Abstract
NAD + -dependent glyceraldehyde dehydrogenases usually had lower activity in the nonphosphorylated Entner–Doudoroff (nED) pathway. In the present study, a new NAD + -dependent glyceraldehyde dehydrogenase was engineered from l-lactaldehyde dehydrogenase of E. coli (EC: 1.2.1.22). Through comparison of the sequence alignment and the active center model, we found that a residue N286 of l-lactaldehyde dehydrogenase contributed an important structure role to substrate identification. By free energy calculation, three mutations (N286E, N286H, N286T) were chosen to investigate the change of substrate specificity of the enzyme. All mutants were able to oxidate glyceraldehyde. Especially, N286T showed the highest activity of 1.1U/mg, which was 5-fold higher than the reported NAD + -dependent glyceraldehyde dehydrogenases, and 70% activity was retained at 55 °C after an hour. Compared to l-lactaldehyde, N286T had a one-third lower Km value to glyceraldehyde.
Graphical abstract
Molecular structure of l-lactaldehyde dehydrogenase from E. coli.
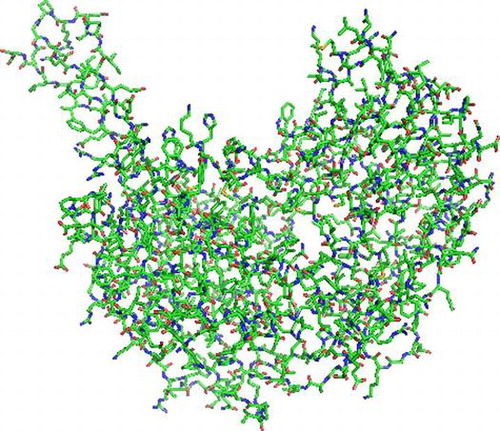
The aldehyde dehydrogenases (ALDH) are mainly NAD+-dependent or NADP+-dependent enzymes, which oxidize the aldehydes to their corresponding carboxylic acids. These enzymes widely exist in living systems from prokaryotes to eukaryotes.Citation1–7) All ALDHs share the same catalytic step that hydrosulfonyl from cysteine nucleophilic attacks the carboxide in aldehyde, afterwards forming appropriate through hydrolyzation; meanwhile, the hydrogen ions transfer to NAD(P)+.Citation8–10)
In Escherichia coli (E. coli), lactaldehyde dehydrogenase (ALDA) is an important intermediate enzyme in the metabolic network of l-fructose and l-rhamnose. In the metabolism process, l-focese and l-rhamnose finally are cleaved to dihydroxyacetone phosphate and lactaldehyde. After that, the lactaldehyde is oxidized to pyruvate, which enters into the Krebs cycle. The whole process takes two steps from lactaldehyde dehydrogenate to lactate dehydrogenate.Citation11–14)
Recently, with better understanding of cell-free system, an important aldehyde dehydrogenase—glyceraldehyde dehydrogenase (AIDH) has drew more attentions. The structure of glyceraldehyde is similar to lactaldehyde, except an extra hydroxyl.
In biocatalytic production, NAD+ represents a cheaper and more stable alternative to NADP+.Citation15) However, it was reported that all available AIDHs are found from archaebacteria, such as Picrophilus torridus and Thermoplasma acidophilum. Furthermore, these enzymes normally require extreme conditions to catalyze the oxidation in d-glyceraldehyde. More importantly, it was found that only one AIDH from Thermoplasma acidophilum uses NAD+ as a cofactor, and the activity of the recombinant of this enzyme in E. coli BL21 (DE3) is nearly undetectable.Citation16) Based on these findings, it is important to develop a high-activity NAD+-dependent AIDH
In this study, the active site residues of ALDA were changed by site-specific mutagenesis. Mutation residues were defined by simulating mutants and calculating the free energy based on molecular mechanics/Poisson–Boltzmann (PB) solvent-accessible surface area (MM/PBSA) methodology.Citation17) A modified NAD+-dependent AIDH presented a highest activity at the time of this report, which was designed from ALDA in E. coli.
