of carbohydrate moiety from Gp-I. The carbohydrate-split GAI could hydrolyze gelatinized substrates almost at the same rate as the native one, but significantly decreased in the absorbability and digestibility onto raw starch in composition with the original one. From the functional and characteristic structure of the parallel short mannoside chains linked to the successive sequence of hydroxyl amino acid residues of Gp-I, the “water-cluster-dissociating model” for the hypothetical mechanism of raw starch digestion by the adsorption of GAI at the affinity site onto starch was proposed.Citation12) The raw starch affinity site of A. luchuensis mut. kawachii glucoamylase I (GAI) was found to be located separately from the active site in the region corresponding to Gp-I liberated from the GAI through the action of subtilisin. Gp-I consists of 45 amino acid residues, hydroxyl amino acids being characteristically abundant, and 56 mannose residues. The sequence was determined to be ATGGTTTATTTGSGGVTSTSKTTTTASKTSTTTSSTSCTTPTAV. A structure of parallelly arranged short mannoside chain linked-O-glycosidically to the successive sequence of hydroxyl amino acid residues on Gp-I was revealed. On comparison of the amino acid sequence of Gp-I with those of three glucoamylases, from A. awamori, A. niger, and Rhizupus oryzae, significantly homologous regions (98, 91, and 97% homology, respectively) were detected as the affinity site that could be functionally constructed and essential for raw starch-digesting glucoamylase.Citation13)
Furthermore, Goto et al.Citation14) analyzed the raw starch-binding domain by mutation of a glucoamylase from A. luchuensis mut. kawachii (A. awamori var. kawachii) expressed in Saccharomyces cerevisiae. Functional analysis of O-linked oligosaccharides in threonine/serine-rich region of glucoamylase by expression in mannosyltransferase-disruptant of yeast was performed by Goto et al.Citation15) The glaA gene-encoding glucoamylase (GAI) of A. luchuensis mut. kawachii was heterologous expressed in mannosyltransferase mutants of Saccharomyces cerevisiae, in which the pmt1 gene and the kre2 gene were disrupted. The GAI enzymes expressed in these mutant cells exhibited a lesser of O-glycosilation. Secretion of GAI expressed in the pmt1-disruptant and in the kre2-disruptant, respectively, was almost the same as that of GAI expressed in wild strains. The number of O-linked mannose in GAI from wild-type yeast strains regard in size from one (Man1) to five (Man5). On the other hand, the O-linked oligosaccharides of GAI from the pmt1-disruptant ranged in size from Man1 to Man4. Man5 was not detected and Man2–Man4 were reduced in proportion to the reaction of Man2. The O-linked disaccharides of GAI from the kre2-disruptant ranged from Man1 to Man4, and the molar amount of the Man4 was reduced to 27.3%, compared to that of the wild-type strain. The hydrolyzing abilities for soluble starch and the absorbing abilities on raw starch were comparable between both disruptants and wild-type strains. However, the digesting abilities for raw starch of the disruptants were decreased to 70% of those of the wild-type strains. Stabilities of GAI of the disruptants were reduced toward extreme pH and high temperature, compared to those of the wild-type strains. These results demonstrated that the O-linked oligosaccharides of GAI are responsible for the enzyme stability and activity toward insoluble substrates but not for secretion.
For industrial production of high-fructose corn syrup, basic study of α-amylase and glucoamylase from Aspergillus is also important for the initial steps in hydrolysis of starch (rice, wheat, and corn). Isomeric conversion from d-glucose to d-fructose by d-xylose ketol isomerase (EC 5.3.1.5) is important process. The initial basic research on d-xylose isomerase from Lactobacillus brevis was carried out by Yamanaka.Citation57,58)
Ethyl-α-d-glucoside (Table ), a reaction product of α-glucosidase (EC 3.2.1.20, Table ), is useful skin dermatitis.Citation59) The catalytic reaction mechanisms of carbohydrate hydrolases were discussed by Chiba.Citation60)
Table 2. Characteristic chemicals obtained from A. oryzae.
I.iii. Nucleases
Ribonuclease T1Citation16) (RNase T1 or guanyloribonuclease, EC 3.1.27.3) (Table ) was discovered by Sato and Egami in 1957. RNase T1 is a guanosine-specific ribonuclease that cleaves the 3′,5′-phosphodiester linkage of single strand RNA. Takahashi determined the primary structure of RNase T1.Citation61) Unfolding of RNase T1 by a denaturant, urea, was suppressed in the presence of salts, and the salt-induced chain folding was observed spectrophotometrically even in 6.9 M urea solution.Citation62) The salts also induced the chain folding of disulfide-reduced and modified (carboxymethylated or carboxamidomethylated) RNase T1 into the native conformation, as indicated its spectroscopic properties, but did not restore the enzymatic activity.Citation62) Such effects of salts in enhancing the stability of the protein conformation have not been observed for pancreatic RNase A (EC 3.1.27.5),Citation63) suggesting that the conditions for correct folding of a polypeptide chain are different from protein. Nishikawa et al.Citation64) reported that mutant of RNase T1 with residue Ala-40 or Ala-92 have almost no activity, while mutants that contain Ala-58 retain considerable activity. These results show that the two histidine residues, His-40 and His-92, but not Glu-58 are indispensable for the catalytic activity of the enzyme. They proposed a revised reaction mechanism in which two histidine residues play a major role as they in the case of RNase A. Protein engineering has been used to identify the residues that contribute to RNase T1-catalyzed transesterification.Citation65)
Table 3. Characteristic endoribonuclease and endonuclease obtained from A. oryzae.
For commercial enzyme, RNase T1 is an endoribonuclease that specifically cuts RNA or denatured RNA at the 3′-end of guanosine and adjacent nucleotides through a 2′,3′-cyclic phosphate intermediate mechanism. Unlike RNase A which degrades RNA through cleavage at pyrimidine residues, or RNase I which cleaves at many sites along the RNA molecule, RNase T1 specifically cuts RNA molecule at guanine residues, making it suitable for assay where base-specific cleavage in desirable. RNase T1 is cloned from A. oryzae and overexpressed in E. coli to produce a high pure enzyme without contaminating nuclease activities. The enzyme is active in a broad range of reaction buffer.
In 1966, AndoCitation17) discovered Aspergillus nuclease S1 (single-stranded nucleate endonuclease, EC 3.1.30.1) (Table ). SuttonCitation66) found that a crude preparation of nuclease S1 from A. oryzae is shown to have a strong preference for heat-denatured DNA as substrate, to be active over a range of ionic strengths, to degrade a variety of a single-strand DNA’s to better than 97% acid-soluble materials, and to be capable for removing the single-strand region from ressociated DNA molecules at a range of temperatures without extensively degrading the ressociated duplex regions. Nuclease S1 displays anomalous reaction kinetics at low substrate concentrations. Aspergillus nuclease S1 is specifically catalyzes endonucleolytic cleavage to 5′-phosphomononucleotide and 5′-phososphooligonucleotide end products. Purification and further properties of single-strand-specific nuclease from A. oryzae were reported by Vogt.Citation67) Penicillium citrinum nuclease P1 (EC 3.1.30.1) is a similar enzyme. Volbed et al.Citation68) reported crystal structure of Penicillium citrinum nuclease P1 at 2.5 A resolution.
BeckCitation69) recorded Aspergillus nuclease S1 in “A Chronology of Microbiology in Historical Context.” Tools of molecular biology for recombinant DNA are the enzymes that cut and join DNA molecules, respectively, called restriction enzymes and ligases. Nuclease S1 is also useful enzyme for recombinant DNA technology. Aspergillus nuclease S1 is also commercial enzyme. Although its primary substrate is single-stranded, it can also occasionally introduce single-stranded breaks in double-stranded DNA or RNA, or DNA–RNA hybrids. The enzyme hydrolyzes single-stranded region in duplex DNA such as loops of gaps.
