ABSTRACT
Acetic acid bacteria are used in the commercial production of lactobionic acid (LacA). However, the lactose-oxidizing enzyme of these bacteria remains unidentified. Lactose-oxidizing activity has been detected in bacterial membrane fractions and is strongly inhibited by d-glucose, suggesting that the enzyme was a membrane-bound quinoprotein glucose dehydrogenase, but these dehydrogenases have been reported to be incapable of oxidizing lactose. Thus, we generated m-GDH-overexpressing and -deficient strains of Komagataeibacter medellinensis NBRC3288 and investigated their lactose-oxidizing activities. Whereas the overexpressing variants produced ~2–5-fold higher amounts of LacA than the wild-type strains, the deficient variant produced no LacA or d-gluconic acid. Our results indicate that the lactose-oxidizing enzyme from acetic acid bacteria is membrane-bound quinoprotein glucose dehydrogenase.
Abbreviations: LacA: lactobionic acid; AAB: acetic acid bacterium; m-GDH: membrane-bound quinoprotein glucose dehydrogenase; DCIP: 2,6-dichlorophenolindophenol; HPAEC-PAD: high-performance anion-exchange chromatography with pulsed amperometric detection
Graphic abstract
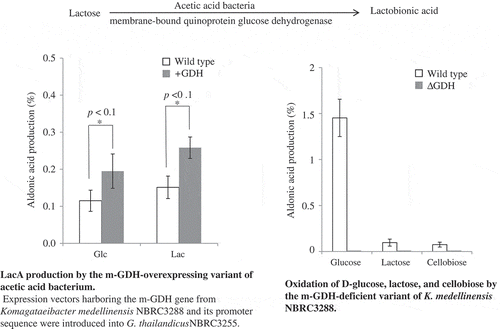
We identified the lactobionic acid-producing enzyme of acetic acid bacterium as membrane-bound quinoprotein glucose dehydrogenase.
Lactobionic acid (LacA; β-d-galactopyranosyl-(1→4)-d-gluconic acid) is an oligoaldonic acid that promotes intestinal absorption of calcium and is contained in “Caspian Sea yogurt,” which is available in Japan [Citation1,Citation2]. Thus, methods to produce LacA as a food additive have been investigated worldwide. Certain bacterial methods applied using Pseudomonas graveolens and Burkholderia cepacia are reportedly efficient for producing LacA from lactose [Citation3–Citation6], and fungal carbohydrate:acceptor oxidoreductases from Microdochium nivale and Paraconiothyrium sp. have also been found to show high lactose-oxidizing activity [Citation7,Citation8]. However, these bacterial and enzymatic methods are not employed for producing food-grade LacA because the safe use of the microorganisms involved remains incompletely ensured. We previously reported the production of LacA from the oxidation of lactose by the acetic acid bacteria (AABs) Acetobacter sp., Gluconobacter sp., and Gluconacetobacter sp. [Citation1,Citation9]. AABs are suitable for food production, and Acetobacter sp. strains are currently used for vinegar fermentation. Other investigations conducted by our group established an industrial LacA-production method by using AABs [Citation10,Citation11], and LacA produced using this method is now added in the yogurts, beverages, and dietary supplements available in Japanese supermarkets [Citation10,Citation11].
Oxidation of d-glucose by AABs is catalyzed by membrane-bound quinoprotein glucose dehydrogenase (m-GDH) [Citation12], but m-GDH reportedly cannot oxidize lactose [Citation12–Citation14]. However, in our previous study, most AAB strains (48 strains) showed lactose-oxidizing activity, although this activity was considerably lower than their d-glucose-oxidizing activity [Citation10]. The lactose-oxidizing enzyme of AABs remains unknown. Here, we investigated the properties of the lactose-oxidizing activity of AABs, and we identified the lactose-oxidizing enzyme from Komagataeibacter medellinensis (Gluconacetobacter xylinus) NBRC3288 as m-GDH.