Materials and methods
Materials and strains
Escherichia coli MG1655, Escherichia coli BL21 (DE3), and expression vector pET-22b were from the China General Microbiological Culture Collection Centre. The plasmids, DNA extraction system kit and protein molecular mass markers were supplied by Takara (Japan). All chemical reagents were purchased from Sigma-Aldrich (Nanjing, China) and Wanqing (Nanjing, China) and used without further purification. Primer oligonucleotides and DNA sequencing were produced by Jinsirui (China).
DNA manipulation
DNA was purified, digested, and ligated using standard protocols.Citation18) The gene aldA was then cloned into the plasmid pET-22b. Site-specific mutagenesis was completed by the Quik Change Site-Directed Mutagenesis Kit from Stratagene (USA). The DNA of presumed mutants was sequenced at Jinsirui to confirm the presence of the desired mutations and absence of secondary mutations.
Expression and purification of wt-ALDA and mutants
Escherichia coli BL21(DE3) cells carrying recombined plasmids were cultivated in 5 mL LB medium containing ampicillin (100 μg/mL) overnight at 37 °C. Then, 2 mL culture was inoculated into 100 mL LB medium containing ampicillin (100 μg/mL) and grown at 37 °C. Afterwards, for the enzyme expression, the 100 mL culture was inoculated into 3 L TB medium (1.5% peptone, 2.5% yeast extract, 1% NaCl, 0.2% glucose, and 0.3% lactose; pH 7) containing ampicillin (100 μg/mL) overnight at 30 °C. After medium removal by centrifugation at 6000 × g for 15 min at 4 °C, the bacterial pellet was washed with phosphate buffer (100 mM, pH 7) and resuspended in the same buffer.Citation19)
The cells were lysed by a French press operating at 800 Mpsi, and the supernatant was collected by centrifugation at 8000 × g for 15 min at 4 °C. Protein purification was performed using AKTA prime plus with software prime view. The ALDA and its mutants were purified using anion-exchange chromatography with a DEAE-Sepharose column. The column was pre-equilibrated with 100 mL equilibrium buffer (25 mM phosphate buffer, pH 7). The sample (50 mL) was loaded with a flow rate of 1.0 mL/min. After washing the unbound protein with elution buffer (25 mM phosphate buffer, 1 M NaCl, pH 7), the bound ALDA or its mutants were eluted with a linear gradient 40–60% elution buffer. The collected proteins were stored at −20 °C. Protein concentration was measured by the Bradford method (Bradford 1976).
Substrate specificity and activity assay
l-Lactaldehyde dehydrogenase activity was measured spectrophotometrically (E340) by the rate of NADH formation at 37 °C for the substrates 5 mM l-lactaldehyde, 5 mM d-glyceraldehyde, 5 mM methylglyoxal, 5 mM acetaldehyde, 5 mM l-lactate, or 5 mM benzaldehyde.Citation20) The reaction (0.2 mL) was started by adding 10 μL of an appropriate amount of enzyme at 37 °C. One unit of activity is defined as reduction of 1 μmol of cofactor per minute.Citation21)
Effects of pH and temperature on enzyme activity
The pH dependences of wt-ALDA and its mutants were determined by activity assays in 100 mM sodium phosphate buffer pH 4–10. The optimal temperature was investigated in the range of 25–60 °C. To analyze the thermal stability, the enzyme was incubated for 1 h at various temperature in the range of 25–60 °C. Residual activity was measured spectrophometrically at 37 °C and pH 7.0 using l-lactaldehyde as the substrate.
Kinetic parameters of wt-ALDA and mutants
Km and kcat were obtained by measuring the initial velocities of the enzymatic reactions and curve fitting according to the Michaelis–Menten equation. The enzyme activity assay was performed by varying the substrate concentrations of l-lactaldehyde (0.1–5 mM) or d-glyceraldehyde (0.1–5 mM).
