ABSTRACT
Terpene alcohol is widely used in perfumes and is known to possess antibacterial activity. Moreover, in its glycosylated form, it can be applied as a nonionic surfactant in food, and in the pharmaceutical, chemical, cosmetic, and detergent industries. Presently, chemical production of terpene glucosides is hampered by high costs and low yields. Here, we investigated the microbial glucosylation of nerol (cis-3,7-dimethylocta-2,6-dien-1-ol), a component of volatile oils, by Agrobacterium sp. M-12 isolated from soil. A microbial reaction using washed cells of Agrobacterium sp. M-12, 1 g/L of nerol, and 100 g/L of maltose under optimal conditions yielded 1.8 g/L of neryl-α-D-glucopyranoside after 72 h. The molar yield of neryl-α-D-glucopyranoside was 87.6%. Additionally, we report the successful transglucosylation of other monoterpene alcohols, such as geraniol, (-)-β-citronellol, and (-)-linalool, by Agrobacterium sp. M-12. Thus, microbial glucosylation has potential widespread applicability for efficient, low-cost production of glycosylated terpene alcohols.
GRAPHICAL ABSTRACT

Agrobacterium sp. M-12 exhibited a high and efficient transglucosylation activity towards terpene alcohols.
Terpene alcohol glycosides are known components of grape, tea, rose, and other plants. They are utilized mainly in perfumes [Citation1], whereas their glycosides have wider uses as flavor precursors, antimicrobials, antifungal agents, and detergents. Not surprisingly, they have attracted increasing attention and a search for efficient production methods [Citation2,Citation3].
In plants, glycosides are synthesized via phosphorylation of a sugar. The aglycone and the sugar are biosynthesized separately, and the sugar is phosphorylated by adenine triphosphate. Then, the phosphorylated sugar reacts with uridine triphosphate to produce uridine diphosphate (UDP) sugar, which is characterized by an energy-rich anhydride. Eventually, this UDP sugar is coupled with the aglycone to produce the glycoside and a free UDP. Based on these mechanisms, several approaches for UDP sugar-driven glycosylation have been developed, including those employing quercetin 3-O-rhamnoside [Citation4] and apigenin 7-O-glucoside [Citation5]. However, these techniques are hampered by low productivity, which has limited their practical use.
Glycosides are also known to be synthesized by chemosynthesis, via the Koenigs-Knorr reaction and Schmidt glycosylation. These techniques, however, require expensive reagents such as silver (I) carbonate, as well as complex, multistage protecting and deprotecting steps.
Enzymatic glycosylation (reversed hydrolysis and transglycosylation) catalyzed by glycosidases represents an attractive method to form glycosidic bonds without the need for costly cofactors, such as UDP, or detailed knowledge of chemical synthesis [Citation6,Citation7]. Preparation of glycosides by transglycosylation benefits from a short reaction time and higher yields. Some glycosides, such as hesperidin glycosides, 2-O-α-D-glucopyranosyl-L-ascorbic acid and arbutin, are already produced at an industrial scale by transglycosylation using bacterial glycosidases [Citation8–Citation10].
A few reports have described the enzymatic production of terpene alcohol glycosides by transglycosylation. Terpene glucosides have been synthesized by Escherichia coli expressing Pichia etchellsi β-glycosidases starting from terpenes, such as nerol, geraniol, and citronellol, plus cellobiose [Citation11]. However, the structure of these terpene glucosides has not been determined. Gunata et al. [Citation12] have described the glucosylation of the above terpene alcohols by β-glycosidases from Aspergillus niger, Trichoderma reesei, Candida molischiana, and almond, using cellobiose as carbohydrate donor. However, the yield of monoterpene glucoside from monoterpene was unattractively low (< 4%). The low yield occurred due to the nature of the enzyme and the accumulation of glucose in the reaction mixture following hydrolysis of cellobiose. The enzymatic transfer of the glycosyl group from cellobiose to terpene alcohols is less efficient than that of phenolic alcohols, such as benzyl alcohol and 2-phenylethyl alcohol. Donho et al. [Citation13] showed that β-galactosidase from Aspergillus oryzae exhibited glucosylation activity towards nerol, using lactose as carbohydrate donor and leading to the accumulation of neryl-β-D-galactopyranoside. However, the amount and yield of the product obtained by the enzymatic reaction was not reported.
