Abstract
Recently, a “human gut microbial gene catalogue,” which ranks the dominance of microbe genus/species in human fecal samples, was published. Most of the bacteria ranked in the catalog are currently publicly available; however, the growth media recommended by the distributors vary among species, hampering physiological comparisons among the bacteria. To address this problem, we evaluated Gifu anaerobic medium (GAM) as a standard medium. Forty-four publicly available species of the top 56 species listed in the “human gut microbial gene catalogue” were cultured in GAM, and out of these, 32 (72%) were successfully cultured. Short-chain fatty acids from the bacterial culture supernatants were then quantified, and bacterial metabolic pathways were predicted based on in silico genomic sequence analysis. Our system provides a useful platform for assessing growth properties and analyzing metabolites of dominant human gut bacteria grown in GAM and supplemented with compounds of interest.

The cultivability in GAM of 44 of the top 56 species listed in the “human gut microbial gene catalogue” was tested; of these, 32 (72%) were successfully cultured.
In the last decade, epidemiological studies and multiple omics studies (e.g. genomics, proteomics, metabolomics) have suggested microbial composition has an impact on the host’s health. For instance, gut microbiota was found to affect the development of the gastrointestinal tract, food digestion and conversion, the host immune response, the energy balance of the host, and as well as play a role in inflammatory bowel disease.Citation1–7) These studies have revealed some of the relationships between the host and microbes; however, there are few cases where the specific microbes or molecules have been identified. Therefore, the current focus in this field has shifted from omics studies toward metabolite(s)-based studies, including the investigation of molecules that play important roles in intestinal symbiosis. These molecules include amino acids, peptides, vitamins, polyamines, and short-chain fatty acids (SCFAs) produced by bacteria.Citation8–12) SCFAs have attracted significant attention due to their potential health benefits. SCFAs are a major energy source for enterocytes, prevent obesity and the metabolic syndrome, have anti-inflammatory and anti-colorectal cancer effects, protect against bacterial toxins, and regulate anxiety and behavior.Citation13–27) However, which microbial species are responsible for the production of these metabolites and how the synthesis of these metabolites is regulated by microbes remain outstanding questions.
In 2010, the identification of the dominant microbe genus/species found in the feces of 124 human subjects established the “human gut microbial gene catalogue.”Citation28) Although bacteria with a small population size must not be ignored, it might be possible to reconstitute microbial composition and metabolic behavior of the gut in vitro using the top-ranked bacteria from the catalog. We found that most (44/56) of the dominant human gut microbes identified in the 2010 studyCitation28) are publicly available from distributors, such as Japan Collection of Microorganisms (JCM), American Type Culture Collection (ATCC), Deutsche Sammlung von Mikroorganismen und Zellkulturen GmbH (DSMZ). However, the recommended media (Table ) by the distributors vary by species and often require cumbersome preparation procedures. For example, ATCC medium 1016 requires boiling of fat-free ground beef and cattle rumen fluid. Additionally, the different constituents of each medium make it difficult to compare metabolic behaviors and growth responses to compounds of interest among species of bacteria.
Table 1. List of bacterial species/strains and description of the media used for culture.
Gifu anaerobic medium (GAM; 1.0% (w/v) peptic digest of animal tissue, 0.3% papaic digest of soybean meal, 1.0% protease peptone, 1.35% digested serum, 0.5% yeast extract, 0.22% beef extract, 0.12% liver extract, 0.3% glucose, 0.25% potassium dihydrogen phosphate, 0.3% sodium chloride, 0.5% soluble starch, 0.03% L-cysteine hydrochloride, and 0.03% sodium thioglycollate, final pH 7.3 ± 0.1)Citation29) is known to be experimentally useful for culturing numerous intestinal bacteria. However, because ATCC and DSMZ did not test GAM, it was unknown whether it could be used to cultivate any of the most dominant human gut microbes. To address the problems associated with media currently used for culturing the dominant human gut microbes, we assessed, whether GAM can serve as a common culture medium for the dominant human gut bacteria. Here, we show that 32 of the 44 publicly obtainable species listed in the “human gut microbial gene catalogue” could be cultured in GAM. In addition, we analyzed the concentrations of SCFAs (i.e. lactic acid, acetic acid, propionic acid, butyric acid) produced by the bacteria. Then, we made a discussion on the SCFAs metabolic pathways of these bacteria, based on the prediction by in silico analysis of their genomic sequences.
