ABSTRACT
Japanese apricot, Prunus mume Sieb. et Zucc., biosynthesizes the l-phenylalanine-derived cyanogenic glucosides prunasin and amygdalin. Prunasin has biological properties such as anti-inflammation, but plant extraction and chemical synthesis are impractical. In this study, we identified and characterized UGT85A47 from Japanese apricot. Further, UGT85A47 was utilized for prunasin microbial production. Full-length cDNA encoding UGT85A47 was isolated from Japanese apricot after 5ʹ- and 3ʹ-RACE. Recombinant UGT85A47 stoichiometrically catalyzed UDP-glucose consumption and synthesis of prunasin and UDP from mandelonitrile. Escherichia coli C41(DE3) cells expressing UGT85A47 produced prunasin (0.64 g/L) from racemic mandelonitrile and glucose. In addition, co-expression of genes encoding UDP-glucose biosynthetic enzymes (phosphoglucomutase and UTP-glucose 1-phosphate uridiltransferase) and polyphosphate kinase clearly improved prunasin production up to 2.3 g/L. These results showed that our whole-cell biocatalytic system is significantly more efficient than the existing prunasin production systems, such as chemical synthesis.
Graphical Abstract

Prunasin production using engineered Escherichia coli expressing UGT85A47 from Japanese apricot.
Plants biosynthesize numerous glycosides, which often provide defensive functions. Glycosides are used as medicines and food additives, because they exhibit important biological activities [Citation1]. Cyanogenic glycosides (CNglcs) are amino acid-derived natural products that are present in more than 3000 different plant species [Citation2]. When plant tissues are disrupted, CNglcs are sequentially degraded into the corresponding carbonyl compounds and hydrogen cyanide (HCN) by a combination of β-glucosidase and hydroxynitrile lyase [Citation3,Citation4]. As HCN inhibits cytochrome c-oxidase in the cellular respiration system [Citation5], stored CNglcs play a crucial role in defense against herbivores and infection by microorganisms. Biosynthesis of one CNglc, dhurrin, has been extensively studied in sorghum, Sorghum bicolor (L.) Moench. In this case, l-tyrosine is converted into (S)-4-hydroxymandelonitrile via 4-hydroxyphenylacetaldoxime by two multifunctional cytochrome P450s, CYP79A1 and CYP71E1 [Citation6–Citation8]. Subsequently (S)-4-hydroxymandelonitrile is glucosylated into the stable storage form, dhurrin, by a uridine diphosphate (UDP) glucosyltransferase (UGT), UGT85B1 [Citation9]. Rosaceae family members, such as Japanese apricot, almond, apple, and sweet cherry, contain a glucoside and gentiobioside of (R)-mandelonitrile, called prunasin and amygdalin respectively [Citation10,Citation11]. Recently, we identified two cytochrome P450s from Japanese apricot, CYP79D16 and CYP71AN24, that catalyze the conversion of l-phenylalanine into (R)-mandelonitrile via phenylacetaldoxime [Citation12]. Although it has been reported that UGT85A19 from almond catalyzes the conversion of (R)-mandelonitrile into prunasin [Citation13], the UGT that catalyzes this reaction in Japanese apricot has not been identified.
l-phenylalanine-derived CNglcs are bioactive compounds with biological activities such as anti-inflammation [Citation14] and DNA polymerase β inhibition [Citation15]. Rosaceae seeds contain a high concentration of amygdalin but a low concentration of prunasin. In Japanese apricot, its seeds contain 33–46 mg/g fresh weight of amygdalin but 0.2–0.4 mg/g fresh weight of prunasin [Citation16]. Therefore, the availability of prunasin from plants is limited. A few attempts at prunasin chemical synthesis have been reported [Citation17,Citation18]. However, efficiency and control of stereochemistry is insufficient for commercial production. Biocatalytic methods are a potential alternative to the synthesis of specialized plant metabolites when the use of chemical synthesis is impractical [Citation19]. Biotransformation of flavonoids and terpenoids using Escherichia coli expressing plant UGTs results in the production of large amounts of flavonoid and terpenoid glucosides [Citation19,Citation20]. However, biocatalytic methods using E. coli expressing UGTs have not been applied to CNglcs production.
In this study, we cloned and characterized the cDNA encoding UGT85A47 from Japanese apricots, which catalyze the glucosylation of mandelonitrile to produce prunasin. Furthermore, E. coli harboring UGT85A47, with the aid of improved UDP-glucose biosynthetic pathway, was applied to prunasin production from mandelonitrile.