The α-glucosidase activities of soil bacteria during the synthesis of α-D-glucopyranoside by transglucosylation have been described previously [Citation14]. We reported that Ensifer sp. M-26 could glycosylate 6-gingerol, the active ingredient in ginger, to 5-O-α-glucosylgingerol using maltose as a less costly glycosyl donor [Citation15]. While screening for microorganisms with alkyl alcohol glycosylation activities [Citation16], we discovered a strain, Agrobacterium sp. M-12, capable of glycosylating terpene alcohol.
The present study reports the synthesis of nerol glucoside via glucosylation of nerol by Agrobacterium sp. M-12 and describes some of the resulting glucoside’s characteristics. We also examine the transglucosylation activity towards other terpene alcohols, such as geraniol, (-)-β-citronellol, and (-)-linalool.
Materials and methods
Chemicals
α-Glucosidase from yeast, β-glucosidase from almond, nerol (cis-3,7-dimethylocta-2,6-dien-1-ol), and geraniol were purchased from Wako Pure Chemical Industries (Osaka, Japan). (-)-β-Citronellol was purchased from Tokyo Chemical Industry (Tokyo, Japan). (-)-Linalool was purchased from Sigma-Aldrich (St. Louis, MO, USA). Transglucosidase from A. niger and cyclodextrin glucosyl transferase (CGTase) from Bacillus macerans were supplied by Amano Enzyme (Nagoya, Japan). Other chemicals were commercially available products and were used without further purification. The glucosides of nerol and geraniol used as standards for high-performance liquid chromatography (HPLC) were prepared by microbial transglucosylation.
Glucosylation of nerol using transglucosidase and CGTase
A reaction mixture (300 µL) containing 0.15 mg nerol, 30 mg maltose, 20 mM potassium phosphate buffer (pH 7.0), and 2 units of transglucosidase or 0.03 units of CGTase were incubated at 30°C for 24 h. The enzymatic activity was determined by thin-layer chromatography (TLC) or HPLC. One unit of transglucosidase was defined as the amount of enzyme that hydrolyzed 1 µmol of maltose per minute at 30°C. One unit of CGTase was defined as the amount of enzyme that produced 1 µmol of β-cyclodextrin per minute.
Culture media and cultivation
Basal medium consisting of 30.0 g maltose, 2.0 g (NH4)2SO4, 1.0 g KH2PO4, 1.0 g K2HPO4, 0.2 g MgSO4 · 7H2O, 2.0 g yeast extract, and 2.0 g polypeptone in 1000 mL distilled water (pH 7.0), was used for a jar fermenter culture, unless otherwise stated. In the case of flask cultures, the maltose content was 50.0 g/L. For the seed culture, Agrobacterium sp. M-12 was inoculated onto 50 mL of medium in a 200-mL flask and incubated at 30°C for one day with shaking at 180 rpm. The cell suspension from the seed culture was then inoculated in 50 mL of medium in a 200-mL baffled flask and grown on a rotary shaker at 180 rpm and 30°C. The cells precultured in flasks were inoculated in a 1-L jar fermenter (Able Co., Tokyo, Japan) containing 400 mL of basal medium. There, cells were grown under controlled conditions of pH 7.0 adjusted with 2 N NaOH, 30°C, and 400 rpm. The air flow rate was 1 vvm. Bacterial growth was measured at 600 nm using an Amersham Biosciences Ultrospec 3300 Pro (GE Healthcare, Little Chalfont, UK).