Molecular modeling of wt-ALDA and mutants
The structure of ALDA from E. coli with accession No. 2IMP was retrieved from the RCSB Protein Data Bank (http://www.rcsb.org/). The selected residues were mutated to all of the other amino acids on the 3D-structure using the software Pymol. Calculation of the Gibbs free energy (ΔG) between each simulated mutant enzyme and substrate was performed by the software AMBER 12.Citation22,23)
Results
The sequence and structure alignment showed that Asn286 played an important role in affecting the accommodation of the substrate in the activity pocket (Fig. ). This residue was mutated to all of the others in the 3D model by Pymol and the Gibbs free energy of protein–substrate interaction calculated by Amber 12. The results showed that the mutants N286T, N286E, and N286H produced obvious reductions in the Gibbs free energy (Table ).
Fig. 1. Sequence and structure alignment of ALDA and BADH, ALDA shows in green, BADH shows in yellow.
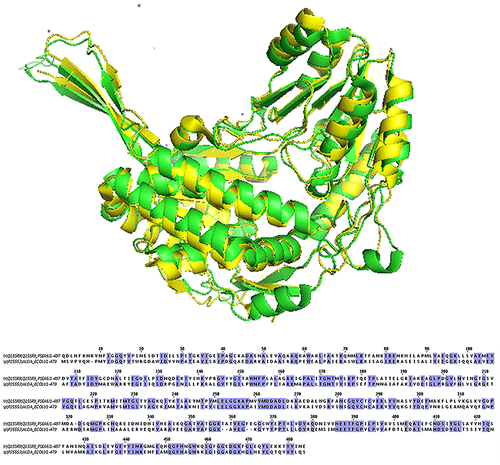
Table 1. Gibbs free energy of protein-substrate interaction calculated by Amber 12, with d-glyceraldehyde as substrate.
Purification of wt-ALDA and mutants
The wt-ALDA and its mutants were expressed in Escherichia coli BL21 at 30 °C. The soluble proteins were purified to near homogeneity in one chromatographic step using a DEAE-Sepharose column. The purified proteins revealed a single band with the expected molecular weight of 52.2 kDa by sodium dodecyl sulfate polyacrylamide gel electrophoresis (Fig. ).
Expanded substrate profile
Aldehydes were selected for catalysis by the purified enzymes. The concentrations of all substrates were 5 mM.
Under the conditions pH = 7, 37 °C, wt-ALDA showed strict substrate specificity and barely catalyzed l-lactaldehyde, but the mutants all showed expanded substrate specificity. The substrate profiles of the mutants of Asn286 were greatly changed, allowing them to that they could catalyze acetaldehyde, methylglyoxal, and d-glyceraldehyde. The mutant N286T showed higher specific activity toward l-lactaldehyde, with an enhancement of 300% compared to the wild-type enzyme. In particular, its activity toward d-glyceraldehyde reached 1.1 U/mg (Table ).
Table 2. Substrate specificity of ALDA and mutants.
pH and temperature stability of wt-ALDA and mutants
Both wt-ALDA and mutant N286T exhibited their maximum activity at pH 10, whereas the mutant N286H showed its maximum activity at pH 9. The mutant N286E exhibited its lowest maximum activity at pH 8 (Fig. ).
Fig. 2. Sodium dodecyl sulfate polyacrylamide gel electrophoresis analysis of ALDA and mutants. M protein markers with indicated molecular mass (kDa) indicated alongside. ALDA wild-type ALDA, H449R mutants H449R, L158Y mutantsL158Y, N286H mutants N286H, N286E mutants N286E, N286T mutants N286T.
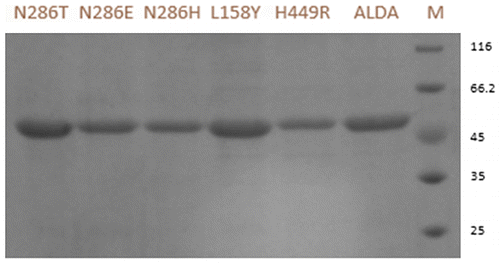
Wild-type ALDA exhibited its maximum activity at 40 °C. The mutants N286T and N286E showed little change, exhibiting their maximum activity at 45 °C. However, the mutant N286H exhibited its maximum activity at 15 °C higher than the wild type. Wt-ALDA was stable at 40 °C after incubation for 1 h, but its stability decreased rapidly above 40 °C. The mutants N286E and N286H showed the same stability, decreasing rapidly above 40 °C and exhibiting 20% and 40% of their maximum activity at 45 °C, respectively. In contrast, N286T was stable above 50 °C and retained 60% of its maximum activity at 55 °C after incubation for 1 h (Figs. and ).