Materials and methods
Analytical methods
Prunasin, mandelonitrile, and benzaldehyde were quantified using a Nexera Ultra Fast Liquid Chromatography System (Shimadzu, Kyoto, Japan) equipped with a COSMOCIL 2.5C18-MS-II column (75 mm × 2.0 mm i.d.; Nacali Tesuque) under the following condition: mobile phase A, 0.1% formic acid in water; mobile phase B, 0.1% formic acid in acetonitrile and a 5–50% linear gradient of B for 3.5 min then maintained at 50% for 0.5 min with a flow rate of 0.4 mL min−1 at 40°C. Prunasin and mandelonitrile were assessed at 210 nm. Benzaldehyde was monitored at 248 nm. To separate prunasin from its epimer sambunigrin, both compounds were analyzed using a Prominence High Performance Liquid Chromatography System (Shimadzu) equipped with a CYCLOBOND I 2000 RSP Chiral HPLC column (250 mm x 4.6 mm i.d.; Sigma-Aldrich, St. Louis, MO, USA) under the following condition: 20% MeOH at a flow rate of 1.0 ml min−1 at 30°C. Prunasin and sambunigrin were assessed at 210 nm. An epimeric mixture of prunasin and sambunigrin was generated by alkalinizing a 10 mM prunasin solution to pH 11 with NaOH at 30°C for 1 h, followed by neutralization with HCl. These methods are similar to previous publications [Citation13,Citation21]. UDP-glucose and UDP were quantified using a Prominence High Performance Liquid Chromatography System equipped with a COSMOCIL PBr column (150 mm x 4.6 mm i.d.; Nacalai Tesuque) under the following condition: 100 mM sodium phosphate buffer (pH 7.0) with a flow rate of 1.0 mL min−1 at 30°C. UDP and UDP-glucose were assessed at 260 nm.
Molecular cloning of UGT from japanese apricot
To obtain cDNA encoding UGT from Japanese apricot, orthologues of UGT85A19 from the genome sequence of Japanese apricot [Citation22] were identified by the tblastn [Citation23]. Total RNA was prepared from seedlings of the Japanese apricot “Nanko” as previously described [Citation12], using the Plant RNA Reagent (Thermo Fisher Scientific, Waltham, MA, USA). cDNA was synthesized using the GeneRacer Cloning Kit (Thermo Fisher Scientific). In cDNA cloning, primers for 5ʹ- and 3ʹ-RACE were designed based on a UGT85A19 orthologue of Japanese apricot (GenBank: XM_008221235). PCR products were cloned into the pCR-blunt vector (Thermo Fisher Scientific) and sequenced. The UGT coding sequence was amplified by PCR using the Tks Gflex DNA Polymerase (Takara, Shiga, Japan) and gene-specific primers designed based on the RACE products. Amplified products were cloned into the pCR-blunt vector (Thermo Fisher Scientific). More than four independent clones were sequenced to confirm their sequences. The cDNA sequence was deposited into the DDBJ/EMBL/GenBank databases under the accession number LC189567. Oligonucletide primers used in this study are summarized in Table S1.
RT-PCR
RT-PCR was performed using cDNA prepared from seedlings, kernels, leaves, and buds. ExTaq (Takara) was used as a Taq DNA polymerase. PCR conditions were as follows: 98°C for 60 s, 32 cycles at 98°C for 10 s, 52.5°C for 20 s, and 72°C for 30 s. The gene encoding actin served as an internal control. PCR products were electrophoresed on agarose gels containing the GelGreen (Biotium, Hayward, CA, USA), and visualized under a green LED transilluminator. Images were cropped with the Illustrator CS6 Extended (Adobe, San Jose, CA, USA).
Production of recombinant UGT85A47 in E. coli
To produce UGT85A47, as an N-terminal His-tagged protein in E. coli, the coding sequence of UGT85A47 was re-amplified by PCR and cloned into NdeI-HindIII site of the pET-28a (+) vector (Novagen, Madison, WI, USA) using the In-Fusion HD Cloning kit (Clontech Laboratories, Palo Alto, CA, USA). After confirmation of inserted DNA sequence, the resultant plasmid (pET28-UGT85A47) was used to transform E. coli BL21(DE3). The transformant (BL21-UGT85A47) was inoculated into LB containing 1% (w/v) glucose and kanamycin (50 µg/mL) and cultured overnight at 37°C. The culture was transferred to 100-times the volume of TB-based-autoinduction medium (1.2% tryptone, 2.4% yeast extract, 0.22% KH2PO4, 0.94% K2HPO4, 0.6% glycerol, 0.05% glucose, 0.2% lactose) containing kanamycin (50 µg/mL). After incubation at 26°C for 24 h, cells were harvested, resuspended in 10 mM Tris-HCl (pH 8.0) containing 100 mM NaCl and 20 mM imidazole, and disrupted by ultrasonication. Insoluble materials were removed by centrifugation and supernatant was recovered as cell free extract (CFE). CFE was loaded into a HisTrap HP column (GE Healthcare, Buckinghamshire, United Kingdom) equilibrated with 10 mM Tris-HCl (pH 8.0) containing 100 mM NaCl and 20 mM imidazole. After a wash using the same buffer, the recombinant UGT85A47 was eluted with a linear gradient of imidazole (20 mM to 300 mM) in the same buffer. The active fractions were pooled and concentrated using a centrifugal filtration device (Amicon Ultra-4 Centrifugal Filter Unit, MWCO 10 kDa: Millipore). The protein was loaded onto a Superdex 200 10/300 GL gel filtration column (GE Healthcare) equilibrated with phosphate buffered saline (PBS). Protein concentration was determined using a protein assay kit (Bio-Rad Laboratories, Hercules, CA, USA) with bovine serum albumin as the standard.