Reaction conditions for Agrobacterium sp. M-12
Cells harvested from a 0.5-mL aliquot samples from the jar fermenter were washed once with 0.5 mL of 20 mM potassium phosphate buffer (pH 7.0). The washed cells were incubated with 0.5 g/L terpene alcohols such as nerol, 100 g/L maltose, and 20 mM potassium phosphate buffer (pH 7.0) in a total volume of 0.5 mL. The mixture was incubated at 30°C with vigorous shaking and then an equal part of methanol was added to the reaction solution and centrifuged at 15,000 rpm for 4 min. After that, the supernatant was analyzed by TLC and HPLC. A time course for the conversion of nerol to neryl-α-D-glucopyranoside was carried out using 8.0 mL of harvested cells in culture medium and 8.0 mL of reaction mixture composed of 20 mM potassium phosphate buffer (pH 7.0), 100 g/L maltose, and 1.0 g/L nerol. The reaction was performed at 40°C with vigorous shaking.
Analysis of transglucosylation products
The reaction solution was analyzed by TLC on silica gel 60 F254 plates of 0.2-mm thickness (Merck, Darmstadt, Germany). Development was performed with a solvent system consisting of 2:2:1 (v/v/v) 1-butanol/2-propanol/water. Separated compounds were detected by spraying 10% H2SO4-methanol on the TLC glass plate and heating at 160°C for 1–2 min. Reaction products were analyzed quantitatively by HPLC using a COSMOSIL® 5C18-AR column (5 µm, 4.6 mm × 250 mm; Nacalai Tesque, Inc., Kyoto, Japan). Conditions included a solvent:water/methanol ratio of 3/7, a flow rate of 0.5 mL/min, temperature of 40°C, and detection at 215 nm. Under these conditions, nerol and neryl-α-D-glucopyranoside were eluted at 11.3 min and 17.3 min, respectively. The amount of neryl-α-D-glucopyranoside was calculated from a standard calibration curve made using the compound isolated in this study. The product was obtained by extraction with ethyl acetate from the reaction mixture. Ethyl acetate was then distilled away from the extract layer and the crude product was dissolved in methanol. The solution was fractionated by reverse-phase HPLC using a YMC-Pack ODS-A column (10 µm, 10.0 mm × 250 mm; YMC co., LTD., Kyoto, Japan) at a solvent:water/methanol ratio of 3/7, flow rate of 1.0 mL/min, room temperature, and detection at 215 nm. Methanol present in the fraction was distilled away, and neryl-α-D-glucopyranoside contained in water was extracted with ethyl acetate. The extract layer was then dehydrated with anhydrous magnesium sulfate, and ethyl acetate was distilled. Finally, the glucoside was obtained as a colorless and transparent liquid. 13C nuclear magnetic resonance (NMR) and 1H NMR spectra of the products were recorded with a JNM-ECXII (JEOL, Tokyo, Japan) spectrometer at room temperature in CDCl3 solution and chemical shifts were recorded in δ (parts per million). 13C NMR (400 MHz, CDCl3) shifts were as follows: 140.2 (7-C), 131.5 (2-C), 123.7 (4-C), 121.3 (9-C), 97.6 (1′-C), 73.8 (3′-C), 72.5 (2′-C), 72.2 (5′-C), 70.4 (4′-C), 63.4 (6′-C), 61.3 (10-C), 31.8 (6-C), 26.4 (5-C), 24.6 (1-C), 22.4 (8-C), and 16.4 (3-C); 1H NMR (400 MHz, CDCl3) shifts were as follows: 5.37 (t, 1H; H-9), 5.09 (t, 1H; H-4), 4.78 (d, 1H; H-1′), 4.10 (dd, 2H, H-10), 3.77 (dd, 1H; H-6′a), 3.66 (dd, 1H; H-2′), 3.61 (t, 1H; H-3′), 3.54 (m, 1H; H-5′), 3.35 (dd, 1H; H-6′b), 3.29 (t, 1H; H-4′), 2.10 (t, 2H; H-5), 2.04 (t, 2H; H-6), 1.73 (s, 3H; H-8), 1.65 (s, 3H; H-3), and 1.59 (s, 3H; H-1).