Kinetic parameters of wt-ALDA and mutants
The Michaelis–Menten kinetic constants (Km, kcat, and kcat/Km) were determined for l-lactaldehyde and d-glyceraldehyde (Table ). With regard to l-lactaldehyde, wt-ALDA revealed the lowest catalytic efficiency (kcat/Km), whereas all mutants showed at least 4-fold higher values. The mutant N286T showed the highest conversion rate (kcat), enhanced 2.5-fold compared to the wild type. All of the mutants had catalytic activity toward d-glyceraldehyde. Among them, the mutant N286T showed the highest catalytic efficiency, 5-fold higher than the others. In particular, the Km value showed that the active site of the enzyme could bind more easily with d-glyceraldehyde than l-lactaldehyde. Therefore, the mutant N286T showed completely altered substrate-binding characteristics.
Table 3. Kinetic parameters of ALDA and mutants.
Discussion
The structure of l-lactaldehyde dehydrogenase from E. coli is very similar to betaine–aldehyde dehydrogenase (BADH). The RMSD of the two enzymes’ superimposition was calculated to be 1.48 Å by the software SPDBV.Citation18) In the active site, the two enzymes were nearly identical except for Asn286 in ALDA and Thr286 in BADH (Fig. ). BADH is also an NAD+-dependent aldehyde dehydrogenase, but the enzymes’ substrates are entirely different. Therefore, residue Asn286 plays a key role in substrate specificity. Based on this result, the amino acid residue Asn286 was selected for mutation. In addition, the AIDH from Thermoplasma acidophilum also shows great homology to BADH,Citation15) and so the mutants in N286 can improve the specific active of d-glyceraldehyde.
Fig. 5. Effects of temperature on the stability of ALDA and mutants, with l-lactaldehyde as substrate.
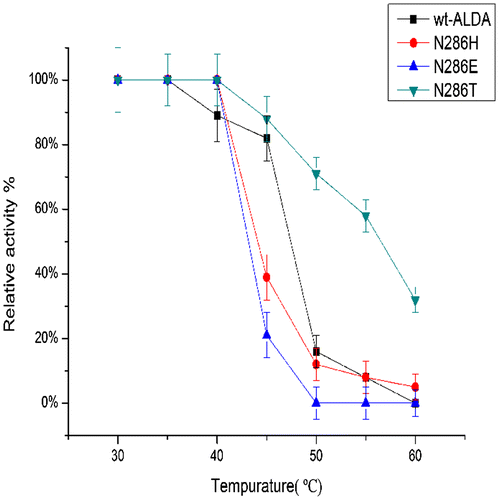
The results showed that Asn286 was closely involved in substrate identification. Therefore, three mutants (N286T, N286E, N286H) were selected based on free-energy calculations by molecular dynamics (MD) simulations.Citation17) Mutating residue Asn286 to Thr expanded the space of the active funnel and improved the hydrophilicity, which enhanced the ability of the substrate binding pocket to accommodate polyhydroxy aldehydes, allowing more types of aldehydes to be bound in the active site. Mutating Asn286 to His or Glu altered the charge of the active site centre, accelerating the electron transfer. Meanwhile, the polar side chains of these residues would combine effectively with a polyhydroxy aldehyde (Fig. ). It was found that all three mutations could catalyze d-glyceraldehyde. Interestingly, based on Km, the binding force to d-glyceraldehyde was much stronger than to the natural substrate l-lactaldehyde.Citation24, 25)
Fig. 6. Superimposition of the secondary structural elements of ALDA (shown in blue) and BADH (shown in green), with residue Asn286 in ALDA, Thr290 in BADH.