Mandelonitrile glucosyltransferase activity assay
A 200-µL reaction mixture containing UGT85A47, 50 mM citrate buffer (pH 4.0), 10 mM racemic mandelonitrile, and 10 mM UDP-glucose was incubated at 30°C. Twenty microliters of the reaction mixture was mixed with 380 µL of 0.1% formic acid in 25% acetonitrile and centrifuged to precipitate insoluble materials. Quantification and chirality assignment of the reaction products were performed as described in the “Analytical methods”.
Effect of pH and temperature on recombinant UGT85A47
To evaluate the effect of pH on UGT85A47 activity, the enzyme activity was assessed in buffers of varied pH (pH 3.0 to 10.5) at 25°C. The pH stability was determined by measuring the remaining activity after incubation in buffers of varied pH (3.0–10.5) at 25°C for 1 h. Sodium citrate buffer (pH 3.0 to 6.0, 50 mM), potassium phosphate (KPB; pH 6.0 to 7.5, 50 mM), Tris-HCl (pH 7.1 to 9.0, 50 mM), and CAPS-NaOH (pH 9.0 to 10.5, 50 mM) buffers were used. The effect of temperature on UGT85A47 activity was assessed between 15 and 50°C. The thermostability was evaluated by pre-incubating the UGT85A47 at different temperatures (15°C to 50°C) for 1 h in 10 mM KPB (pH 7.0), and then the residual enzyme activity was determined.
Measurement of the kinetic parameters of UGT85A47
Various concentrations of racemic mandelonitrile (50–2000 µM) or UDP-glucose (0.2–10 mM) were used to plot the substrate saturation curve for kinetic study of the enzyme under the standard assay conditions. Steady-state kinetic parameters were calculated using the KaleidaGraph version 4.1 (Synergy Software, Reading, PA, USA), fitting the data to the Michaelis-Menten equation.
Construction of UDP-glucose biosynthetic enzyme gene co-expression plasmids
To enhance UDP-glucose biosynthesis from glucose in E. coli, genes encoding UDP-glucose biosynthetic enzymes and polyphosphate kinase co-expression plasmids were constructed. The genes encoding glucokinase (Glk; NP_416889), phosphoglucomutase (Pgm; NP_415214), UTP-glucose-1-phosphate uridiltransferase (GalU; NP_415752), and polyphosphate kinase (PPK; NP_416996) were amplified from the genomic DNA of E. coli JM109 by PCR using the Tks Gflex DNA polymerase (Takara). Amplified products were cloned into the NcoI-HindIII site of pET28-a (+) using the InFusion HD cloning kit. After the confirmation of the inserted DNA sequences, each gene with T7 promoter and terminator regions were amplified by PCR using the PrimeSTAR MAX DNA Polymerase (Takara). Amplified products were assembled into the pCDF-1b (Clontech) using the Gibson Assembly Master Mix (New England Biolabs, Ipswich, MA, USA). The resultant plasmid was designated pCDF-GPGP. To remove the glk expression cassette from the plasmid, inverse PCR and self-ligation were performed. The resultant plasmids were designated pCDF-PGP. Plasmids used in this study are summarized in .
Table 1. Plasmids and bacterial strains used in this study.
Whole-cell biotransformation
The plasmids pCDF-1b, pCDF-PGP or pCDF-GPGP, and pET28-UGT85A47 were used to co-transform E. coli BL21(DE3) or C41(DE3). These transformants were inoculated into LB containing 1% glucose, kanamycin (50 µg/mL), and streptomycin (50 µg/mL) and then incubated overnight at 37°C. Cultures were transferred to 100-times the volume of TB-autoinduction medium and cultured at 30°C for 24 h. After culture, cells were harvested and resuspended in 100 mM KPB (pH 7.4). To compare the prunasin producing ability of each transformant, a 200-µL reaction mixture containing 100 mg/mL of wet cells, 10 mM MgSO4, 30 mM racemic mandelonitrile, 150 mM glucose, 0.1% (v/v) polyphosphate (PPn), and 100 mM KPB (pH 7.0) was incubated at 30°C while being shaken. After the 24 h, prunasin was quantified as described above. Bacterial strains used in this study are summarized in .