Materials and methods
Elimination of dissolved oxygen from GAM
GAM bouillon (#05422), purchased from Nissui Pharmaceutical (Tokyo, Japan), was completely dissolved in deionized water and sterilized by autoclaving at 115 °C for 15 min. When the temperature of the autoclave dropped to 97 °C, GAM was immediately transferred into an anaerobic chamber (INVIVO2 400, Baker Ruskinn, Sanford, ME, USA) and was left overnight at 37 °C to eliminate dissolved oxygen. All procedures for bacterial culture were performed in the anaerobic chamber.
Culture of bacteria in their recommended media and GAM
Bacteria were obtained from ATCC, JCM, and DSMZ (Table ). Recovery of the bacteria was carried out according to the distributors’ instructions. Media other than GAM were prepared according to the methods indicated by the distributors. Bacteria were first cultured in their recommended medium (Table ) at 37 °C under anaerobic conditions (0% O2, 5% H2, 10% CO2, and 85% N2) for 1−5 days. The bacterial cultures grown in the recommended medium were then mixed with sterilized and deoxygenized glycerol (15%) and stored in a −80 °C freezer. Bacteria from the glycerol stock were then inoculated in GAM using a sterilized toothpick. The possibility of contamination of the culture was eliminated by 16S rDNA sequencing as described below. The bacterial cultures grown in GAM were mixed with sterilized and deoxygenized glycerol (15%), aliquoted into 96-well plates, and stored in a −80 °C freezer. Deoxygenation of glycerol was performed using the same protocol as that for deoxygenation of GAM.
Growth curves of bacteria in GAM
Frozen stock-GAM bacterial cultures were completely melted in the anaerobic chamber, and aliquots (5 μL) of melted stocks were pre-cultured in 500 μL of GAM in 96-deep well plates at 37 °C for 1–2 days. The pre-cultures were inoculated in 500 μL of GAM in 96-deep well plates using a copy plate stand (Tokken, Chiba, Japan). Growth was monitored by measuring OD600 by the following protocol. Cultures growing in 96-deep-well plates were mixed by pipetting, and a fraction was removed to measure OD600. The OD600 value of an appropriately diluted culture measured in a cuvette with a 1-cm optical path length was divided by the absorbance at 600 nm of the same sample measured by a microplate reader in a 96-well plate with unknown optical path length. The resulting value was used as a factor to calculate the OD600 value from the raw data obtained by a microplate reader. At the end of culture, cells were pelleted and 16S rDNA sequencing (described below) was performed to confirm the purity of the culture. Culture supernatants were stored at −30 °C until pH and SCFA analyses.
16S rDNA sequencing
Genomic DNAs (gDNAs) were extracted by a beads shocker [Shake Master ver. 1.2 (#BMS-12, Biomedical Science, Tokyo, Japan)] or by phenol-chloroform extraction and subjected to DNA sequencing of the V1–V3 region in the 16S rRNA gene (16S rDNA). Briefly, a DNA fragment encoding 16S rRNA (1500 base pairs) was amplified from gDNAs by PCR using the primer pair: 7f (5′-AGAGTTTGATYMTGGCTCAG-3′) and 1510R (5′-ACGGYTACCTTGTTACGACTT-3′). The purified DNA fragments were used as templates for DNA sequencing with the primer 518R (5′-GTATTACCGCGGCTGCTGG-3′). The obtained sequences were analyzed using a nucleotide basic local alignment search tool (BLAST) program (Blastn; https://blast.ncbi.nlm.nih.gov/Blast.cgi). To confirm the purity of the culture, the waveform representing the nucleotide sequence during Sanger sequencing was visually inspected and confirmed to consist of a single signal. Then, BLAST analysis was performed on the obtained sequence, and if the intended bacterial 16S rDNA sequence was the top hit, the culture was judged to be successful. For two species, Parabacteroides distasonis and Pseudoflavonifractor capillosus, the 16S rDNA sequences of which are significantly polymorphic within each species, the 16S rDNA sequence was cloned into a plasmid vector and 10 resultant plasmids were sequenced for each strain, followed by the BLAST analysis. If only the intended bacterial 16S rDNA sequences were found in the search results, the culture was considered successful.