Materials and methods
AABs
Acetobacter orientalis KYG22, which produces LacA in Caspian Sea yogurt, was isolated from yogurt [Citation1]. Gluconobacter frateurii NBRC3285 was selected as a high lactose-oxidizing AAB [Citation10]. Genome and plasmid sequences of K. medellinensis NBRC3288 are available for download from the public genome database of the Kyoto Encyclopedia of Genes and Genomes (KEGG). The plasmid (pGXY070) and m-GDH sequences of this strain were downloaded and used for preparation of the Escherichia coli-AAB shuttle vector and m-GDH-overexpressing variant, respectively. The m-GDH-deficient variant was prepared via knockout of the m-GDH gene from the genome of this strain. Gluconobacter thailandicus NBRC3255 and Gluconobacter oxydans NBRC3244 were used as host strains for m-GDH-overexpressing variants.
Preparation of resting cells
AABs were precultured in YPGL medium (pH 7.0) [Citation1] at 27°C for 2 days, with shaking at 240 oscillations/min, after which the cultures (1/100 volume) were transferred to new test tubes containing YPGL medium (pH 7.0) or SG medium (pH 7.0); SG medium was composed of 1% sorbitol, 1% glycerol, 0.3% Polypepton (Nihon Pharmaceuticals Co., Ltd., Tokyo, Japan), and yeast extract D-3 (Nihon Pharmaceuticals Co., Ltd.). After cultivation for 3 days under the same conditions as the preculture, cells were harvested from the broths, washed twice with saline solution, and resuspended in saline (1/20 volume of the broth) [Citation1]. This 20-fold-concentrated cell suspension was used as 20× resting cells.
Preparation of membrane and soluble fractions
Acetobacter orientalis KYG22 cells [Citation1] were cultivated in five shaking flasks containing 100 mL of YPGL medium. Resting cells (15 U of d-glucose-oxidizing activity, 1.1 g wet-cell weight) were prepared and resuspended in 20 mL of saline, lysed using a French press, and centrifuged to prepare a cell-free extract, as described previously [Citation15], and the extract (10 mL) was separated into membrane and soluble fractions through ultracentrifugation [Citation15]. The protein concentration in the fractions was measured using a protein assay (Bio-Rad Laboratories, Hercules, CA, USA).
Measurement of sugar-oxidizing activity of resting cells
Mixtures containing 7.5 μM 2,6-dichlorophenolindophenol (DCIP), 100 mM acetate buffer (pH 5.5), and substrate (25 mM d-glucose or 250 mM lactose) were reacted with 1/40 volume of 2×–20× concentrated cell suspension or membrane fraction at 40°C, and then the 530-nm absorbance (DCIP ε530 = 7500 M−1cm−1) was measured to calculate the DCIP-consumption rate. One unit of activity was defined as the amount of 20× concentrated cell suspension (d-glucose) or membrane fraction (d-glucose and lactose) that reduced 1 μmol of DCIP per minute by using d-glucose as a substrate.
Substrate specificities of resting cells of NBRC3288
Reaction mixtures containing NBRC3288 resting cells (0.017–0.68 U/mL), 7.5 μM DCIP, 100 mM acetate buffer (pH 5.5), and 50 mM substrate () were incubated at 40°C for 6–30 min; subsequently, the 530-nm absorbance of the reaction mixtures was measured and the DCIP-consumption rate was determined to calculate oxidizing activity.
Table 1. Sugar oxidizing activities of AABs.
Effect of D-glucose on lactose oxidation by resting cells
Resting cells of G. frateurii NBRC3285 (0.070 U/mL) and K. medellinensis NBRC3288 (0.031 U/mL) were mixed with 138 mM lactose, 0–111 mM d-glucose, and 500 mM acetate buffer (pH 5.5), and the reaction mixtures were shaken (240 oscillations/min) at 27°C for 1 h. LacA production was measured by using high-performance anion-exchange chromatography with pulsed amperometric detection (HPAEC-PAD) under conditions described previously [Citation1].