Results and discussion
Molecular cloning of UGT85A47 from Japanese apricot
From Japanese apricot, we identified two cytochrome P450s, CYP79D16 and CYP71AN24, that catalyze the conversion of l-phenylalanine into mandelonitrile via phenylacetaldoxime [Citation12]. In these plants, mandelonitrile is further converted to prunasin and amygdalin for storage. However, the UGTs that catalyze the glucosylation steps in Japanese apricot have not been identified. To identify the mandelonitrile glucosyltransferase from Japanese apricot, the genome sequence of Japanese apricot [Citation22] was searched, using tblastn, with the almond UGT85A19 (GenBank: ABV68925) query sequence, since UGT85A19 has mandelonitrile glucosyltranferase activity [Citation13]. We identified 17 genes encoding UGT85A in the Japanese apricot genome sequence. Among them, we selected a UGT85A19 orthologue (GenBank XM_008221235; 7-deoxyloganetin glycosyltransferase-like), since it had the highest similarity with UGT85A19. The UGT85A19 orthologue cloned from Japanese apricot “Nanko” had four nucleotide substitutions and two amino acid substitutions compared to the sequence in the database. The identified UGT was assigned as UGT85A47 by the UGT nomenclature committee [Citation24]. UGT85A47 shared 99% and 46% identities at the amino acid sequence level with UGT85A19 from almond and UGT85B1 from sorghum, respectively.
To localize expression of UGT85A47, CYP79D16, and CYP71AN24 at the tissue level, RT-PCR was performed. UGT85A47 was expressed in the seedling, kernel, leaf, and bud (), whereas CYP79D16 and CYP71AN24 were expressed specifically in the seedling, as previously reported [Citation12]. Expression of UGT85A47 in various tissues aligns with the mandelonitrile glucosyltransferase activity detected in various almond tissues [Citation25]. These results indicated that biosynthesis of l-phenylalanine-derived CNglcs in Japanese apricot is regulated at the expression of CYP79D16 and CYP71AN24, but not the expression of UGT85A47.
Figure 1. Expression analysis of UGT85A47, CYP79D16, and CYP71AN24 in Japanese apricot by RT-PCR. cDNA synthesized from kernels, seedlings, leaves, and buds was used for RT-PCR. Gene encoding actin served as an internal control.
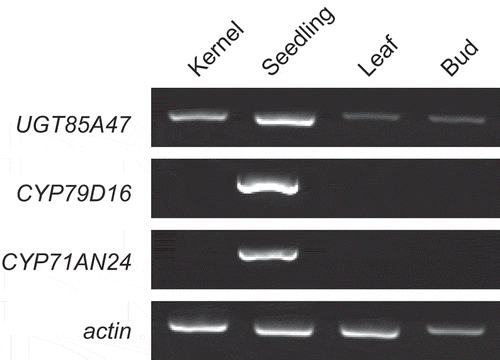
Characterization of the recombinant UGT85A47
In order to biochemically characterize UGT85A47, it was produced as an N-terminal His-tagged protein in E. coli and purified. The purified enzyme showed a single band on SDS-PAGE with a molecular mass of 52,000 and relative molecular mass of the enzyme was determined to be 61,500 by gel filtration, indicating that this enzyme functions as a monomer. The recombinant UGT85A47 stoichiometrically catalyzed consumption of mandelonitrile and UDP-glucose and synthesis of mandelonitrile-glucoside and UDP (). Since mandelonitrile has a chiral center, mandelonitrile-glucoside has two epimeric forms, prunasin and sambunigrin. UGT85A17 from the almond produces prunasin as a major product from racemic mandelonitrile [Citation13]. Therefore, we analyzed the reaction products using HPLC equipped with a chiral column. As shown in , prunasin was detected but sambunigrin was not, indicating that UGT85A47 acts on (R)-mandelonitrile but not on (S)-mandelonitrile.
Figure 2. Production of prunasin by recombinant UGT85A47. (a) Time course of prunasin production and mandelonitrile consumption. Benzaldehyde was quantified as a decomposed product of mandelonitrile. (b) Time course of UDP production and UDP-glucose consumption. (c) Chiral HPLC analysis of prunasin. Prunasin and its epimer sambunigrin were separated using a chiral column (CYCLOBOND I 2000 RSP Chiral HPLC column). An epimeric mixture of prunasin was obtained by alkalinization of the prunasin solution.