I.iv. Proteases and glutaminases
Two typical koji molds, A. sajae and A. oryzae, are used. During the fermentation process, koji molds act by breaking down the ingredients. Each species of koji molds reacts differently to the ingredients used and must, therefore, be selected based on the desired product. For example, A. sojaeCitation2,3) is selected to produce miso and soy sauce due to its high proteolytic ability, and A. oryzae is used widely in sake, miso, and soy sauce production for its high amylolytic ability.
Sato et al.Citation3) conducted genome sequencing of A. sojae NBRC 4239 isolated from the solid-state culture used to prepare Japanese soy sauce. Of the 2847 open-reading frames with Pfam domain score of >150, 81% had a high degree of similarity with the genes of A. oryzae RIB 40. Comparative analysis identified serine carboxypeptidase (EC 3.4.16.5) (Table ) and aspergillopepsin I (EC 3.4.23.18) (Table ) genes unique to A. soaje NBRC 4239. A. sojae NBRC 4239 had 13 serine carboxypeptidases, which was one more than A. oryzae RIB 40, and it had 7 aspartic proteases (aspergillopepsin I) which was 2 less than A. oryzae RIB 40. While A. oryzae possessed three copies of α-amylase (EC 3.2.1.1) gene, A. sojae NBRC 4239 possessed only a single copy. In A. oryzae, amyB codes for so-called Taka-amylase A, which is important for amylolysis. Therefore, a decreased copy number of amyB orthologues in A. sojae likely accounts for the lower amylolytic ability of A. sojae than that of A. oryzae.
Table 4. Characteristic proteolytic enzymes obtained from A. oryzae, A. sojae, A. luchuensis, and A. tubingensis.
DeuterolysinCitation18,19) (EC 3.4.24.39; formerly designated as neutral protease II) (Table ) from A. oryzae and A. sojae, which contains 1 g atom of zinc/mol of enzyme, is a single chain of 177 amino acid residues, including three disulfide bonds, and has a molecular mass of 19,018 Da. Active-site determination of the recombinant enzyme expressed in Escherichia coli was performed by site-directed mutagenesis. Substitutions of His-128 and His-132 with Arg, of Glu-129 with Gln or Asp, of Asp-143 with Asn or Gln, of Asp-164 with Asn, and Tyr-106 with Phe resulted in almost complete loss of the activity of the mutant enzymes. It can be concluded that His-128, His-132, and Asp-164 provide the Zn2+ ligands of the enzyme according to a 65Zn binding assay. Based on site-directed mutagenesis experiments, it was demonstrated that the three essential amino acid residues Glu-129, Asp-143, and Tyr-106 are catalytically crucial residues in the enzyme. Glu-129 may be implicated in a central role in the catalytic function. Fushimi et al.Citation18) concluded that deuterolysin is a member of a family of Zn2+-metallo-endopeptidase with a new zinc-binding motif, aspzincin, defined by the “HEXXH + D” motif and as aspartic acid as the third zinc ligand.
Deuterolysin is extreamly stable at 100 °C for 10 min, but relatively unstable around 75 °C. Deuterolysin has high activity toward peptides next to a pair of basic residues (Lys–Arg, Arg–His, Arg–Lys, and Arg–Arg) in histone H4 of the calf thymus.Citation70)
PenicillolysinCitation71,72) from Penicillium citrinum is a member of the clan MX and the family of M35 proteases. Penicillolysin is a thermolabile and its activity is almost completely lost above 66 °C. Penicillolysin shows 68% sequence identity with that of deuterolysin.Citation72) Doi et al.Citation73) had expressed recombinant penicillolysin in A. oryzae and generated several site-directed mutants, R33E/E60R, A167E, and T81P, exploring thermal stabilization of penicillolysin. For thermal stabilization of penicillolysin, they expected that on the polar surface of the helix, electrostatic interaction between acidic and basic residues at Glu-33 and Arg-60 would copy the N-terminal sequence of deuterolysin. They generated the mutant, R33E/E60R. For A167E, they expected penicillolysin to have a hydrogen bond between the acidic residue Glu at position 167 and Thr at position 125. They therefore generated the mutant A167E. Generally, proline residues have less conformational freedom in unfolded structures than any other residues since the proline side chain is fixed by a covalent bond to the main chain. From the structure of deuterolysin, it is evident that three proline residues, Pro-81, Pro-87, and Pro-112, are present in the molecule, but not in penicillolysin. Pro-81 and pro-87 are located in the loop region between β3 and β4, and Pro-112 is located in the loop region between αD and αE. They decided on the position and species of amino acids to be replaced to give enhanced thermal stability. They generated those mutants T81P, T87P, and A112P. The resulting mutant proteins exhibited comparable catalytic efficiency to the wild-type enzyme and some showed a higher tolerance to temperature.Citation73)
Aspergillus alkaline proteinase (orizin, aspergillopeptidase B, EC 3.4.21.63) (Table ) is a predominant extracellular endopeptidase of A. oryzaeCitation74,75) and A. sojae.Citation76) Isolation and characterization of the alkaline protease gene of A. oryzae were reported by Murakami et al.Citation77)
l-Glutamate (monosodium glutamate) is the most important taste and flavor component in Japanese traditional soy sauce.Citation78) In soy sauce fermentation, l-glutamate is produced via 2 pathways: [i] direct release from material proteins by proteases and/or peptidases, and [ii] hydrolysis of free-l-glutamine released from material proteins by glutaminases (EC 3.5.1.2). The latter is more important for enhancing taste during soy sauce fermentation because L-glutamine can be converted to pyroglutamate, which has no taste.Citation20)
Recently, 10 genes encoding proteins with glutaminase activity were identified in A. sojae genome; one of the encoded proteins, GahB, acted as the main glutaminase in soy sauce solid-state culture.Citation21) Ito et al.Citation22) also found that 4 of the 10 glutaminases, GahA, GahB, GgtA, and Gls, were involved in glutamate production in soy sauce and that peptidglutaminase reaction of GahA and GahB was very important for enhancing glutamate.Citation22) A. sojae GahA (AsGahA) was localized on the cell wall overexpressing in submerged culture, but was secreted extracellularly in solid-state culture. AsGahA was purified from the cell surface in submerged culture as a complex of three polypeptides A, B, and C.Citation23)
I.v. Cutinase
The fungal cell wall is a complex structure that is essential for the maintenance of cellular shape and integrity, prevention of cell lysis, and protection against adverse environmental conditions. Hydrophobins are involved in fungal adhesion to solid surface, the formation of protective surface coating on fungal cells, the reduction of water surface tension, and processes that support the growth of fungal aerial structures such as hyphae and conidiospores.
Hydrophobins are amphiphilic secretory proteins with eight conserved cysteine residues and are ubiquitous among filamentous fungi. Hydrophobins are a group of small (~100 amino acids) cysteine-rich proteins that are expressed only by filamentous fungi. They are known for their ability to form a hydrophobic (water-repellent) coating on the surface of an object.Citation79) Hydrophobin genes involved in formation of aerial hyphae and fruit bodies were discovered in Schizophyllum commune.Citation80)
Maeda et al.Citation81) purified cutinase (EC 3.1.1.74) from A. oryzae, and its molecular mass was determined as 21.6 kDa. Specific activities of against poly-(butylene succinate) (PBS), poly-(butylene succinate-co-adipate) (PBSA), and poly-(lactic acid) (PLA) were determined as 0.42 U/mg, 11 U/mg, and 0.067 U/mg, respectively. In this connection, PLA, PBSA are the most promising materials among commercially available synthetic biodegradable plastics. Biodegradable plastics, including PBS and PBSA, which have excellent properties, have not yet been commonly used. Determination of the affinities for different chemicals indicated that the most preferred substrate for the enzyme would consist of butylic acid and n-hexanol.