Quantification of SCFAs
Concentration of SCFAs were measured by high-performance liquid chromatography (HPLC) analysis. Cells cultured in GAM were cleared by centrifugation at 4400 rpm for 40 min at 4 °C. The culture supernatants were diluted 300-fold with water, and aliquots (10 μL) were analyzed using a Dionex ICS-3000 system equipped with an AC11-HC (4 by 250 mm) column (Dionex, Sunnyvale, CA, USA). The system was pre-equilibrated with 1 mM sodium hydroxide (solvent A) for 10 min at a flow rate of 1.5 mL/min. The elution was performed with solvent A and 100 mM sodium hydroxide (solvent B) by a binary gradient. The gradient was performed from 0% of B in 0−8 min, 0 to 10% of B in 8−15 min, and 59% of B in 15−25 min, at a flow rate of 1.5 mL/min.
In silico pathway analysis using Blast.Protein BLAST (Blastp)Citation30) analysis was performed against protein sequences that is obtained from NCBI protein searches, where the name of 32 dominant bacterial species detected in the human gut microbiomeCitation28) were submitted. The accession IDs and the sources of the reference protein sequences used for the Blastp search are listed in Table . Proteins with more than 100- and 300-bit scores were extracted.
Table 2. List of proteins used as the query sequences for Blastp analysis.
Results
Growth of bacteria ranked in the top 56 of the “human gut microbial gene catalogue” in GAM
Among the bacteria ranked in the top 56 of the “human gut microbial gene catalogue,”Citation28) 44 species (79%) were available from ATCC, JCM, and DSMZ (Table ). Of these 44 species, four species (9%) were recommended to be cultured in GAM, and the rest (91%) were recommended to be cultured in the specific media listed in Table (the underlined media were used to recover and store the microbes). The six species indicated with asterisks (*) were cultured in media different from the recommended media because they were previously confirmed in our laboratory to be cultivable in these media. Bacteria inoculated in each medium were cultured at 37 °C in an anaerobic chamber for 1–5 days. The purity of the cultures was confirmed by gDNA extraction followed by 16S rDNA sequencing.
Following pre-culture in the recommended media, bacteria were inoculated in GAM and incubated at 37 °C in an anaerobic chamber for 1–5 days. Of the species studied, 28 species that were cultured in their recommended medium were also able to grow in GAM (Table ). The purity and success of culture in GAM was assessed by 16S rDNA sequencing.
In total, 32 (73%) of the 44 publicly available species identified in the “human gut microbial gene catalogue”Citation28 were successfully cultured in GAM. This corresponds to over half of the top 56 species ranked in the “human gut microbial gene catalogue”Citation28) (32 species; 57%) (Table and Supplemental Fig. S1).
Determination of growth curves of 32 dominant human gut bacteria cultivable in GAM
Supplemental Fig. S2 shows the growth curves of 32 bacterial species in GAM. Almost all of the species (27 species) entered the stationary phase within 1 day. Microbes classified under the phylum Bacteroidetes tended to grow well in GAM. The maximum OD600 values at the stationary phase and the shape of the growth curves were species-dependent.
Analysis of SCFAs produced by 32 dominant human gut bacteria cultivable in GAM
To confirm the usefulness of GAM in the investigation of functional metabolic molecules, SCFAs (i.e. lactic acid, acetic acid, propionic acid, butyric acid) were measured in culture supernatants. Supernatants (60 h) from the cultivable 32 species in GAM were used to measure SCFA concentrations and pH (Fig. and Supplemental Fig. S3).
Fig. 1. Concentration of SCFAs in culture supernatants.