To further characterize UGT85A47, the effects of pH and temperature on enzyme catalysis were elucidated. UGT85A47 had two pH optimums at 4.0 and 9.0–9.5 (). Although the enzyme activity at 9.0–9.5 was higher than pH 4.0, we selected pH 4.0 for latter enzyme assays, since mandelonitrile is unstable to degrade benzaldehyde and HCN at higher pH [Citation26]. Optimum temperature was 30°C (). After a 1-hour incubation at 25°C, UGT85A47 retained its activity over a pH range of 4.0 to 9.5 (). After a 1-hour incubation in 10 mM KPB (pH 7.0), UGT85A47 retained its activity between 15°C and 40°C (). At the optimum condition, pH 4.0 and 30°C, UGT85A47 showed a Km of 283 ± 69 µM and 2.3 ± 0.4 mM toward racemic mandelonitrile and UDP-glucose respectively.Vmax and kcat were 8.0 ± 0.6 µmol/min/mg and 7.4 s−1 respectively. UGT85A47 catalytic efficiency (kcat/Km) for racemic mandelonitrile was 26,100 s−1 M−1. Although kinetic parameters of UGT85A19 has not been reported, the catalytic efficiency of UGT85A47 was superior to that of UGT85B1 from sorghum (Km = 840 µ M ; kcat = 10.6 s−1; kcat/Km= 12,600 s−1 M−1) [Citation27].
Figure 3. Effect of pH and temperature on recombinant UGT85A47.(A) Optimum pH. Reactions were performed at 25°C in the following buffers: 50 mM sodium citrate buffer (pH 3.0 to 6.0), 50 mM potassium phosphate buffer (KPB; pH 6.0 to 7.5), 50 mM Tris-HCl buffer (pH 7.1 to 9.0), and 50 mM CAPS-NaOH buffer (pH 9.0 to 10.5). (b) Optimum temperature. Reactions were performed in 50 mM sodium citrate buffer (pH 4.0) at various temperatures (15°C to 50°C). (c) pH stability. Remaining activity was measured after incubation at 25°C for 60 min in the pH range of 3.0–10.5 without substrate. (d) Temperature stability. Remaining activity was measured after incubation at 15–50°C for 60 min in 100 mM KPB (pH 7.0) without substrate. Values are presented as the mean ± SD (n = 4).
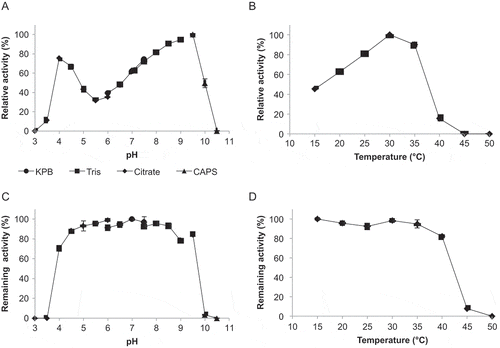
The substrate specificity of UGT85A47 was examined using potential acceptor molecules and nucleotide-sugar donor molecules. UGT85A47 acted on (S)-1-phenyl-2-propyn-1-ol, (R,S)-2-cyclohexyl-2-hydroxyacetonitrile, (R,S)-1-phenylethanol, and (R)-mandelic acid with relative activity of 59.2, 15.4, 9.0, and 5.5% of (R,S)-mandelonitrile respectively (). UGT85A47 utilized UDP-glucose as nucleotide-sugar donor but not UDP-galactose, UDP-glucuronic acid, UDP-N-acetylglucosamine, or UDP-N-acetylgalactosamine. The broad substrate specificity of UGT85A47 toward acceptor molecules aligns with that of UGT85B1 from sorghum and UGT85K4 and UGT85K5 from cassava catalyze glucosylations of α-hydroxynitriles into CNglcs with broad substrate specificity [Citation27,Citation28]. In sorghum CYP79A1, CYP71E1, UGT85B1, and cytochrome P450 reductase are thought to be form metabolon [Citation29] that increase the efficiency of metabolic pathways by channeling substrates between enzymes [Citation30]. Although UGT85A47 has broad substrate specificity, the enzyme may specifically act on (R)-mandelonitrile converted from l-phenylalanine by CYP79D17 and CYP71AN24 to produce prunasin in Japanese apricot.
Table 2. Substrate specificity of UGT85A47.