Preparation of competent cells and transformation
LB broth was purchased from Wako Pure Chemical (Tokyo, Japan). Plasmids were introduced into competent Escherichia coli DH5α cells (Toyobo Co., Ltd., Osaka, Japan), and then transformants were selected on LB plates containing 50 μg/mL ampicillin (LB-amp) or 20 μg/mL chloramphenicol.
Competent cells of AABs were prepared as follows. G. thailandicus NBRC3255, G. oxydans NBRC3244, and K. medellinensis NBRC3288 were cultured in SG medium (pH 7.0), and then the cells (A660 = 0.3) in 3 mL of the broth were harvested, washed twice with ice-cold 10% sucrose, and centrifuged; the collected cell pellets were used as electro-competent cells. Cells were mixed with 70 μL of plasmid solution (100 ng), which was introduced into the cells by using a MicroPulser electroporator (at 1.8 kV) and 1-mm electroporation cuvettes (Bio-Rad Laboratories). The transformants were selected on LB-amp plates or SG plates containing 40 μg/mL chloramphenicol (SG-cm).
Preparation of m-GDH-overexpressing AAB strains
Genomic DNA of K. medellinensis NBRC3288 was purified using a DNeasy Blood and Tissue Kit (Qiagen, Hilden, Germany). The m-GDH gene (GXL-05970; GenBank GeneID, 347759818) including the promoter sequence (−122 to 0) was amplified, using Takara EX Taq polymerase (Takara Bio, Inc., Otsu, Japan), from the genomic DNA by using the primer pair gam-GDH-(-122)-NdeI (5′-CAACCATATGCGAACTGCAAGGGTGGTTT-3′) and gam-GDH-Eco (5′-CAGAATTCTCAGTTCTGGTCGGCCAGGG-3′). This fragment was cloned in T-vector pMD20 (Takara Bio, Inc.), using a DNA Ligation Kit Ver.2.1 (Takara Bio, Inc.), and the resultant plasmids were transformed into competent E. coli DH5α cells. The transformed cells were cultivated in LB-amp medium to obtain plasmid-cloned m-GDH [pMD20-(-122)-GXL-05970]. Moreover, certain plasmids were extracted from K. medellinensis NBRC3288 for use as PCR template: pGXY070 (GenBank GeneID, 347581138) was amplified from the extracted plasmids by using PCR (Phusion Hot Start II High-Fidelity DNA Polymerase, Thermo Fisher Scientific, Inc., Waltham, MA, USA) with the primer pair pGXY070-IF-F (5′-TATGGATATCGGATCAGACGAAATTGGTGTTTTGTA-3′) and pGXY070-IF-R (5′-CGGTACCCGGGGATCGGGTGGGAGAAGGCGAAAA-3′). The amplified 2.4-kb products were ligated with BamHI-digested pMD20-(-122)-GXL-05970 by using an In-Fusion HD Cloning Kit (Takara Bio, Inc.), and the resultant plasmid, pMD20-(-122)-GXL-05970-pGXY070, was introduced into E. coli DH5α cells and the transformants were selected on LB-amp plates. pMD20-(-122)-GXL-05970-pGXY070 was purified and introduced into G. thailandicus NBRC3255 and G. oxydans NBRC3244 competent cells through electroporation, and the transformants were selected on SG-amp plates. Introduction of plasmids was verified by sequencing.