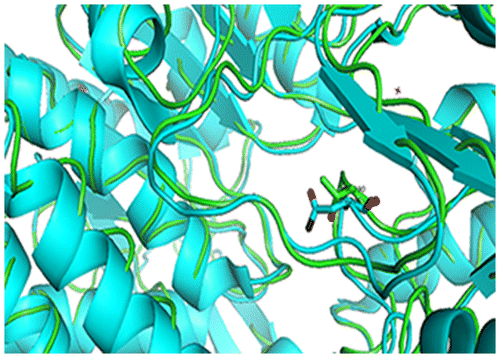
Fig. 7. Superimposition of mutants and wt-ALDA. Molecules are color-coded corresponding to the coordinates of the mutational residues. The dotted yellow lines are the distances between two atoms. White is N286, yellow is H286, green is T286, blue is E286, and pink is C285.
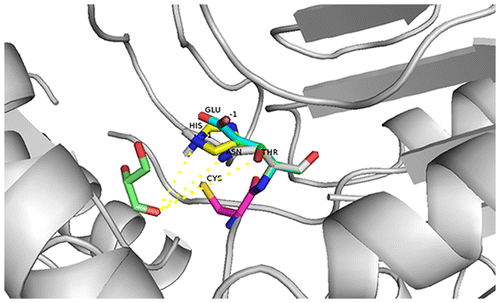
Among the mutants, only N286T exhibited a more stable enzymatic structure. All of the other mutants showed no catalytic ability above 40 °C. The mutation to residue T improved the hydrophilicity, and the hydroxyl on the side chain was able to form hydrogen bonds with nearby residues, such as E443 and H449. Therefore, the additional hydrogen bonds let the structure in active site more stable. As this reason, the mutation N286T improved the stability.
This work elucidates that the mutant N286T is a novel d-glyceraldehyde dehydrogenase.
Author contributions
Ming Yan and Lin Xu conceived and designed the study. Xing Wu performed the experiments and analysed the data. Xing Wu and Ming Yan wrote the manuscript. Ming Yan and Lin Xu reviewed and commented on the manuscript. Ming Yan and Lin Xu. supported in the finance. All authors have read and approved of the manuscript.
Funding
This work was supported by the National Basic Research Program of China [grant number 2011CBA00804].
Disclosure statement
No potential conflict of interest was reported by the authors.
References
- Monder C. Alpha-keto aldehyde dehydrogenase, an enzyme that catalyzes the enzymic oxidation of methylglyoxal to pyruvate. J. Biol. Chem. 1967;242:4603–4609.
- Boronat A, Aguilar J. Rhamnose-induced propanediol oxidoreductase in Escherichia-coli – purification, properties, and comparison with the fucose-induced enzyme. J. Bacteriol. 1979;140:320–326.
- Ray M, Ray S. On the interaction of nucleotides and glycolytic-intermediates with nad-linked alpha-ketoaldehyde dehydrogenase. J. Biol. Chem. 1982;257:571–574.
- Hempel J, Nicholas H, Lindahl R. Aldehyde dehydrogenases: widespread structural and functional diversity within a shared framework. Protein Sci. 1993;2:1890–1900.
- Liu ZJ, Sun YJ, Rose J, et al. The first structure of an aldehyde dehydrogenase reveals novel interactions between NAD and the Rossmann fold. Nat. Struct. Biol. 1997;4:317–326.
- Perozich J, Nicholas H, Lindahl R, et al. The big book of aldehyde dehydrogenase sequences – an overview of the extended family. Adv. Exp. Med. Biol. 1999;463:1–7.
- Sophos NA, Vasiliou V. Aldehyde dehydrogenase gene superfamily: the 2002 update. Chem. Biol. Interact. 2003;143–144:5–22.
- Rodríguez-Zavala Jose S. Enhancement of coenzyme binding by a single point mutation at the coenzyme binding domain of E-coli lactaldehyde dehydrogenase. Protein Sci. 2008;17:563–570.