Microbial production of prunasin
Prunasin is a bioactive compound with important biological activities. However, extraction from plants and chemical synthesis are impractical. Since the R- and S-configurations of CNGlcs can have significantly different biochemical and physiological properties [Citation31], biocatalytic methods using stereoselective UGT85A47 are good alternatives for prunasin production. Since UDP-glucose is prohibitively expensive to be used in stoichiometric ratio for prunasin production from mandelonitrile by UGT85A47 in vitro, using whole cells as catalysts where UDP-glucose generated in situ is preferred [Citation32]. First, E. coli BL21(DE3) and E. coli C41(DE3) containing UGT85A47 were employed to produce prunasin. After incubation of E. coli cells with racemic mandelonitrile and glucose, we quantified prunasin in the reaction mixture. C41(DE3) produced 2.2 mM (0.64 g/L) of prunasin, which was two-times higher than 1 mM (0.29 g/L) produced by BL21(DE3). Considering that expression level of UGT85A47 was similar in both strains (Figure S1), the difference of prunasin production level might be due to the differences in the other factors, such as the supply of UDP-glucose and the permeability of substrate.
The intracellular UDP-glucose pool in E. coli is approximately 2.5 mM [Citation33], which is near the Km value of UGT85A47 for UDP-glucose. During catalysis, insufficient UDP-glucose generation depletes UDP-glucose in E. coli cells. Therefore, we enhanced the UDP-glucose biosynthetic pathway to improve prunasin production in E. coli C41(DE3). UDP-glucose is biosynthesized from glucose via glucose 6-phosphate and glucose 1-phosphate by Glk, Pgm, and GalU (). Glk and GalU utilize ATP and UTP respectively. PPK is often utilized to regenerate NDP into NTP using PPn [Citation34,Citation35], since the intracellular pool of ATP and UTP is also limited. Based on these information, glk, pgm, galU, and ppk were cloned from E. coli and the pCDF-1b based vectors, containing multi-monocistronic operon systems with or without glk, were generated for efficient production of UDP-glucose in E. coli cells (). In order to evaluate the effects of gene overexpression encoding UDP-glucose biosynthetic enzymes and PPK in E. coli C41(DE3), intact cells from transformants were incubated with racemic mandelonitrile in the presence of glucose and PPn. C41-85A47-GPGP and C41-85A47-PGP clearly improved prunasin production to 4.9 mM (1.4 g/L) and 7.8 mM (2.3 g/L), respectively (). Glucose 6-phospahte converted from glucose by Glk is a branching point of glycolysis and UDP-glucose biosynthesis. When glk was not overexpressed, metabolic flow was likely concentrated on UDP-glucose biosynthesis resulting in higher prunasin production level with C41-85A47-PGP. These results suggested that C41-85A47-PGP has potential for improved prunasin production.
Figure 4. Schematic representation of prunasin biosynthetic pathway in E. coli.(a) Prunasin production from mandelonitrile coupled with UDP-glucose biosynthetic enzymes and the regeneration system of ATP and UTP. Glk, glucokinase; Pgm, phosphoglucomutase; GalU, glucose 1-phosphate uridyltransferase; PPK, polyphosphate kinase. (b) Schematic representation of pCDF-1b based on multi-monocistronic expression cassettes for the biosynthesis of UDP-glucose.
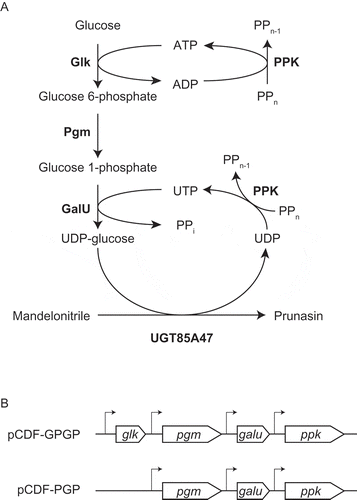
Figure 5. Microbial production of prunasin by E. coli cells.E. coli cells harboring the UGT85A47 expression plasmid, with or without the UDP-glucose biosynthetic gene expression plasmid, were incubated with racemic mandelonitrile and glucose.The error bars represent the standard deviation of four replicates.

Conclusions
In this study, we identified and characterized UGT85A47 catalyzing the glucosylation of (R)-mandelonitrile from Japanese apricot. Further, the enzyme was utilized for microbial prunasin production with the aid of UDP-glucose biosynthetic enzymes. RT-PCR showed that UGT85A47 was expressed in various tissues (). In addition, recombinant UGT85A47 acted on (R)-mandelonitrile to produce prunasin () with broad substrate specificity (). Biosynthesis of prunasin and amygdalin in Japanese apricot is regulated at the level of gene expression and substrate specificity of mandelonitrile biosynthetic cytochrome P450s, rather than that of UGT85A47. E. coli C41(DE3) cells carrying the UGT85A47 expression plasmid (pET28-UGT85A47) produced prunasin (0.64 g/L) from racemic mandelonitrile and glucose. To improve prunasin production, we constructed the UDP-glucose biosynthetic enzymes genes (pgm, galU, and ppk) co-expression vector, pCDF-PGP (). Prunasin production with UGT85A47-expressing E. coli C41(DE3) was significantly elevated to 2.3 g/L by co-overexpression of pgm, galU, and ppk (). Our constructed pCDF-PGP can be applied to the production of other biologically important glucosides by combining it with other UGTs expression plasmids.