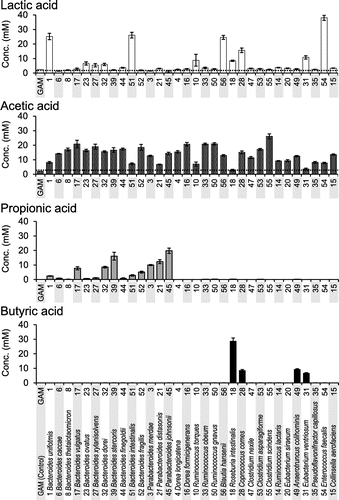
Six of the 32 species (18.8%) produced more than 10 mM lactic acid and the other 26 species produced low levels of lactic acid (Fig. ). E. faecalis, classified in the order Lactobacillales, produced the highest concentration of lactic acid. Acetic acid was detected in culture supernatants of all of the tested species. Thirty species (93.8%) produced more than 10 mM acetic acid when grown in GAM. Most Bacteroides and Parabacteroides species that grew well (Supplementary Fig. S3) in GAM produced significant amounts of acetic acid (Fig. ). All of the tested species belonging to the genera Bacteroides and Parabacteroides produced propionic acid (Fig. ). Butyric acid was detected in the supernatants of four species: Roseburia intestinalis, Coprococcus comes, Anaerotruncus colihominis, and Eubacterium ventriosum (Fig. ), which belong to Clostridiales (Supplemental Fig. S1). The results of in silico analysis of the metabolic pathways in the bacteria were described in Discussion section.
Discussion
In the study of the post-microbiome era, assays using viable dominant human gut bacteria are important and involve extensive bacterial culturing. However, the media recommended by distributors for culturing the dominant human gut bacteria require cumbersome preparation procedures and contain different chemical compounds, preventing cross-species comparisons of bacterial metabolites. In the present study, to reduce the difficulty of preparing the media and to generalize the growth conditions for metabolite analysis, we demonstrated the versatility of GAM as a standard medium for culturing the major species in human gut microbiota. Wu and Gordon et al. developed Megamedium 1.0 as a standard medium for establishing a model of human intestinal microbiota model and cultured 13 species, which included 10 species of the dominant human gut bacteriaCitation31) listed in the “human gut microbial gene catalog.” In the present study, these 10 species could be cultured using GAM (Table ). Compared to Megamedium 1.0 which requires 26 different components, GAM can be simply prepared by dissolving a powder in water. Additionally, it was shown that 32 species, corresponding to a considerable proportion of the dominant human gut bacteria, could be cultured in GAM. GAM has several other advantages over other generally utilized media. For example, it does not require supplementation with horse blood, allowing it to remain transparent after autoclaving and making it easier to monitor growth monitoring at OD600. Furthermore, the use of GAM as a standard medium would allow for the quantification of the metabolites, such as SCFAs, and the standardization of growth conditions would allow elucidation and comparison of bacterial metabolism and responses to various growth conditions.
SCFAs are produced through several pathways. Fig. shows a summary of the metabolic pathways that produce lactic acid, acetic acid, propionic acid, and butyric acid using glucose as a carbon source.Citation22,32,33) Fig. shows the occurrence of key enzymes in the dominant human gut bacteria, which were grown in GAM.
Fig. 2. Pathways responsible for the biosynthesis of SCFAs from glucose in gut microbes.
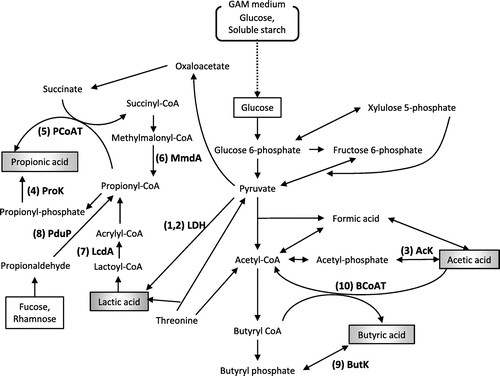
Fig. 3. Occurrence of homologous proteins responsible for SCFA synthesis from glucose in the genomes of dominant human gut bacteria.