The Cys3–Cys4 and Cys7–Cys8 loops of hydrophobins are thought to form hydrophobic segments involved in adsorption of hydrophobins on hydrophobic surface. When the fungus A. oryzae is grown in a liquid medium containing the polyester, polybutylen succinate-co-adipate (PBSA), A. oryzae produces hydrophobin RolA, which attaches to PBSA. Tanaka et al.Citation82) analyzed the kinetics of RolA adsorption on PBSA using a PBSA pull-down assay and a quartz crystal microbalance (QCM) with PBSA-coated electrodes. They constructed RolA mutants in which hydrophobic amino acids in the two loops were replaced with serine, and they examined the kinetics of mutant adsorption on PBSA. Quartz crystal microbalance (QCM) analysis revealed that mutants with replacements in the Cys7–Cys8 loop had lower affinity than wild-type RolA for PBSA, suggesting that this loop is involved in RolA adsorption on PBSA. It can be concluded that the hydrophobic amino acid residues in the Cys7–Cys8 loop of hydrophobins are directly involved in their interaction with the solid materials. The A. oryzae hydrophobin RolA attaches the aliphatic polyester PBSA, then recruits and condenses cutinase CutL1 on the surface. Takahashi et al.Citation83) identified amino acid residues that are required for the RolA–CutL1 interaction. Quartz crystal microbalance (QCM) analysis revealed that negatively charged residues (Asp-142, Asp-171, and Glu-31) of CutL1, and positively charged residues (His-32 and Lys-34) of RolA play crucial roles in the RolA–CutL1 interaction via ionic interactions.
II. Our results for A. oryzae and A. tubingensis
II.i. Aspergillopepsin I, aorsin, and serine carboxypeptidase
An aspartic endopeptidase from A. tubingensis (formerly designated as A. saitoiCitation84)) produced on an industrial scale by us was used in preparation of a human digestant, Molsin, (Fujisawa Pharmaceutical Co., Osaka).Citation85)
Aspergillopepsin I (EC 3.4.23.18) (Table ) was isolated by YoshidaCitation24) from A. tubingensis (A. saitoi), a micro-organism used in fermentation of the traditional Japanese spiritual liquor in Kagoshima prefecture. Aspergillopepsin I from A. tubingensis (A. saitoi R-3813, ATCC 14332) was purified to a high degree.Citation25,26) The specificity of several fungal aspartic endopeptidases toward the tetradecapeptide of a renin substrate was compared and reported by Majima et al.Citation27) Aspergillopepsin I favors hydrophobic amino acid residues in P1 and P1′, but it also accepts Lys in P1, which leads to activation of trypsinogen at acidic pH.Citation28,29)
The selective cleavage by enteropeptidase (enterokinase, EC 3.4.21.9) initiates Lys6-Ile7 bond for trypsinogen. Then trypsin (EC 3.4.21.4) activates other zymogens. Thus, the formation of trypsin by enteropeptidase is the master activation step.
To identify the residue of the substrate binding pocket in determining the specificity of aspergillopepsin I toward basic substrate, this residue was replaced with a serine residue by site-directed mutagenesis.Citation30) The mutation is a single amino acid change, Asp76 converted to Ser76 (D76S), in the enzyme. The striking feature of this is that only the trypsinogen activating activity was destroyed. It can be concluded that Asp76 is the binding site toward basic substrate.Citation30)
On the basis of amino acid sequence alignments of aspartic proteinases, Asp-76 and Ser-78 of aspergillopepsin I are conserved only in fungal enzymes with the ability to activate trypsinogen, and are located in the active-site flap. Site-directed mutants (D76 N, D76E, D76S, D76T, S78A, and ⊿S78) were constructed, overexpressed in Escherichia coli cells and purified for comparative studies using natural and synthetic substrates. Substitution of Asp-76 to Ser or Thr and deletion of Ser-78, corresponding to the mammalian aspartic proteinases, caused drastic decreases in the activities toward substrates containing a basic amino acid residue at P1. In contrast, substrates with a hydrophobic residue at P1 were effectively hydrolyzed by each mutant enzyme. These results demonstrate that Asp-76 and Ser-78 residues on the active-site flap play important roles in the recognition of a basic amino acid residue at the P1 position.Citation86)
The substrate specificity of porcine pepsin (EC 3.4.23.1) has been altered to resemble that of fungal aspartic proteinase with preference for a basic amino acid residue in P1 by site directed mutagenesis.Citation87) On the basis of primary and tertiary structures of aspartic proteinases, the active-site flap mutants of porcine pepsin were constructed, which involved the replacement of Thr-77 by Asp (T77D), the insertion of Ser between Gly-78 and Ser-79 (G78(S)S79), and the double mutation (T77D/G78(S)S79). The specific activities of the mutants were determined using p-nitrophenylalanine-based substrates containing a Phe or Lys residue at the P1 position. The double mutant cleaved the Lys-Phe(4-NO2) bonds, while wild-type enzyme digested other bonds. In addition, the pH dependence of hydrolysis of Lys-containing substrates by the double mutant indicates that the interactions between Asp-77 of the mutant and P1 Lys constitute to the transition state stabilization. The double mutant was also able to activate bovine trypsinogen to trypsin by the selective cleavage of the Lys6–Ile7 bond of trypsinogen. Results of this study suggest that the substrate of the active-site flap contributes to the S1 substrate specificity for basic amino acid residues in aspartic proteinases.
AorsinCitation31) (EC 3.4.21.-) (Table ) from A. oryzae RIB40 (ATCC 42149) appeared to require a basic residue at the P1 position and to prefer paired basic residues. Aorsin activated plasminogen at pH 5.4 and converted trypsinogen to trypsin at pH 5.5. The activity was inhibited strongly by antipain or leupeptin, but was not inhibited by any other standard inhibitors of peptidases. Aorsin has a catalytic triad, Glu-86, Asp-211, and Ser-354, and is not inhibited by pepstatin. Aorsin is another of the S53 family serine-carboxyl proteinases (EC 3.4.21.100, sedolisin) proteinases that are not inhibited by pepstatin.
SedolisinsCitation88) (serine-carboxyl peptidases, EC 3.4.21.100) are proteolytic enzymes that fold resembles that of subtilisin; however, they are considerably larger, with the mature catalytic domains containing approximately 375 amino acids. The defining features of these enzymes are a unique catalytic triad, Ser–Glu–Asp, as well as the presence of an aspartic acid residue in the oxyanion hole.
A new type of acid carboxypeptidasee (serine carboxypeptidase, EC 3.4.16.5)Citation32–34) of A. tubingensis (A. saitoi) (Table ) was found in 1972. The purified enzyme was found to be essentially homogeneous by such criteria as sedimentation in the ultracentrifuge and disk electrophoresis. According to the Yphantis’ procedure, the molecular weight of the enzyme was found to be 139,000. The sedimentation constant was determined to be 7.3 S. The enzyme has a pH optimum at pH 3.1 for benzyloxycarbonyl–Glu–Tyr (Z–Glu–Tyr), pH 3.5 for Z–Tyr–Leu, pH 3.2 for Z–Gly–Pro–Leu–Gly, and pH 3.5 for benzoyl–Gly–Lys (Bz–Gly–Lys). The activity was inhibited by diisopropylfluorophosphate (DFP) at pH 6.0, and was not affected by ethylenediamine tetraacetate (EDTA) at pH 5.2. These observations were considered as suggesting that the Aspergillus carboxypeptidase differs from the known carboxypeptidase A (EC 3.4.17.1) and carboxypeptidase B (EC 3.4.17.2). Takaki et al.Citation35) reported that lysine, glutamic acid and leucine were released from the anticoagulant decapeptide (SQLQEAPLEK) by A. tubingensis (A. saitoi) serine carboxypeptidase. Serine carboxypeptidase from A. tubingensis (A. saitoi) removes acidic, neutral, and basic amino acids as well as proline from the C-terminal position at pH 2–5. The bitterness of peptides from soybean protein hydrolysates was reduced by A. tubingensis (A. saitoi) serine carboxypeptidase.Citation89)
cpdS, cDNA encoding A. tubingensis (A. saitoi) carboxypeptidase, was cloned and expressed.Citation36) Analysis of the 1816-nucleotide sequence revealed a single open-reading frame coding for 523 amino acids. When A. tubingensis (A. saitoi) carboxypeptidase cDNA was expressed in yeast cells, carboxypeptidase activity was detected in the cell extract and was immunostained with a 72 kDa protein with polyclonal anti-(A. tubingensis (A. saitoi) carboxypeptidase) serum. The recombinant enzyme treated with glycopeptidase F migrated with an apparent molecular mass of 60 kDa on SDS/PAGE, which was the same as that of the de-N-glycosilated carboxypeptidase from A. tubingensis (A. saitoi). Site-directed mutagenesis of the cpdS indicated that Ser-153, Asp-357 and His-436 residues were essential for the enzyme catalysis. It can be concluded that A. tubingensis (A. saitoi) carboxypeptidase has a catalytic triad comparing Asp–His–Ser and is a member of serine carboxypeptidase family (EC 3.4.16.1).