Author contributions
T.Y. and Y.A. conceived and designed the study; T.Y. performed the experiment; T.Y. and Y.A. wrote the manuscript.
FigureS1_SDS-Page_Yamaguchi-Asano_.docx
Download MS Word (312.5 KB)Acknowledgments
We thank Ms. Sayuri Ohno for technical assistance. This study was supported by the Exploratory Research for Advanced Technology Program (ERATO) Asano Active Enzyme Molecule Project from the Japan Science and Technology Agency (Grant number: JPMJER1102). This research was also supported in part by a grant-in-aid for Scientific Research (S) from The Japan Society for Promotion of Sciences (Grant number: 17H06169) to Y. Asano.
Disclosure statement
No potential conflict of interest was reported by the authors.
Supplemental Material
Supplemental data for this article can be accessed here.
Additional information
Funding
References
- Facchini PJ, Bohlmann J, Covello PS, et al Synthetic biosystems for the production of high-value plant metabolites. Trends Biotechnol. 2012;30(3):127–131.
- Gleadow RM, Møller BL. Cyanogenic glycosides: synthesis, physiology, and phenotypic plasticity. Annu Rev Plant Biol. 2014;65:155–185.
- Dadashipour M, Asano Y. Hydroxynitrile lyases: insights into biochemistry, discovery, and engineering. ACS Catal. 2011;1(9):1121–1149.
- Asano Y, Kawahara N. A new S-hydroxynitrile lyase from baliospermum montanum—its structure, molecular dynamics simulation, and improvement by protein engineering. Ind Biotechnol. 2016;12(2):91–97.
- Price NR. The mode of action of fumigants. J Stored Prod Res. Elsevier. 1985;21(4):157–164.
- Sibbesen O, Koch B, Halkier BA, et al Isolation of the heme-thiolate enzyme cytochrome P-450TYR, which catalyzes the committed step in the biosynthesis of the cyanogenic glucoside dhurrin in Sorghum bicolor (L.) Moench. Proc Natl Acad Sci USA. 1994;91(21):9740–9744.
- Maria Koch B, Sibbesen O, Halkier BA, et al The primary sequence of cytochrome p450tyr, the multifunctional n-hydroxylase catalyzing the conversion of l-tyrosine to p-hydroxyphenylacetaldehyde oxime in the biosynthesis of the Cyanogenic Glucoside Dhurrin in Sorghum Bicolor (L.) Moench. Arch Biochem Biophys. 1995;323(1):177–186.
- Bak S, Kahn RA, Nielsen HL, et al Cloning of three A-type cytochromes P450, CYP71E1, CYP98, and CYP99 from Sorghum bicolor (L.) Moench by a PCR approach and identification by expression in Escherichia coli of CYP71E1 as a multifunctional cytochrome P450 in the biosynthesis of the cyanogenic glucoside dhurrin. Plant Mol Biol. 1998;36(3):393–405.
- Thorsøe KS, Bak S, Olsen CE, et al Determination of catalytic key amino acids and UDP sugar donor specificity of the cyanohydrin glycosyltransferase UGT85B1 from Sorghum bicolor. Molecular modeling substantiated by site-specific mutagenesis and biochemical analyses. Plant Physiol. 2005;139(2):664–673.
- Del Cueto J, Ionescu IA, Pičmanová M, et al Cyanogenic glucosides and derivatives in almond and sweet cherry flower buds from dormancy to flowering. Front Plant Sci. 2017;8:800.
- Bolarinwa IF, Orfila C, Morgan MRA. Amygdalin content of seeds, kernels and food products commercially-available in the UK. Food Chemistry. 2014;152:133–139.
- Yamaguchi T, Yamamoto K, Asano Y. Identification and characterization of CYP79D16 and CYP71AN24 catalyzing the first and second steps in L-phenylalanine-derived cyanogenic glycoside biosynthesis in the Japanese apricot, Prunus mume Sieb. et Zucc. Plant Mol Biol. 2014;86(1–2):215–223.