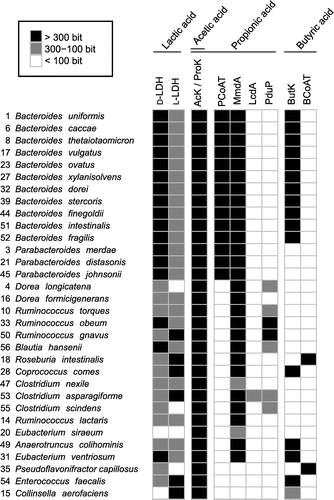
Lactic acid is mainly produced from pyruvate by lactate dehydrogenase (LDH) (Fig. ). Among the 32 bacterial species tested, 25 species produced low levels of lactic acid (Fig. ), despite having a highly conserved LDH homolog (Fig. ). This is surprising because efficient glycolysis under anaerobic conditions generally requires NAD+ formed in the reaction catalyzed by LDH. The growth of B. uniformis and B. intestinalis, whose culture supernatants contained high levels of lactic acid (Fig. ), was lower than that of other species belonging to the same genus, Bacteroides. This suggests that the high concentration of lactic acid might inhibit the growth of B. uniformis and B. intestinalis.
Acetic acid is mainly produced from pyruvate via acetyl-CoA. Acetate kinase (AcK)Citation34) (Fig. ) is an enzyme that catalyzes the last-step of the pathway and is widely conserved in the genomes of the dominant human gut bacteria (Fig. ). It was previously reported that acetic acid derived from probiotic Bifidobacteria is absorbed by the intestinal epithelium of mice and promotes a protective mechanism against pathogenic bacteria such as Escherichia coli O157:H7.Citation13) In the present study, a considerably high concentration (>5 mM) of acetic acid was detected in the culture supernatant of 30 strains of the 32 bacterial species tested, excluding R. intestinalis and E. ventriosum. This result suggests that the most dominant human gut bacteria provide acetic acid to the intestinal epithelium and contribute to the defense mechanism of intestinal epithelial cells.
In the present study, seven bacterial species (22% of tested strains) produced propionic acid at a considerably high concentration (> 5 mM) among the dominant human gut bacteria. They seemed to possess both methylmalonyl-CoA decarboxylase (MmdA) and propionyl-CoA:succinate CoA transferase (PCoAT), which catalyze the biosynthesis of propionic acid (Fig. ). MmdA is involved in the succinate pathway, which is the primary pathway for the production of propionic acid from glucose (Fig. ). The final step in the succinate pathway is a reaction between propionyl-CoA and succinate catalyzed by PCoAT (Fig. ).Citation32,35) Two other pathways producing propionic acid—the acrylate pathway, which uses lactoyl-CoA dehydratase (LcdA), and the propanediol pathway, which uses CoA-dependent propionaldehyde dehydrogenase (PduP)—are also known (Fig. ).Citation33) In the final reaction of the acrylate pathway, phosphoryltransfer of propionyl-CoA by phosphate acetyltransferase results in the formation of propionyl-phosphate, which is eventually converted to propionic acid by propionate kinase (ProK) (Fig. ).Citation25) The reaction catalyzed by PCoAT can also be the another final reaction.Citation33) In the propanediol pathway, both of the reactions catalyzed by PCoAT and ProK, respectively, have a potential to be the final step. The result of Blastp analysis with ProK as a query was all the same as that with AcK as a query. Indeed, it has been shown that the reactions of acetyl-phosphate to form acetic acid and propionyl-phosphate to form propionic acid could be catalyzed by the same kinase (AcK/ProK).Citation36–38) However, propionic acid was undetectable (Fig. ) in the culture supernatants of the species classified under the phyla Firmicutes and Actinobacteria (Supplementary Fig. S1). Almost all species classified under these phyla possess AcK/ProK, and MmdA, LcdA, or PduP homologs, but all of them lack the PCoAT homolog.