II.ii. α-1,2-Mannosidase from A. tubingensis (A. saitoi)
An acidic α-1,2-mannosidaseCitation39) (mannosyl-oligosaccharide 1,2-α-mannosidase, EC 3.2.1.113) (Table ) has been isolated from A. tubingensis (formerly designated as A. saitoi). The molecular mass of the enzyme was 51,000 and the isoelectric point pH 4.5. The enzyme has a pH optimum of 5.0 with bakers’ yeast mannan and has no activity toward p-nitrophenyl-α-d-mannoside.
Aspergillus α-1,2-mannosidase can readily convert Manα→1–2Manα1→3Manβ1→4GlucNAcOT to Manα1→3Manβ1→4GlucNAcOT.Citation40) On the other hand, Manα1→3Manβ1→4GlcNAcOT and Manα1→6Manβ1→4XylNAcOT were completely resistant to enzyme action. The optimum pH for Manα1→2Manα1→3Manβ1→4GlucNAcOT is approximately 5.0. The Km and Vmax values for the tetrasaccharide were calculated as 0.8 mM and 10 μmol/min/mg enzyme. The oligosaccharides described above were purified from urine of mannosidosis patient, hereditary disease.
The serine carboxypeptidase (acid carboxypeptidase) from A. tubingensis (A.saitoi) contains a high amount of the carbohydrates. The new oligosaccharide moieties, Man10GlcNAc2Citation37) and Man11GlcNAc2,Citation38) were identified with α-1,2-mannosidaseCitation39) from A. tubingensis (A.saitoi) by Chiba et al. (Fig. ). These sugar chain structures have not been found in yeast and animal.
Fig. 1. Proposed structures of the new oligosaccharides (Man10GlcNAc2Citation37) [A] and Man11GlcNAc2Citation38) [B]) of Aspergillus serine carboxypeptidase.
![Fig. 1. Proposed structures of the new oligosaccharides (Man10GlcNAc2Citation37) [A] and Man11GlcNAc2Citation38) [B]) of Aspergillus serine carboxypeptidase.](/cms/asset/3f1419d7-c0e3-4c46-9089-a0d71e26d566/tbbb_a_1177445_f0001_b.gif)
Five crucial carboxyl residues of α-1,2-mannosidase from A. tubingensis (A. saitoi) were identified by site-directed mutagenesis.Citation90) Molecular properties of recombinant α-1,2-mannosidase overexpressed in A. oryzae were determined.Citation91) Approx. 320 mg of pure enzyme was obtained per liter of culture. The roles of conserved active carboxylic acids in the catalytic mechanism of A. tubingensis (A. saitoi) α-1,2-mannosidase were studied by site-directed mutagenesis and kinetic analysis.Citation41) Tatara et al.Citation41) estimated that Glu-124 is a catalytic residue based on the drastic decrease of kcat values of the E124Q and E124D mutant enzymes. Glu-124 may work as an acid catalyst, since the pH dependence of its mutants affected the basic limb. D269 N and E411Q were catalytically inactive, while D269E and E411D showed considerable activity. This indicated that the negative charges at these points are essential for the enzymatic activity and that none of these residues can be a base catalyst in the normal sense. Km values of E273D, E411D, and E474D mutants were greatly increased to 17–31-fold wild-type enzyme, and the kcat values were decreased, suggesting that each of them is a binding site of the substrate. Ca2+, essential for the mammalian and yeast enzymes, is not required for the enzymatic activity of A. tubingensis (A. saitoi) α-1,2-mannosidase. EDTA inhibits the Ca2+-free α-1,2-mannosidase as a competitive inhibitor, not as a chelator. The catalytic mechanism of α-1,2-mannosidase may deviate from that typical glycosyl hydrolase. This is the first report demonstrating that α-1,2-mannosidase requires no divalent metal cation for the activity. Tatara et al.Citation92) also reported a single free cysteine residue (Cys-443) and disulfide bond (Cys-334 and Cys-363) contribute to the thermostability of A. tubingensis (A. saitoi) α-1,2-mannosidase. These results are consistent with the crystallographic data of Penicillium α-1,2-mannosidaseCitation93) in the corresponding cysteine residues are involved in the formation of disulfide bond.
A yeast mutant capable of producing Man5GlcNAc2 human-compatible sugar chains on glycoprotein was constructed.Citation42) An expression vector for α-1,2-mannosidase with the “HDEL” endoplasmic reticulum retention/retrieval tag was designed and expressed in Saccharomyces cerevisiae. An in vitro α-1,2-mannosidase assay and western blot analysis showed that it was successfully localized in the endoplasmic reticulum. A triple mutant yeast lacking three glycosyltransferase activities was then transformed with an α-1,2-mannosidase expression vector. The oligosaccharide structures of carboxypeptidase Y as well as cell surface glycoproteins were analyzed, and the recombinant yeast was shown to produce a series of high mannose-type sugar chains, including Man5GlcNAc2. This is the first report of a recombinant S. cerevisiae able to produce Man5GlcNA2-oligosaccharides, the intermediate for hybrid-type and complex-type sugar chains.Citation42)
α-Mannosidase activities toward high-mannose oligosaccharides were examined with a detergent-solubilized microsomal preparation from A. oryzae.Citation94) In the enzymatic reaction, the pyridylaminated substrate Man9GlcNAc2-PA was trimmed to Man8GlcNAc2-PA which lacked one α-1,2-mannose residue at the non-reducing terminus of the middle branch (Man8B isomer), and this manno-oligosaccharide remained predominant through the overall reaction.Citation94) The results suggest that the activity is the same type as was previously observed with human and yeast endoplasmic reticulum (ER) α-mannosidases.
The gene manE, encoding probable class I endoplasmic reticulum α-1,2-mannosidases (ER-Man), was identified from A. oryzae due to similarity to orthologs. It removes a single mannose residue from Man9GlcNAc2, generating Man8GlcNAc2 isomer B. Disruption of manE caused drastic decreases in ER-Man activity in A. oryzae microsomes.Citation95)
II.iii. Acid activation of proyrosinase from A. oryzae
Kojic acid (5-hydroxy-2-(hydroxymethyl)-4H-pyran-4-one) (Table ) is antibiotic substance produced by A. oryzae. Isolation was performed by Saito in 1907. The chemical structure of kojic acid was reported by Yabuta in 1924.Citation96) For A. oryzae tyrosinase, competitive inhibition was observed with kojic acid.Citation97)
Melanin has many biological functions. Reactive quinone intermediates in the melanin biosynthetic pathway exhibit antibiotic properties. The melanin polymer is an important strengthening element of plant cell walls and insect cuticles. Melanin is a powerful cation chelator and may act as free radical sink.
Tyrosinase is a key enzyme involved in the biosynthetic pathway for melanin formation. The first two steps in the pathway are the oxygenation of monophenol to o-diphenol (monophenol monooxygenase, EC 1.14.18.1) and the oxidation of diphenol to o-quinones (catechol oxidase, EC 1.10.3.1), both using molecular oxygen.