Properties of neryl-α-D-glucopyranoside
To confirm the glucosidic linkage between glucose and nerol, 2.9 g/L neryl-α-D-glucopyranoside and 0.5 units of glucosidase were incubated at 30°C for 2 h. The enzymatic activity of glucosidases was determined by HPLC. One unit of α-glucosidases was defined as the amount of enzyme that hydrolyzed 1 μmol of maltose per minute at 25°C. One unit of β-glucosidases was defined as the amount of enzyme that hydrolyzed 1 μmol of ρ-nitrophenyl-β -D-glucopyranoside per minute at 37°C. To evaluate its solubility, purified neryl-α-D-glucopyranoside was diluted 10 times with water and agitated at 30°C for two days. After centrifugal separation, the supernatant was analyzed by HPLC.
Results and discussion
Glucosylation of nerol
We previously screened for microorganisms in the soil that could accumulate glucosylated nerol from nerol and maltose [Citation16]. After screening a large number of bacteria, Agrobacterium sp. M-12, which can grow on a medium containing maltose as the sole carbon source, was found to exhibit high glucosylation activity towards nerol when maltose was added to the reaction mixture. Supporting our finding, an earlier study revealed α-anomer-selective glucosyl transfer from maltose by a maltose-assimilating bacterium [Citation14]. The 16S rDNA sequence (Figure S1) revealed 99.8% identity to Agrobacteium larrymoorei strain AF3.10 (type strain). Agrobacterium sp. M-12 was deposited at the National Institute of Technology and Evaluation (NITE) under accession number NITE AP-02189.
In the present study, we compared the synthesis of nerol glycoside using transglucosidase, CGTase, or Agrobacterium sp. M-12. As shown by TLC analysis, a clear spot thought to be a transglucosylation product (indicated by an arrow in ) was detected in the reaction mixture of Agrobacterium sp. M-12. Other oligosaccharides such as malto-oligosaccharides, or other glucosylated derivatives such as nerol oligoglucosides (for example, nerol α-maltoside) were not detected in the reaction mixture, indicating that Agrobacterium sp. M-12 had strong transglucosylation activity towards nerol. Transglucosidase produced only small amounts of the product, as revealed by TLC analysis. The accumulation of glucose and oligosaccharides was considerably more pronounced in the reaction with transglucosidase than with Agrobacterium sp. M-12. Maltose was preferred over nerol as an acceptor substrate for the transglucosylation reactions. Glucosylated compounds from nerol were not observed in the reaction with CGTase.
Figure 1. Thin-layer chromatography showing reaction products obtained from nerol and maltose using CGTase (lane 1), transglucosidase (lane 2), and washed cells of Agrobacterium sp. M-12 (lane 3).
The arrow indicates the reaction product derived from nerol. Spots of lower mobility than maltose indicate malto-oligosaccharides. P, nerol glucoside; G, glucose; M, maltose.
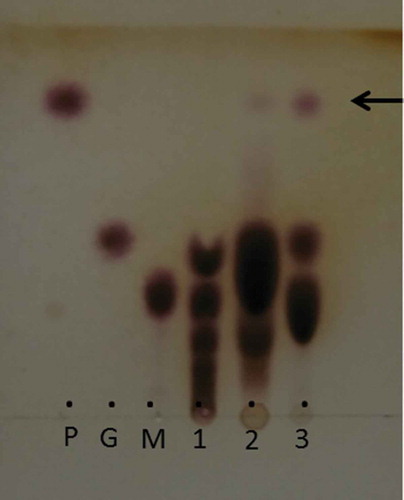
Isolation and identification of the product obtained by glucosylation of nerol by Agrobacterium sp. M-12
The product obtained by glucosylation of nerol by Agrobacterium sp. M-12 was a colorless and transparent liquid; it was identified as neryl-α-D-glucopyranoside () via 1H NMR and 13C NMR. The specific chemical shifts of the product are listed in Materials and methods. 13C NMR singlet signal at δ 97.6, which is assignable as an anomeric carbon of glucose, indicated that Agrobacterium sp. M-12 selectively produced a stereoisomer of an anomer. Furthermore, 1H NMR doublet signal at δ 4.78 was derived from an anomeric proton of glucose, its small coupling constant (J = 3.00 Hz) suggested an α-glucosyl linkage to nerol. Moreover, we confirmed the glycosidic linkage between glucose and nerol following hydrolysis by α- and β-glucosidase. In that reaction, the glucosylated nerol was hydrolyzed by α-glucosidase, with the concomitant release of glucose. In contrast, β-glucosidase did not catalyze the hydrolysis of glucosylated nerol (Figure S2).