Butyric acid, which was detected in the culture supernatant of R. intestinalis, C. comes, A. colihominis, and E. ventriosum in the present study, is formed from two molecules of acetyl-CoA by butyrate kinase (ButK) or butyryl-CoA:acetate CoA-transferase (BCoAT).Citation39) It was previously reported that ButK contributes to butyric acid synthesis in vitro in C. comes, A. colihominis, and E. ventriosum isolated from human feces.Citation39) R. intestinalis was reported to produce butyric acid through a pathway driven by BCoAT instead of ButK.Citation39) The culture supernatant of R. intestinalis had the highest concentration of butyric acid, while acetic acid, which is utilized by the BCoAT pathway, was detected at low concentrations. However, butyric acid was undetectable in the culture supernatants of E. faecalis, C. aerofaciens, and all of the Bacteroides species, despite the presence of ButK homologs. Additionally, P. capillosus, which posesses a BCoAT homolog, did not produce butyric acid.
Recently, it was reported that butyric acid and propionic acid derived from intestinal bacteria belonging to the genus Clostridium play an important role in inhibiting inflammation in the colon in mice through induction of colonic regulatory T cells.Citation20) Although none of the two Clostridium species (C. asparagiforme and C. scindens) tested in the present study produced butyric acid and propionic acid; seven of 32 strains tested produced propionic acid and four produced butyric acid. These results indicate that a proportion of the dominant human gut bacteria regulates the intestinal environment through production of SFCAs, which have an immunoregulatory function and may be important for the symbiotic relationship between the host and the intestinal bacteria.
The results of the SCFA analysis in the present study are consistent with those of the biochemical analysis in previous studiesCitation22) and the in silico analysis of chromosomes of the dominant human gut bacteria in the present study. The SCFA-producing bacteria in the present study possess the genes or their homologs responsible for SCFA production, suggesting that this study is in agreement with previous in vitro enzymatic studies in which the SCFA-related metabolic pathways in several human gut bacteria were identified. However, some bacteria possessing the genes or their homologs involved in SCFA synthesis did not produce the corresponding SCFAs. For example, all of the bacteria of the genus Bacteroides possess a ButK homolog (Fig. ), but none of them produced butyric acid (Fig. ). These results suggest that other factors, such as the presence of certain compounds, immune system of the host, existence of other bacteria are required for the expression of butK in Bacteroides. These stimulating factors need to be identified by a comprehensive in vitro screening using the system developed in this study.
Thus, our system provides a useful platform for assessing the growth properties and analyzing the metabolites of the dominant human gut bacteria under reproducible conditions using GAM as a standardized medium. The approach employed in this study could be used as a model to understand the metabolic behavior of the dominant human gut bacterial species, thereby encouraging species- and metabolite-based studies to better understand the symbiotic relationship between gut microbes and their human hosts.
Author contributions
AG, TK, and SK conceived and designed the experiments. AG, MN, YS, MS, HY, AK, SK performed the experiments. SO performed the in silico analysis. All authors discussed the results for the completion of the manuscript. AG, MN, SO, TK, and SK analyzed the data and wrote the manuscript. SK and TK edited the manuscript.
Funding
This work was supported by Institute for Fermentation (Osaka, Japan) and by the Mitani Foundation for Research and Development.
Disclosure statement
No potential conflict of interest was reported by the authors.
Supplemental materials
The supplemental material for this paper is available at https://doi.org/10.1080/09168451.2017.1359486.