A. oryzae protyrosinase (pro-TY) has a unique feature that the proenzyme is activated under condition of acidic pH (3.0).Citation43) The coding region of the protyrosinase gene, melO, from A. oryzae occupies 1671 bp of the genomic DNA.Citation44)
The protyrosinase was inactive at pH 7.0. The latent enzyme was activated at pH 3.0, and was slightly activated by sodium dodecyl sulfate (SDS). The molecular masses of the pro-TY and acid-activated tyrosinase (acid-TY) were 266 and 165 kDa, respectively, as estimated by gel filtration chromatography. The CD spectra showed that the tertiary and/or quaternary structure changed after acid activation. On the basis of these results, Tatara et al.Citation45) deduced that the inter-subunit polar interaction is disrupted at pH 3.0, and the tetrameric pro-TY dissociates to dimers. Tryptophan fluorescence spectra and binding assay of 8-anilino-1-naphthalene sulfonic acid (ANS) suggested that hydrophobic amino acid residues of the active site were exposed to solvent after acid treatment. It was likely that Cys-108 formed an intermolecular disulfide bond between the subunits of dimeric acid-TY. The dimerization of acid-TY involving the intermolecular disulfide bond is essential for the activity.
The hyper-protein production system was established in submerged culture under the tyrosinase-encoding gene (melOCitation44)) promoter.Citation46) Expression of the glucoamylase-encoding geneCitation52,53) (glaB), which is specifically expressed in solid-state culture of A. oryzae, and the tyrosinase-encoding gene (melO), was analyzed using an E. coli β-gluculonidase (GUS) reporter assay to investigate this phenomenon. Although no common regulation was found for melO and glaB expression, the former was greatly enhanced in submerged culture. Interestingly, the melO promoter was about four times stronger for GUS production than the powerful promoters, amyB, glaA, and modified agdA, previously isolated for industrial heterologous gene expression in A. oryzae. These findings indicated that the melO promoter would be suitable for hyper-production of heterologous protein in Aspergillus. The glaB-type glucoamylase selected the target protein was produced in a submerged culture of A. oryzae under the control of the melO promoter. The maximum yield was 0.8 g/L broth, and the total extracellular protein purity was 99%. Repeated batch culture, to improve productivity, gave a maximum yield of 3.3 g/L broth. The importance of this work is in the establishment of both high-level and high-purity protein overexpression system in A. oryzae by use of the melO promoter.Citation46)
Obata et al.Citation98) cloned a novel tyrosinase-encoding gene (melB) specifically expressed in solid-state culture of A. oryzae. Molecular cloning showed that the gene carried six exons interrupted by five introns and had an open-reading frame encoding 616 amino acid residues. This gene was designated as melB. The deduced amino acid sequence of the gene had weak homology with the tyrosinase MelOCitation44) in submerged culture of A. oryzae, but the sequences of the copper-binding domains were highly conserved. These results indicated that the melB gene encodes a novel tyrosinase associated with melanization in solid-state culture.
III. Conclusion
In conclusion, modern enzymology in Japanese bioindustries is advancing by quantum leaps over 100 years. All described above are widely recognized worldwide. The year of 1894 saw the true beginnings of modern microbial enzyme technology, with Takadiastase. The new enzymes, RNase T1 (EC 3.1.27.3), Aspergillus nuclease S1 (EC 3.1.30.1), γ-amylase (glucoamylase, EC 3.2.1.3), α-1,2-mannosidase (EC 3.2.1.113), serine carboxypeptidase (acid carboxypeptidase, EC 3.4.16.5), oryzin (aspergillopeptidase B, EC 3.4.21.63), aorsin (EC 3.4.21.-), aspergillopepsin I (aspartic endopeptidase, EC 3.4.23.18), deuterolysin (microbial neutral protease II, EC 3.4.24.39), and cutinase (EC 3.1.1.74) were discovered individually from Aspergullus fungi.
On the basis of the difference Fourier analysis and the model fitting study of Taka-amylase A, glutamic acid (Glu-230) and aspartic acid (Asp-297); which are located at the bottom of the cleft, were concluded to be the catalytic residues, serving as the general acid and base, respectively, by Matsuura et al.Citation51)
For the chemical mechanisms of catalysis of enzymes, protein engineering has been used to identify the residues that contribute to RNase T1-catalyzed transesterification reported by Steyaert.Citation65) Fushimi et al. concluded that deuterolysin is a member of a family of Zn2+-metalloendopeptidase with a new zinc-binding motif, aspzincin, defined by the “HEXXH + D” motif and as aspartic acid as the third zinc ligand.Citation18)
Two new evidences for enzyme-substrate formation were accumulated in this review. The first evidence was the trypsinogen activation by aspergillopepsin I from A. tubingensis (A.saitoi). Shintani et al. concluded that Asp-76 of aspergillopepsin I was the essential binding site for Lys-6 of basic substrate of trypsinogen.Citation30) Hence, electrostatic forces are stronger in the hydrophobic interior of proteins than on the solvent-exposed surface. For the raw starch affinity site of glucoamylase I, the second evidence was found by Goto et al. as O-linked oligosaccharides in threonine/serine-rich region of glucoamylase I from A. luchuensis mut. kawachii (A. awamori var. kawachii) by expression in mannosyltransferase-disruptant of yeast.Citation15)
For the gene expression, effects of water environment on glucoamylase gene from A. oryzae were appeared. The A. oryzae glucoamylase (EC 3.2.1.3)-encoding gene, glaB, is expressed specifically and strongly only during solid-state culture, koji, and the levels in submerged culture are much lower.Citation52) The glucoamylase encoding glaB gene is highly repressed in the submerged culture.
Acid activation of protyrosinase from A. oryzae to tyrosinase (EC 1.10.3.1, and EC 1.14.18.1) at pH 3.0 was discovered as a unique phenomenon. Tatara et al. resolved that the intersubunit polar interaction of tetrameric protyrosinase is disrupted at pH 3.0, and the tetrameric protyrosinase dissociates to the active dimers.Citation45) The dimerization of acid-activated tyrosinase involving the intermolecular disulfide bond with Cys-108 is essential for the activity.
The new oligosaccharide moieties, Man10GlcNAc2Citation37) and Man11GlcNAc2,Citation38) of serine carboxypeptidase from A. tubingensis (A. saitoi) were identified with α-1,2-mannosidaseCitation39) from A. tubingensis (A.saitoi). Tatara identified catalytic residues of Ca2+-independent α-1,2-mannosidase from A. tubingensis (A. saitoi) by site-directed mutagenesis.Citation41)
A yeast mutant capable of producing Man5GlcNAc2 human-compatible sugar chains on glycoprotein was constructed by Chiba et al.Citation42) This is the first report of a recombinant S. cerevisiae able to produce human compatible high mannose-type Man5GlcNA2-oligosaccharides.
For the overexpression system, recombinant α-1,2-mannosidase (EC 3.2.1.113) from A.tubingensis (A. saitoi) was constructed in A. oryzae cells.Citation91) Approx. 320 mg of pure enzyme was obtained per liter of culture. The hyper-protein production system of glucoamylase (EC 3.2.1.3) was also established.Citation46) The glaB-type glucoamylase of A. oryzae selected as the target protein was produced in a submerged culture of under the control of the melO promoter. The importance of this work is in the establishment of a high-level and high-purity protein overproduction system.
Fundamental questions still remain regarding the detailed mechanisms of enzyme activity and its relationship to enzyme structure. The two most powerful tools that have been brought to bear questions in modern times are the continued development and use of biophysical probes of protein structure, and the application of molecular biological methods to enzymology. Furthermore, the tools of molecular biology have allowed scientists to clone and express enzymes in foreign host organisms with great efficiency. Enzymes that had never before been isolated have been identified and characterized by molecular cloning. Overexpression of enzymes in prokaryotic and eukaryotic hosts has allowed the purification and characterization of enzymes that are available only in minute amounts from their natural sources. This has been a tremendous advance for protein science in general.Citation99)
Aspergillus oryzae is generally recognized as a non-pathogenic fungus. A history of saftyCitation100) and non-productivity of aflatoxin is well established for industrial strains, and A. oryzae is considered “general recognized as safe (GRAS)” by the United States Food and Drug Administration (FDA).Citation101) Molecular analysis of an inactive aflatoxin biosynthesis gene cluster in A. oryzae was reported by Tominaga et al.Citation102)
We have seen in this conclusion that the science of enzymology and enzyme technology for A. oryzae, A. sojae, and A. luchuensis, the national fungi of Japan, has a long and rich history. From phenomenological observations, enzymology has grown to a quantitative molecular science.