Optimization of culture and reaction conditions for Agrobacterium sp. M-12
To obtain active cells of Agrobacterium sp. M-12 for neryl-α-D-glucopyranoside production, Agrobacterium sp. M-12 was cultured in a jar fermenter. The time course of the fermentation is illustrated in . As enzymatic activity appeared similar during growth and stationary phase, the glucosylation reaction was evaluated on cells harvested when optical density at 600 nm was nearly 5.0.
Figure 3. Time course showing growth and transglucosylation activity of Agrobacterium sp. M-12 in a 1-L jar fermenter.
Closed circles, turbidity at 600 nm; closed squares, transglucosylation activity. Values represent means of three independent experiments.
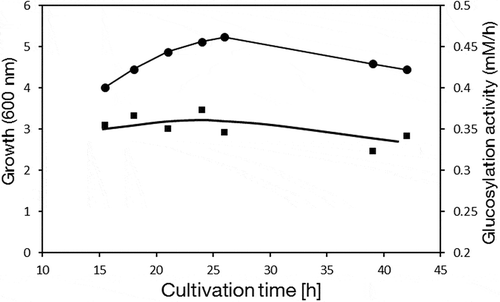
The conditions of glucosylation by Agrobacterium sp. M-12 were examined in detail, including the effect of maltose concentration and temperature. The process of nerol α-glucosylation was positively affected by increasing the starting concentration of glycosyl donor (maltose). We also confirmed that the washed cells of Agrobacterium sp. M-12 could not form neryl-α-D-glucopyranoside when glucose replaced maltose as an α-glucosyl donor. After investigating the rate of the reaction using a range of maltose concentrations up to 300 g/L, we selected 100 g/L of maltose as the optimal glycosyl donor concentration, as it resulted in the highest reaction rate (Figure S3). shows the effect of reaction temperature. At 60°C, the activity decreased rapidly and therefore optimal temperature was set to 50°C, which allowed the reaction to be completed within 6 h. Nevertheless, it should be noted that the largest amount of neryl-α-D-glucopyranoside accumulated at 40°C in 48 h. These results may be explained by a gradual loss of activity at temperatures above 50°C.
Production of neryl-α-D-glucopyranoside
shows the time course for the synthesis of neryl-α-D-glucopyranoside under optimal conditions. These allowed for 1.0 g/L of nerol to be converted to 1.8 g/L of neryl-α-D-glucopyranoside after 72 h, corresponding to a molar yield of 87.6%. Other oligosaccharides such as maltotriose and maltotetraose, or neryl maltoside were not detected. However, in the time course monitored by HPLC, other small peaks appeared in addition to those for nerol and neryl-α-D-glucopyranoside. These were subsequently identified as geraniol and geraniol glucoside (geranyl-α-D-glucopyranoside) by HPLC and NMR analyses (data not shown). Zhou et al. [Citation17] reported endogenous dehydrogenation and isomerization of geraniol into nerol in E. coli. Thus, we suspected that the formation of geraniol and the glucoside was due to endogenous isomerization of nerol to geraniol and its subsequent glucosylation in the Agrobacterium sp. M-12 reaction mixture. This hypothesis is supported by the accumulation, after a 72-h reaction period, of 0.03 g/L of geraniol and 9.3 mg/L of geraniol glucoside. According to our knowledge, neither chemical nor enzymatic preparation of neryl-α-D-glucopyranoside has been published to date. Recently, various glucosyltransferases identified in plant genomes, have been expressed in other organisms by heterologous recombination. β-D-Glucosides of terpene alcohols, such as geraniol, nerol, and citronellol, were synthesized by a microbial-based whole cell biotransformation system [Citation3]; however, the accumulation of glucosides was low. Selection of the active enzyme and construction of an efficient UDP-glucose regeneration system are necessary for any future practical applications.