- Franks TK, Yadollahi A, Wirthensohn MG. A seed coat cyanohydrin glucosyltransferase is associated with bitterness in almond (Prunus dulcis) kernels. Func Plant Biol. 2008;35(3):236.
- Paoletti I, De Gregorio V, Baroni A, et al Amygdalin analogues inhibit IFN-γ signalling and reduce the inflammatory response in human epidermal keratinocytes. Inflammation. 2013;36(6):1316–1326.
- Mizushina Y, Takahashi N, Ogawa A, et al The cyanogenic glucoside, prunasin (D-mandelonitrile-beta-D-glucoside), is a novel inhibitor of DNA polymerase beta. J Biochem. 1999;126(2):430–436.
- Ohtsubo T, Ikeda F. Seasonal changes of cyanogenic glycosides in Mume (Prunus mume Sieb. et Zucc.) seeds. J Jpn Soc Hortic Sci. 1994;62(4):695–700.
- Fischer E, Bergmann M. Synthese des mandelnitril-glucosids, sambunigrins und ähnlicher stoffe. Ber Dtsch Chem Ges. Berlin, Heidelberg: Springer, Berlin, Heidelberg. (Chapter7). 1917. Vol. 50. 1047–1069.
- Nakajima N, Ubukata M. Facile synthesis of cyanogen glycosides (R)-prunasin, linamarin and (S)-heterodendrin. Biosci Biotechnol Biochem. 1998;62(3):453–458.
- Rivas F, Parra A, Martinez A, et al Enzymatic glycosylation of terpenoids. Phytochem Rev. 2013;12(2):327–339.
- Lim E-K, Ashford DA, Hou B, et al Arabidopsis glycosyltransferases as biocatalysts in fermentation for regioselective synthesis of diverse quercetin glucosides. Biotechnol Bioeng. 2004;87(5):623–631.
- Chassagne D, Crouzet JC, Bayonove CL. Identification and quantification of passion fruit cyanogenic glycosides. J Agric Food Chem. 1996;44(12):3817–3820.
- Zhang Q, Chen W, Sun L, et al The genome of Prunus mume. Nat Commun. 2012;3:1318.
- Gertz EM, Yu Y-K, Agarwala R, et al Composition-based statistics and translated nucleotide searches: improving the TBLASTN module of BLAST. BMC Biol. 2006;4:41.
- Mackenzie PI, Bock KW, Burchell B, et al Nomenclature update for the mammalian UDP glycosyltransferase (UGT) gene superfamily. Pharmacogenet Genomics. 2005;15(10):677–685.
- Sánchez-Pérez R, Jørgensen K, Olsen CE, et al Bitterness in almonds. Plant Physiol. 2008;146(3):1040–1052.
- Yamaguchi T, Kuwahara Y, Asano Y. A novel cytochrome P450, CYP3201B1, is involved in (R)‐mandelonitrile biosynthesis in a cyanogenic millipede. FEBS Open Bio. 2017;7:335–347.
- Hansen KS, Kristensen C, Tattersall DB, et al The in vitro substrate regiospecificity of recombinant UGT85B1, the cyanohydrin glucosyltransferase from Sorghum bicolor. Phytochemistry. 2003;64(1):143–151.
- Kannangara R, Motawia MS, Hansen NKK, et al Characterization and expression profile of two UDP-glucosyltransferases, UGT85K4 and UGT85K5, catalyzing the last step in cyanogenic glucoside biosynthesis in cassava. Plant J. 2011;68(2):287–301.
- Nielsen KA, Tattersall DB, Jones PR, et al Metabolon formation in dhurrin biosynthesis. Phytochemistry. 2008;69(1):88–98.
- Singleton C, Howard TP, Smirnoff N. Synthetic metabolons for metabolic engineering. J Exp Bot. 2014;65(8):1947–1954.
- Wahab MF, Breitbach ZS, Armstrong DW, et al. Problems and pitfalls in the analysis of amygdalin and its epimer. J Agric Food Chem. 2015;63(40):8966–8973.
- Mao Z, Shin H-D, Chen RR. Engineering the E. coli UDP-glucose synthesis pathway for oligosaccharide synthesis. Biotechnol Prog. 2006;22(2):369–374.
- Bennett BD, Kimball EH, Gao M, et al Absolute metabolite concentrations and implied enzyme active site occupancy in Escherichia coli. Nat Chem Biol. 2009;5(8):593–599.
- Noguchi T, Shiba T. Use of Escherichia coli polyphosphate kinase for oligosaccharide synthesis. Biosci Biotechnol Biochem. 1998;62(8):1594–1596.
- Murata K, Uchida T, Kato J, et al Polyphosphate kinase: distribution, some properties and its application as an ATP regeneration system. Agric Biol Chem. 1988;52(6):1471–1477.