Disclosure statement
No potential conflict of interest was reported by the author.
Acknowledgments
We gratefully dedicate this review to our co-workers, for their kind collaborations, as well as to their technical assistants, who helped with the studies. The review is compiled in commemoration of our long enzyme research works published in original articles, and we hope that our results will contribute in helping others in attaining greater heights.
References
- Machida M, Asai K, Sano M, et al. Genome sequencing and analysis of Aspergillus oryzae. Nature. 2005;438:1157–1161.10.1038/nature04300
- Sakaguchi K, Yamada K. Morphology and classification of Aspergillus fungi. Nihon Nougeikagaku Kaishi [J. Agric. Chem. Soc. Japan]. 1944;20:65–73, 141–154. Japanese.
- Sato A, Oshima K., Noguchi H, et al. Draft genome sequencing and comparative analysis of Aspergillus sojae NBRC4239. DNA Res. 2011;18:165–176.10.1093/dnares/dsr009
- Yamada O, Takara R, Hamada R, et al. Molecular biological researches of Kuro-Koji molds, their classification and safety. J. Biosci. Bioeng. 2011;112:233–237.10.1016/j.jbiosc.2011.05.005
- Hong SB, Lee M, Kim DH, et al. Aspergillu luchuensis, an industrially important black Aspergillus in east Asia. PLOS ONE. 2013;8:e63769.
- Hong SB, Yamada O, Samson RA. Taxinomic re-evaluation of black koji mold. Appl. Microbiol. Biotechnol. 2014;98:555–561.10.1007/s00253-013-5332-9
- Takamine J. Process of making diastatic enzyme. US Patent No.525,813. Peoria, IL. patented 1894 Sept 11.
- Ishida M. Hormone hanters - Discovery of adrenaline. Kyoto: Kyoto University Press; 2012. Japanese. ISBN: 978-4-87698-587-6.
- Akabori S, Hagihara B, Ikenaka T. Purification and crystallization of Taka-amylase. Proc. Jpn. Acad. Ser. B. 1951;27:350–351.
- Akabori S, Ikenaka T, Hagihara B. Isolation of crystalline taka-amylase A from “Takadiastase Sankyo”. J. Biochem. 1954;41:577–582.
- Kitahara K, Kurushima M. Studies on the components of diastases from Aspergillus filamentous fungi. (Part 5) On the existence of a new amylase, γ-amylase. Hatuko Kogakukai-Shi [J. Ferment. Technol. Japan]. 1949;21:254–257. Japanese.
- Hayashida S, Narahara K, Kanlayakrit W, et al. Characteristics and function of raw-starch-affinity site on Aspergillus awamori var. kawachii glucoamylase molecule. Agric. Biol. Chem. 1989;53:143–149.
- Hayashida S, Nakahara K, Kuroda K, et al. Structure of the raw-starch-affinity site on the Aspergillus awamori var. kawachii. Agric. Biol. Chem. 1989;53:135–141.
- Goto M, Semimaru T, Furukawa K, et al. Analysis of the raw-starch-binding domain by mutation of a glucoamylase from Aspergillus awamori var. kawachii expressed in Saccahromyces cerevisiae. Appl. Environ. Microbiol. 1994;60:3926–3930.
- Goto M, Tsukamoto Masaki, Kwon I, et al. Functional analysis of O-linked oligosaccharides in threonine/serine-rich region of Aspergillus glucoamylase by expression in mannosyl transferase-disruptants of yeast. Eur. J. Biochem. 1999;260:596–602.10.1046/j.1432-1327.1999.00207.x
- Sato K, Egami F. Studies on ribonucleases in Takadiastase. I. J. Biochem. 1957;44:753–763.
- Ando T. A nuclease specific for heat-denatured DNA isolated from product of Aspergillus oryzae. Biochim. Biophys. Acta. 1966;114:158–168.
- Fushimi N, Ee CE, Nakajima T, et al. Aspzincin, a family of metalloendopeptidases with a new zinc-binding motif. J. Biol. Chem. 1999;274:24195–24201.10.1074/jbc.274.34.24195
- Sekine H. Neutral proteinases I and II of Aspergillus sojae. Isolation in homogeneous form. Agr. Biol. Chem. 1972;36:198–206.
- Ito K, Koyama Y. Analysis of specific proteolytic digestion of peptidoglutaminase-asparaginase of koji molds. J. Biosci. Bioeng. 2014;118:253–255.10.1016/j.jbiosc.2014.02.007
- Ito K, Hanya Y, Koyama Y. Purification and characterization of a glutaminase enzyme accounting for the majority of glutaminase activity in Aspergillus sojae under solid-state culture. Appl. Microbiol. Biotechnol. 2013;97:8581–8590.10.1007/s00253-013-4693-4
- Ito K, Koyama Y, Hanya Y. Identification of the glutaminase genes of Aspergillus sojae involved in glutamate production during soy sauce fermentation. Biosci. Biotechnol. Biochem. 2013;77:1832–1840.10.1271/bbb.130151
- Ito K, Matsushima K, Koyama Y. Gene cloning, purification and characterization of a novel peptidoglutaminase-asparaginase from Aspergillus sojae. Appl. Environ. Microbiol. 2013;78:5182–5188.
- Yoshida F. Studies on the proteolytic enzymes of black aspergilli. Part I. Investigation of strains producing proteinase yields a black Aspergillus and the crystallization of proteolytic enzymes from Aspergillus saitoi. Bull. Agric. Chem. Soc. Japan. 1956;20:252–256.
- Ichishima E, Yoshida F. Molecular weight of acid proteinase from Aspergillus saitoi. Nature. 1965;207:525–526.10.1038/207525a0
- Ichishima E, Yoshida F. Chromatographic purification and physical homogeneity of acid proteinase of Aspergillus saitoi. Biochim. Biophys. Acta. 1965;99:360–366.10.1016/S0926-6593(65)80133-3
- Majima E, Oda K, Murao S, et al. Comparative study on the specificity of several fungal aspartic and acidic proteinases towards the tetradecapeptide of a renin substrate. Agirc. Biol. Chem. 1988;52:787–793.10.1271/bbb1961.52.787
- Gabeloteau C, Desnuelle P. On the activation of beef trypsinogen by a crystallized proteinase of Aspergillus saitoi. Biochim. Biophys. Acta. 1960;42:230–237.10.1016/0006-3002(60)90786-1
- Abita JP, Delaage M, Lazdunski M, et al. The mechanism of activation of trypsinogen. The role of the four N-terminal aspartyl residues. Eur. J. Biochem. 1969;8:314–324.10.1111/ejb.1969.8.issue-3
- Shintani T, Ichishima E. Primary structure of aspergillopepsin I deduced from nucleotide sequence of the gene and aspartic acid-76 is an essential active site of the enzyme for trypsinogen activation. Biochim. Biophys. Acta. 1994;1204:257–264.10.1016/0167-4838(94)90016-7
- Lee BR, Furukawa M, Yamashita K, et al. Aorsin, a novel serine proteinase with trypsin-like specificity at acidic pH. Biochem. J. 2003;371:541–548.10.1042/bj20021691
- Ichishima E. Purification and characterization of a new type of acid carboxypeptidase from Aspergillus saitoi. Biochim. Biophys. Acta. 1972;258:274–288.10.1016/0005-2744(72)90985-0
- Ichishima E, Arai T. Specificity and mode of action of acid carboxypeptidase from Aspegillus saitoi. Biochim. Biophys. Acta. 1973;293:444–450.10.1016/0005-2744(73)90351-3
- Ichishima E. Mode of action and application of Aspergillus carboxypeptidase. Comments Agric. & Food Chemistry. 1991;2:279–298.