Figure 5. Time course showing the conversion of nerol by Agrobacterium sp. M-12.
Cells harvested from an 8.0-mL aliquot sampled from the jar fermenter were washed once with 8.0 mL of 20 mM potassium phosphate buffer (pH 7.0). The washed cells were incubated with 1.0 g/L nerol, 100 g/L maltose, and 20 mM potassium phosphate buffer (pH 7.0) in a total volume of 8.0 mL. The reaction was carried out in a 15.0-mL conical tube at 40°C with vigorous shaking. Closed circles, nerol; open circles, neryl-α-D-glucopyranoside; open squares, geraniol.
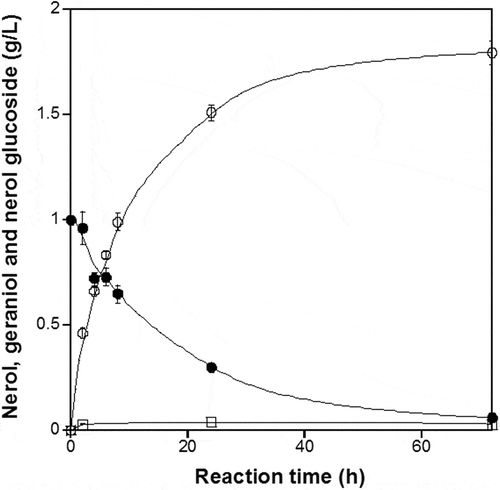
Properties of neryl-α-D-glucopyranoside
In general, glycosylation of non-sugar compounds increases their solubility; this was confirmed in the present case. The amount of neryl-α-D-glucopyranoside dissolved in water was 22.0 g/L, substantially higher than the 0.53 g/L of nerol (at 25°C) [Citation18]. Neryl-α-D-glucopyranoside had also a slightly sweeter smell compared to nerol, which explains its use in fragrances.
Glucosylation of other alcohols
Agrobacterium sp. M-12 exhibited a high and efficient transglucosylation activity towards nerol, which prompted us to investigate the glucosylation of other terpene alcohols, such as geraniol, (-)-β-citronellol, and (-)-linalool. Transglucosylation products were detected in all the reactions tested (Figure S4). When geraniol was used as substrate, nerol and neryl-α-D-glucopyranoside appeared in the reaction mixture (Figure S5), in agreement with nerol transglucosylation and the consequent accumulation of geraniol and the glucoside. (-)-Linalool, which has a sterically hindered hydroxyl group, was used as an acceptor in this reaction. It should be noted that the order of reactivity of glycosidases toward hydroxyl groups is primary alcohols > secondary alcohols > phenols; whereas tertiary alcohols are unreactive [Citation5]. Therefore, it will be interesting, in the future, to confirm the substrate specificity of transglucosylation by Agrobacterium sp. M-12.
On the basis of our recent discovery of α-anomer-selective glucosylation of terpene alcohol by an intracellular enzyme of Agrobacterium sp. M-12, which uses maltose as the α-glucosyl donor, we expect future work to focus on identification and cloning of the enzyme responsible for transglucosylation in this species. This will proceed in parallel with characterization of its acceptor specificity for terpene alcohols and related compounds, including enantioselectivity of chiral alcohols.
supplemental_ueda_revised.pdf
Download PDF (144.8 KB)Acknowledgments
We thank Lecturer Kei Nishii for performing the NMR studies and Amano Enzyme (Nagoya, Japan) for the kind gift of transglucosidase and CGTase.
Disclosure statement
No potential conflict of interest was reported by the authors.