- Baker P, Carere J, Seah SYK. Substrate specificity, substrate channeling, and allostery in BphJ: an acylating aldehyde dehydrogenase associated with the pyruvate aldolase BphI. Biochemistry-US. 2012;51:4558–4567.
- Zheng HB, Wang X, Yomano LP, et al. Improving Escherichia coli FucO for furfural tolerance by saturation mutagenesis of individual amino acid positions. Appl. Environ. Microbiol. 2013;79:3202–3208.
- Baldoma L, Aguilar J. Involvement of lactaldehyde dehydrogenase in several metabolic pathways of Escherichia-coli-k12. J. Biol. Chem. 1987;262:13991–13996.
- Altaras NE, Cameron DC. Metabolic engineering of a 1, 2-propanediol pathway in Escherichia coli. Appl. Environ. Microbiol. 1999;65:1180–1185.
- David L, Lucy AH. Methylglyoxal metabolism and diabetic complications: roles of aldose reductase, glyoxalase-I, betaine aldehyde dehydrogenase and 2-oxoaldehyde dehydrogenase. Chem. Biol. Interact. 2003;143–144:341–351.
- Di Costanzo L, Gomez GA, Christianson DW. Crystal structure of lactaldehyde dehydrogenase from Escherichia coli and inferences regarding substrate and cofactor specificity. J. Mol. Biol. 2007;366:481–493.
- Steffler Fabian, Sieber Volker. Refolding of a thermostable glyceraldehyde dehydrogenase for application in synthetic cascade biomanufacturing. PLoS ONE. 2013;8:e70592.
- Chenault HK, Whitesides GM. Regeneration of nicotinamide cofactors for use in organic synthesis. Appl. Biochem. Biotechnol. 1987;14:147–197.
- Cornell WD, Cieplak P, Bayly CI, et al. A second generation force field for the simulation of proteins, nucleic acids, and organic molecules. J. Am. Chem. Soc. 1996;118:2309–2309.
- Hidalgo E, Chen YM, Lin EC, et al. Molecular cloning and DNA sequencing of the Escherichia coli K-12 ald gene encoding aldehyde dehydrogenase. J. bacteriol. 1991;173:6118–6123.
- Sridhara S, Wu TT. Purification and properties of lactaldehyde dehydrogenase from Escherichia coli. J. Biol. Chem. 1969;244:5233–5238.
- Li G, Ren J, Iwaki H, et al. Substrate profiling of cyclohexylamine oxidase and its mutants reveals new biocatalytic potential in deracemization of racemic amines. Appl. Microbiol. Biotechnol. 2014;98:1681–1689.
- Zhu Y, Lin EC. Loss of aldehyde dehydrogenase in an Escherichia coli mutant selected for growth on the rare sugar l-galactose. J. Bacteriol. 1987;169:785–789. Cornell Wendy D, et al. A second generation force field for the simulation of proteins, nucleic acids, and organic molecules. J. Am. Chem. Soc. 1995;117:5179–5197.
- Salomon-Ferrer R, Case DA, Walker RC. An overview of the Amber biomolecular simulation package. Wiley Interdisciplinary Rev: Comput. Mol. Sci. 2013;3:198–210.
- Yen C-C, Malmis CC, Lee GC, et al. Site-specific saturation mutagenesis on residues 132 and 450 of Candida rugosa LIP2 enhances catalytic efficiency and alters substrate specificity in various chain lengths of triglycerides and esters. J. Agric. Food Chem. 2010;58:10899–10905.
- Guex, N, Peitsch MC. SWISS-MODEL and the Swiss-Pdb viewer: an environment for comparative protein modeling. Electrophoresis. 1997;18:2714–2723.
- Reher, Matthias, Schönheit Peter. Glyceraldehyde dehydrogenases from the thermoacidophilic euryarchaeota Picrophilus torridus and Thermoplasma acidophilum, key enzymes of the non-phosphorylative Entner–Doudoroff pathway, constitute a novel enzyme family within the aldehyde dehydrogenase superfamily. FEBS letters 580: 1198–1204.