- Takaki A, Yoshitake S, Ishiguro M, et al. Anticoagulant peptide obtained from fibrinogenic product by plasmin. II. Sequence determination of the peptide. Proc. Jpn. Acad. 1972;48:534–538.
- Chiba Y, Midorikawa T, Ichishima E. Cloning and expression of the carboxypeptidase gene from Aspergillus saitoi and determination of the catalytic residues by site-directed mutagenesis. Biochem. J. 1995;308:405–409.10.1042/bj3080405
- Chiba Y, Yamagata Y, Nakajima T, et al. A new high-mannose type N-linked oligosaccharide from Aspergillus carboxypeptidase. Biosci. Biotechnol. Biochem. 1992;56:1371–1372.10.1271/bbb.56.1371
- Chiba Y, Yamagata Y, Iijima S, et al. The carbohydrate moiety of the acid carboxypeptidase from Aspergillus saitoi. Curr. Microbiol. 1993;27:281–288.
- Ichishima E, Arai M, Shigematsu Y, et al. Purification and acidic α-d-mannosidase from Aspergillus saitoi and specific cleavage of 1,2-α-d-mannosidic linkage in yeast manan. Biochim. Biophys. Acta. 1981;658:45–53.
- Yamashita K, Ichishima E, Arai M, et al. An α-mannosidase purified from Aspergillus saitoi is specific for α1,2 linkages. Biochem. Biophys. Res. Commun. 1980;96:1335–1342.10.1016/0006-291X(80)90097-2
- Tatara Y, Lee BR, Yoshida T, et al. Identification of catalytic residues of Ca2+-independent 1,2-α-mannosidse from Aspergillus saitoi by site-directed mutagenesis. J. Biol. Chem. 2005;278:25289–25294.
- Chiba Y, Suzuki M, Yoshida S, et al. Production of human compatible high mannose-type (Man5GlcNAc2) sugar chains in Saccharomyces cerevisiae. J. Biol. Chem. 1998;273:26298–26304.10.1074/jbc.273.41.26298
- Ichishima E, Maeba H, Amikura T, et al. Multiple forms of protyrosinase from Aspergillus oryzae and their mode of activation at pH 3.0. Biochim. Biophys. Acta. 1984;786:25–31.10.1016/0167-4838(84)90149-3
- Fujita Y, Uraga Y, Ichisima E. Molecular cloning and nucleotide sequence of the protyrosinase gene, melO, from Aspergillus oryzae and expression of the gene in yeast. Biochim. Biophys. Acta. 1995;1261:151–154.10.1016/0167-4781(95)00011-5
- Tatara Y, Namba T, Yamagata Y, et al. Acid activation of protyrosinase from Aspergillus oryzae: homo-tetrameric protyrosinase is converted to active dimers with an essential intersubunit disulfide bond at acidic pH. Pigment Cell Melanoma Res. 2007;21:89–96.10.1111/j.1755-148X.2007.00422.x
- Ishida H, Matsumura K, Hata Y, et al. Establishment of a hyper-protein production system in submerged Aspergillus oryzae culture under tyrosinase-encoding gene (melO) promoter control. Appl. Microbiol. Biotechnol. 2001;57:131–137.
- Brew. Soc. Jpn. The national fungi of Japan. http://www.jozo.or.jp/koujikinnituite2.pdf 2006
- Toda H, Kondo K, Narita K. The complete amino acid sequence of Taka-amylaseA. Proc. Jpn. Acad. Ser. B. 1982;58:208–212.10.2183/pjab.58.208
- Tada S, Iimura Y, Gomi K, et al. Cloning and nucleotide sequence of the genomic Taka-amylase A gene of Aspergillus oryzae. Agric. Biol. Chem. 1989;53:593–599.
- Matsuura Y, Kusunoki M, Harada W, et al. Molecular structure of Taka-amylase A. I. Backbone chain folding at 3 Ǻ. J. Biochem. 1980;87:1555–1558.
- Matsuura Y, Kusunoki M, Harada W, et al. Structure and possible catalytic residue of Taka-amylase A. J. Biochem. 1984;95:697–702.
- Hata Y, Ishida H, Ichikawa E, et al. Nucleotide sequence of an alternative glucoamylase-encoding gene (glaB) expressed in solid-state culture of Aspergillus oryzae. Gene. 1987;207:127–134.
- Hata Y, Kitamoto K, Gomi K, et al. The glucoamylase cDNA from Aspergillus oryzae: its cloning, nucleotide sequence, and expression in Saccharomyces cerevisiae. Agric. Biol. Chem. 1991;55;941–949.
- Hata Y, Ishida H, Kojima Y, et al. Comparison of two glucoamylases produced by Aspergillus oryzae in solid-state culture (koji) and in submerged culture. J. Ferment. Bioeng. 1997;84:532–537.10.1016/S0922-338X(97)81907-1
- Hisada H, Sano M, Ishida H, et al. Identification of regulatory elements in the glucoamylase-encoding gene (glaB) promoter from Aspergillus oryzae. Appl. Microbiol. Biotechnol. 2013;97:4951–4956.10.1007/s00253-012-4622-y
- Ueda S. Studies on the amylolytic system of the black-koji molds. Part II. Raw starch digestability of the saccharogenic amylase fraction and its interaction with the dextrogenic amylase fraction. Bull. Agr. Chem. Soc. Japan. 1956;21:284–287.
- Yamanaka K. Studies on the pyruvate and carbohydrate metabolisms by lactic acid bacteria. Part IX. Formation of ketopentose by lactic acid bacteria. Bull. Agr. Chem. Soc. Japan. 1958;22:299–308.
- Yamanaka K. Fructose synthesis from glucose ‐ A new method of isomerizing sugar. Kagaku To Saeibutu. 2011;48:643–651. Japanese.
- Hirotsune M. New function of ethyl α-d-glucoside. Nippon Jyōzōkyōkai Shi [J. Brewing Soc. Japan]. 2004;99:836–841. Japanese.
- Chiba S. Molecular mechanism in α-glucosidase and glucoamylase. Biosci. Biotechnol. Biochem. 1997;61:1233–1239.10.1271/bbb.61.1233
- Takahashi K. The amino acid sequence of ribonuclease T1. J. Biol. Chem. 1965;240:4117–4119.
- Oobatake M, Takahashi K, Ooi T. Conformational stability of ribonuclease T1. II. Salt-induced renaturation. J. Biochem. 1979;86:65–70.
- Anfinsen CB, Scheraga HA. Experimental aspects of protein folding. Adv. Protein Chem. 1975;29:205–300.10.1016/S0065-3233(08)60413-1
- Nishikawa S, Morioka H, Kim HJ, et al. Two histidine residues are essential for ribonuclease T1. Biochemistry. 1987;26:8620–8624.10.1021/bi00400a019
- Steyaert J. A decade of protein engineering on ribonuclease T1-atomic dissection of the enzyme-substrate interactions. Eur. J. Biochem. 1997;247:1–11.10.1111/ejb.1997.247.issue-1
- Sutton WD. A crude nuclease preparation suitable for use in DNA ressociation experiments. Biochim. Biophys. Acta. 1971;240:522–531.10.1016/0005-2787(71)90709-X
- Vogt VM. Purification and further properties of single-strand-specific nuclease from Aspergillus oryzae. Eur J Biochem. 1973;33:192–200.10.1111/ejb.1973.33.issue-1
- Volbed A, Lahm A, Sakiyama F, et al. Crystal structure of Penicillium citrinum P1 nuclease at 2.8 A resolution. EMBO J. 1991;10:1607–1618.
- Beck RW. 1966. P. Molecular biology: S1 Nuclease. BISEIBUTUGAKU NO REKISHI [A Chronology of Microbiology in Historical Context]. Tokyo: ASAKURA SHOTEN (ASM Press,Washington, DC 2004). 2004;II:144. Japanese.
- Doi Y, Lee BR, Ikeguchi M, et al. Substrate specificities of deuterolysin from Aspergillus oryzae and electron paramagnetic resonance measurement of cobalt-substituted deuterolysin. Biosci. Biotechnol. Biochem. 2003;76:264–270.