Supplementary Material
Supplementary data for this article can be accessed here.
Additional information
Funding
References
- Inoue Y, Shiraishi A, Hada T, et al The antibacterial effects of terpene alcohols on Staphylococcus aureus and their mode of action. FEMS Microbiol Lett. 2004;237(2):325–331.
- Schwab W, Fischer T, Wüst M. Terpene glucoside production: improved biocatalytic processes using glycosyltransferases. Eng Life Sci. 2015;15(4):376–386.
- Schwab W, Fischer T, Giri A, et al Potential applications of glucosyltransferases in terpene glucoside production: impacts on the use of aroma and fragrance. Appl Microbiol Biotechnol. 2015;99(1):165–174.
- Kim B, Kim H, Ahn J. Production of bioactive flavonol rhamnosides by expression of plant genes in Escherichia coli. J Agric Food Chem. 2012;60(44):11143–11148.
- Thuan NH, Park JW, Sohng JK. Toward the production of flavone-7-O-β-D-glucopyranosides using Arabidopsis glycosyltransferase in Escherichia coli. Process Biochem. 2013;48(11):1744–1748.
- van Rantwijk F, Woudenberg-van Oosterom M, Sheldon RA. Glycosidase-catalysed synthesis of alkyl glycosides. J Mol Cat B Enzym. 1999;6:511–532.
- Divakar S, editor. Glycosidases. In: Enzymatic transformation. India: Springer; 2013. p. 5–21.
- Kometani T, Terada Y, Nishimura T, et al Transglycosylation to hesperidin by cyclodextrin glucanotransferase from an alkalophilic Bacillus species in alkaline pH and properties of hesperidin glycosides. Biosci Biotech Biochem. 1994;58(11):1990–1994.
- Aga H, Yoneyama M, Sakai S, et al Synthesis of 2-O-α-D-glucopyranosyl L-ascorbic acid by cyclomaltodextrin glucanotransferase from Bacillus stearothermophilus. Agric Biol Chem. 1991;55(7):1751–1756.
- Nishimura T, Kometani T, Takii H, et al Purification and some properties of α-amylase from Bacillus subtilis X-23 that glucosylates phenolic compounds such as hydroquinone. J Ferment Bioeng. 1994;78(1):31–36.
- Bachhawat P, Mishra S, Bhatia Y, et al Enzymatic synthesis of oligosaccharides, alkyl and terpenyl glucosides, by recombinant Escherichia coli-expressed Pichia etchellsii β-glucosidase II. Appl Microbiol Biotechnol. 2004;118(1–3):269–282.
- Gunata Z, Vallier MJ, Sapis JC, et al Enzymatic synthesis of monoterpenyl β-D-glucosides by various β-glucosidases. Enzyme Microb Technol. 1994;16(12):1055–1058.
- Donho M, Kimura T, Hara H, inventors; Unitika Ltd, assignee. Japan Kokai Tokkyo Koho188,590. 1996-188590. 1996 Jul 23.
- Sato T, Hasegawa N, Saito J, et al Purification, characterization, and gene identification of an α-glucosidase from Xanthomonas campestris WU-9701. J Mol Cat B Enzyme. 2012;80:20–27.
- Matsumoto R, Satoh H, Ueda M. Production of 5-O-α-glucosylgingerol via glucosylation of 6-gingerol by Ensifer sp. M-26. J Mol Cat B Enzym. 2016;133:S200–S203.
- Ueda M, Satoh T, inventors; Nissei Bio Co., Ltd, assignee. Japan Kokai Tokkyo Koho123,844. 2017-123844. 2017 Jul 20.
- Zhou J, Wang C, Yoon SH, et al Engineering Escherichia coli for selective geraniol production with minimized endogenous dehydrogenation. J Biotechnol. 2014;169:42–50.
- Miller DJ, Hawthorne SB. Solubility of liquid organic flavor and fragrance compounds in subcritical (hot/liquid) water from 298 K to 473 K. J Chem Eng Data. 2000;45:315–318.