2017.7.18._Supple_Fig_BBB-170317_Gotoh.pdf
Download PDF (291.1 KB)References
- Hooper LV, Midtvedt T, Gordon JI. How host-microbial interactions shape the nutrient environment of the mammalian intestine. Annu Rev Nutr. 2002;22:283–307.10.1146/annurev.nutr.22.011602.092259
- Sommer F, Bäckhed F. The gut microbiota–masters of host development and physiology. Nat Rev Microbiol. 2013;11(4):227–238.10.1038/nrmicro2974
- Gevers D, Kugathasan S, Denson LA, et al. The treatment-naive microbiome in new-onset Crohn’s disease. Cell Host Microbe. 2014;15(3):382–392.10.1016/j.chom.2014.02.005
- Peterson DA, Frank DN, Pace NR, et al. Metagenomic approaches for defining the pathogenesis of inflammatory bowel diseases. Cell Host Microbe. 2008;3(6):417–427.10.1016/j.chom.2008.05.001
- Turnbaugh PJ, Hamady M, Yatsunenko T, et al. A core gut microbiome in obese and lean twins. Nature. 2009;457(7228):480–484.10.1038/nature07540
- Honda K, Littman DR. The microbiome in infectious disease and inflammation. Annu Rev Immunol. 2012;30:759–795.10.1146/annurev-immunol-020711-074937
- Spor A, Koren O, Ley R. Unravelling the effects of the environment and host genotype on the gut microbiome. Nat Rev Microbiol. 2011;9(4):279–290.10.1038/nrmicro2540
- Flint HJ, Bayer EA, Rincon MT, et al. Polysaccharide utilization by gut bacteria: potential for new insights from genomic analysis. Nat Rev Microbiol. 2008;6(2):121–131.10.1038/nrmicro1817
- El Kaoutari A, Armougom F, Gordon JI, et al. The abundance and variety of carbohydrate-active enzymes in the human gut microbiota. Nat Rev Microbiol. 2013;11(7):497–504.10.1038/nrmicro3050
- Woyke T, Teeling H, Ivanova NN, et al. Symbiosis insights through metagenomic analysis of a microbial consortium. Nature. 2006;443(7114):950–955.10.1038/nature05192
- Matsumoto M, Kibe R, Ooga T, et al. Cerebral low-molecular metabolites influenced by intestinal microbiota: a pilot study. Front Syst Neurosci. 2013;7:9.
- Donia MS, Fischbach MA. Small molecules from the human microbiota. Science. 2015;349(6246):1254766.10.1126/science.1254766
- Fukuda S, Toh H, Hase K, et al. Bifidobacteria can protect from enteropathogenic infection through production of acetate. Nature. 2011;469(7331):543–547.10.1038/nature09646
- Fukuda S, Toh H, Taylor TD, et al. Acetate-producing bifidobacteria protect the host from enteropathogenic infection via carbohydrate transporters. Gut Microbes. 2012;3(5):449–454.10.4161/gmic.21214
- Shepherd ML, Ponder MA, Burk AO, et al. Fibre digestibility, abundance of faecal bacteria and plasma acetate concentrations in overweight adult mares. J Nutr Sci. 2014;3:e10.10.1017/jns.2014.8
- Turnbaugh PJ, Ley RE, Mahowald MA, et al. An obesity-associated gut microbiome with increased capacity for energy harvest. Nature. 2006;444(7122):1027–1031.10.1038/nature05414
- Murugesan S, Ulloa-Martínez M, Martínez-Rojano H, et al. Study of the diversity and short-chain fatty acids production by the bacterial community in overweight and obese Mexican children. Eur J Clin Microbiol Infect Dis. 2015;34(7):1337–1346.10.1007/s10096-015-2355-4
- Murphy EF, Cotter PD, Healy S, et al. Composition and energy harvesting capacity of the gut microbiota: relationship to diet, obesity and time in mouse models. Gut. 2010;59(12):1635–1642.10.1136/gut.2010.215665
- Perry RJ, Peng L, Barry NA, et al. Acetate mediates a microbiome–brain–β-cell axis to promote metabolic syndrome. Nature. 2016;534(7606):213–217.10.1038/nature18309
- Furusawa Y, Obata Y, Fukuda S, et al. Commensal microbe-derived butyrate induces the differentiation of colonic regulatory T cells. Nature. 2013;504(7480):446–450.10.1038/nature12721
- Csordas A. Butyrate, aspirin and colorectal cancer. Eur J Cancer Prev. 1996;5(4):221–231.10.1097/00008469-199608000-00002
- Louis P, Hold GL, Flint HJ. The gut microbiota, bacterial metabolites and colorectal cancer. Nat Rev Microbiol. 2014;12(10):661–672.10.1038/nrmicro3344
- Hamer HM, Jonkers D, Venema K, et al. Review article: the role of butyrate on colonic function. Aliment Pharmacol Ther. 2008;27(2):104–119.