- Yamaguchi M, Hanzawa S, Hirano K, et al. Specificity and molecular properties of penicillolysin, a metalloproteinase from Penicillium citrinum. Phytochemistry. 1993;33:1317–1321.10.1016/0031-9422(93)85082-3
- Matsumoto K, Yamaguchi M, Ichishima E. Molecular cloning and nucleotide sequence of the complementary DNA for penicillolysin gene, plnC, an 18 kDa metalloendopeptidase gene from Penicillium citrinum. Biochim. Biophys. Acta. 1994;1218:469–472.10.1016/0167-4781(94)90209-7
- Doi Y, Akiyama H, Yamada Y, et al. Thermal stabilization of penicillolysin, a thermolabile 19 kDa Zn2+-protease, obtained by site-directed mutagenesis. Protein Eng. Des. Select. 2004;17:261–266.
- Subramanian AR, Kalnitsky G. The major alkaline proteinase of Aspergillus oryzae, aspergillopeptidase B. I. Isolation in homogeneous form. Biochemistry. 1964;3:1861–1867.
- Subramanian AR, Kalnitsky G. The major alkaline proteinase of Aspergillus oryzae, aspergillopeptidase B. II. Partial specific volume, molecular weight and amino acid composition. Biochemistry. 1964;3:1868–1874.10.1021/bi00900a013
- Hayashi K, Terada M. Some characteristics of hydrolysis of synthetic substrates and proteins by the alkaline proteases from Aspergillus sojae. Agric. Biol. Chem. 1972;36:1755–1765.10.1080/00021369.1972.10860476
- Murakami K, Ishida Y, Masaki A, et al. Isolation and characterization of the alkaline protease gene of Aspergillus oryzae. Agric. Biol. Chem. 1991;55:2807–2811.
- Udo S. Studies on the Umami taste of soy sauce. (Part 4) Correlation of the chemical components and the Umami taste of soy sauce. Nihon Nougeikagaku Kaishi [J. Agric. Chem. Soc. Japan]. 1932;8:675–684. Japanese.
- Sunde M, Kwan AH, Templeton MD, et al. Structural analysis of hydrophobins. Micron. 2008;39:773–784.10.1016/j.micron.2007.08.003
- Wessels J, De Vries O, Asgerirsdottir SA, et al. Hydrophobin genes involved in formation of aerial hyphae and fruit bodies in Schizophyllum. Plant Cell. 1991;3:793–799.10.1105/tpc.3.8.793
- Maeda H, Yamagata Y, Abe K, et al. Purification and characterization of a biodegradable plastic-degrading enzyme from Aspergillus oryzae. Appl. Microbiol. Biotechnol. 2005;67:778–788.10.1007/s00253-004-1853-6
- Tanaka T, Tanabe H, Uehara K, et al. Involvement of hydrophobic amino acid residues in C7–C8 loop of Aspergillus oryzae hydrophobin RolA in hydrophobic interaction between RolA and a polyester. Biosci. Biotechnol. Biochem. 2014;78:1693–1699.10.1080/09168451.2014.932684
- Takahashi T, Tanaka T, Tsushima Y, et al. Ionic interaction of positive amino acid residues of fungal hydrophobin RolA with acidic amino acid residues of cutinase CutL1. Mol. Microbiol. 2015;96:14–27.
- Sakaguchi K, Iizuka H, Yamazaki S. A study on black Aspergilli. Nihon Nougeikagaku Kaishi [J. Agric. Chem. Soc. Japan]. 1950;24:138–142. Japanese.
- Ichishima E. Unique enzymes of Aspergillus fungi used in Japnese bioindustries. New York, NY: Nova Science Publishers; 2012.
- Shintani T, Kobayashi M, Ichishima E. Characterization of the S1 subsite specificity of aspergillopepsin I by site-directed mutagenesis. J. Biochem. 1996;120:974–991.
- Shintani T, Nomura K, Ichishima E. Engineering of porcine. Alterration of S1 substrate specificity of pepsin to those of fungal aspartic proteinases by site-directed mutagenesis. J. Biol. Chem. 1997;272:18855–18861.10.1074/jbc.272.30.18855
- Wlodawer A, Li M, Dauter Z, et al. Carboxyl proteinase from pseudomonas defines a novel family of subtilisin-like enzymes. Nat. Struct. Biol. 2001;8:442–446.10.1038/87610
- Arai S, Yamashita M, Kato H, et al. Applying proteolytic enzymes on soybean. Part V. A nondialyzable bitter peptide in peptic hydrolysate of soybean protein and its bitterness in relations to the chemical structure. Agric. Biol. Chem. 1970;34:729–738.
- Fujita A, Yoshida T, Ichishima E. Five crucial carboyl residues of 1,2-α-mannosidase from Aspergillus saitoi (A. phoenisis), a food microorganism, are identified by site-directed mutagenesis. Biochem. Biophys. Res. Commun. 1997;238:779–783.10.1006/bbrc.1997.7389
- Ichishima E, Taya N, Ikeguchi M, et al. Molecular and enzymatic properties of recombinant 1,2-α-mannosidase from Aspergillus saitoi overexpressed in Aspergillus oryzae cells. Biochem. J. 1999;339:589–597.10.1042/bj3390589
- Tatara Y, Yoshida T, Ichishima E. A single free cysteine residue and disulfide bond contribute to the thermostability of Aspergillus saitoi 1,2-α-mannosidase. Biosci. Biotechnol. Biochem. 2005;69:2101–2108.10.1271/bbb.69.2101
- Lobsanov YD, Vallée F, Imberty A, et al. Structure of Penicillium citrinum α1,2-mannosidase reveals the basis for differences in specificity of the endoplasmic reticulum and Golgi Class I enzymes. J. Biol. Chem. 2002;277:5620–5630.
- Yoshida T, Kato Y, Asada Y, et al. Filamentous fungus Aspergillus oryzae has two types of α-1,2-mannosidases, one of which is a microsomal enzyme that removes a single mannose residue from Man9GlcNAc2. Glycoconj. J. 2000;17:745–748.
- Akao T, Yahara A, Sakamoto K, et al. Lack of endoplasmic reticulum 1,2-α-mannosidase activity that trims N-glycan Man9GlcNAc2 to Man8GlcNAc2 isomer B in a manE gene disruptant of Aspergillus oryzae. J. Biosci. Bioeng. 2012;113:438–441.10.1016/j.jbiosc.2011.11.015
- Yabuta T. The constitution of kojic acid, a γ-pyrone derivative formed by Aspergillus oryzae from carbohydrates. J. Chem. Soc. 1924;125:575.10.1039/CT9242500575
- Tanaka T, Takeuchi M, Ichishima E. Inhibition study of tyrosinase from Aspergillus oryzae. Agric. Biol. Chem. 1989;53:557–558.
- Obata H, Ishida H, Hata Y, et al. Cloning of a novel tyrosinase-encoding gene (melB) from Aspergillus oryzae and its overexpression in solid-state culture (Rice Koji). J. Biosci. Bioeng. 2004;97:400–405.10.1016/S1389-1723(04)70226-1
- Copeland RA. ENZYMES – a practical introduction to structure, mechanism, and data analysis. 2nd ed. New York, NY: John Wiley & Sons; 2000.
- Barkesgaard P, Heldt-Hansen HP, Diderichsen B. On the safety of Aspergillus oryzae: a review. Appl. Microbiol. Biotechnol. 1992;36:569–572.
- Taylor MJ, Richardson T. Application of microbial enzymes in food systems and in biotechnology. Adv. Appl. Microbiol. 1979;25:7–35.10.1016/S0065-2164(08)70144-8
- Tominaga M, Lee YH, Hayashi R, et al. Molecular analysis of an inactive aflatoxin biosynthesis gene claster in Aspergillus oryzae RIB strains. Appl. Environ. Microbiol. 2006;72:484–490.10.1128/AEM.72.1.484-490.2006