- Berni CR, Di Costanzo Μ, Λeone L. The epigenetic effects of butyrate: potential therapeutic implications for clinical practice. Clin Epigenetics. 2012;4(1):4.10.1186/1868-7083-4-4
- Hosseini E, Grootaert C, Verstraete W, et al. Propionate as a health-promoting microbial metabolite in the human gut. Nutr Rev. 2011;69(5):245–258.10.1111/nure.2011.69.issue-5
- Vinolo MAR, Rodrigues HG, Nachbar RT, et al. Regulation of inflammation by short chain fatty acids. Nutrients. 2011;3(10):858–876.10.3390/nu3100858
- Arora T, Sharma R, Frost G. Propionate. Anti-obesity and satiety enhancing factor? Appetite. 2011;56(2):511–515.10.1016/j.appet.2011.01.016
- Qin J, Li R, Raes J, et al. A human gut microbial gene catalogue established by metagenomic sequencing. Nature. 2010;464(7285):59–65.10.1038/nature08821
- Yamamoto-Osaki T, Kamiya S, Sawamura S, et al. Growth inhibition of Clostridium difficile by intestinal flora of infant faeces in continuous flow culture. J Med Microbiol. 1994;40(3):179–187.10.1099/00222615-40-3-179
- Camacho C, Coulouris G, Avagyan V, et al. BLAST+: architecture and applications. BMC Bioinf. 2009;10:421.10.1186/1471-2105-10-421
- Wu M, McNulty NP, Rodionov DA, et al. Genetic determinants of in vivo fitness and diet responsiveness in multiple human gut Bacteroides. Science. 2015;350(6256):aac5992–aac5992.10.1126/science.aac5992
- Rios-Covian D, Sánchez B, Salazar N, et al. Different metabolic features of Bacteroides fragilis growing in the presence of glucose and exopolysaccharides of bifidobacteria. Front Microbiol. 2015;6:825.
- Reichardt N, Duncan SH, Young P, et al. Phylogenetic distribution of three pathways for propionate production within the human gut microbiota. ISME J. 2014;8(6):1323–1335.10.1038/ismej.2014.14
- Louis P, Duncan SH, McCrae SI, et al. Restricted distribution of the butyrate kinase pathway among butyrate-producing bacteria from the human colon. J Bacteriol. 2001;186(7):2099–2106.
- Haller T, Buckel T, Rétey J, et al. Discovering new enzymes and metabolic pathways: conversion of succinate to propionate by Escherichia coli†. Biochemistry. 2000;39(16):4622–4629.10.1021/bi992888d
- Diez-Gonzalez F, Russell JB, Hunter JB. The acetate kinase of Clostridum acetobutylicum strain P262. Arch Microbiol. 1996;166(6):418–420.10.1007/BF01682990
- Chittori S, Savithri HS, Murthy MRN, et al. Structural and mechanistic investigations on Salmonella typhimurium acetate kinase (AckA): identification of a putative ligand binding pocket at the dimeric interface. BMC Struct Biol. 2012;12(1):24.10.1186/1472-6807-12-24
- Hesslinger C, Fairhurst SA, Sawers G. Novel keto acid formate-lyase and propionate kinase enzymes are components of an anaerobic pathway in Escherichia coli that degrades L-threonine to propionate. Mol Microbiol. 1998;27(2):477–492.10.1046/j.1365-2958.1998.00696.x
- Duncan SH, Barcenilla A, Stewart CS, et al. Acetate utilization and butyryl coenzyme A (CoA): acetate-CoA transferase in butyrate-producing bacteria from the human large intestine. Appl Environ Microbiol. 2002;68(10):5186–5190.10.1128/AEM.68.10.5186-5190.2002
- Charrier C, Duncan GJ, Reid MD, et al. A novel class of CoA-transferase involved in short-chain fatty acid metabolism in butyrate-producing human colonic bacteria. Microbiology. 2006;152(Pt 1):179–185.10.1099/mic.0.28412-0