Preparation of m-GDH-knockout K. medellinensis NBRC3288
The m-GDH gene was amplified from genomic DNA by using Phusion Hot Start II Fidelity DNA Polymerase with the primer pair gaM-GDH2-Eco (5′- CAGAATTCTCAGTTCACGTTATCCAGCGC-3′) and gaM-GDH2-Xho (5′-CACTCGAGTCAGTTCTGGTCGGCCAGGG-3′). The PCR products were restriction-digested with EcoRI and XhoI. Moreover, the plasmid pUC19 (Takara Bio, Inc.) was digested with the corresponding restriction enzymes and dephosphorized using calf alkaline phosphatase (Toyobo Co., Ltd.). The digested plasmids and m-GDH gene were ligated and then introduced into DH5α cells, the transformants were selected on LB-amp medium, and the plasmid pUC19/m-GDH2 was purified. The E. coli chloramphenicol acetyltransferase gene (cat) was prepared from pETDuet plasmid through PCR performed using the primer pair cm-NheI-F (5′-AATGCTAGCGGAAGATCACTTCGCAGAATA-3′) and cm-NheI-R (5′-TAAGCTAGCAGGAACTTCATTTAAATGGCG-3′). The PCR products and pUC19/m-GDH2 plasmids were restriction-digested with NheI and then ligated to insert cat into the m-GDH gene on the plasmid. The resultant plasmid (pUC19/m-gdh2::cm) was introduced into competent K. medellinensis NBRC3288 cells through electroporation. The mixture was preincubated with shaking (120 oscillations/min) at 27°C in SG medium (1 mL) for 6 h to promote homologous recombination, and m-GDH-deficient variants were then selected from SG-cm plates. The m-GDH-deficient strain was verified by sequencing of PCR products.
Oxidation of D-glucose, lactose, and cellobiose by resting cells of transformants
The wild-type strain, m-GDH-overexpressing transformants, and m-GDH-deficient variants were cultivated in SG medium. Ampicillin (50 μg/mL) was added to the broth in the case of the m-GDH-overexpressing strains. The 20× resting-cell suspensions were appropriately diluted, 1-fold (for lactose and cellobiose) or 10-fold (for d-glucose), and mixtures containing the diluted suspensions of resting cells (1/40 volume), 100 mM acetate buffer (pH 5.5), and substrate (100 mM lactose, 100 mM cellobiose, 25 mM d-glucose) were reacted at 27°C with shaking (240 oscillations/min). After 2 h (d-glucose) or 24 h (lactose and cellobiose), d-gluconic acid, LacA, and cellobionic acid production by the AAB cells was measured using HPAEC-PAD.
Preparation of Caspian Sea yogurt
Prior to the preparation of Caspian Sea yogurt, the growth of NBRC3285, NBRC3288, KYG22, and the m-GDH-deficient variant of NBRC3288 in milk was investigated by culturing these AABs at 27°C for 24 h with shaking (240 oscillation/min)
Lactococcus lactis subsp. cremoris KYG33 [Citation1] was cocultivated in 10 mL of Lactobacilli MRS Broth (Becton, Dickinson and Company, Franklin Lakes, NJ, USA) without shaking for 2 days. Wild-type NBRC3288, the m-GDH-deficient variant of NBRC3288, A. orientalis KYG22, and G. frateurii NBRC3285 were cultivated in 5 mL of SG medium (pH 7.0) at 27°C for 2 days with shaking (240 oscillations/min). The broths containing lactobacilli (1 mL) or AABs (0.5 mL) were centrifuged to harvest the cells, which were then washed twice with 0.85% NaCl and resuspended in 100 μL (KYG33) or 500 μL (AABs) of 0.85% NaCl. The lactobacillus suspension (100 μL) was added to 10 mL of milk, and the cell suspensions (50 μL) of wild-type AAB strains or the m-GDH-deficient variant, or water (for the sample containing KYG33 only), were also added to the milk, and the yogurt seeds were fermented at 27°C. After 24 h of pre-fermentation, yogurt seeds (1 g) were transferred to a new tube containing 10 mL of milk. After fermentation at 27°C for 24 h, 10 mL of water and 2 mL of 1.0 M acetate were added to the yogurt, and the mixtures were centrifuged. Half volume of ethanol was added to the collected supernatants, and after storing at 4°C for 24 h, the mixtures were centrifuged and the amounts of LacA in the final supernatants were measured using HPAEC-PAD.
Results
Properties of sugar-oxidizing activities of AABs
Previously, we reported the lactose- and d-glucose-oxidizing activities of AABs [Citation1,Citation10]. Comparison of LacA production of 8 AAB strains with their d-glucose-oxidizing activities () revealed that the lactose- and d-glucose-oxidizing activities of the AABs were positively correlated. Moreover, when A. orientalis KYG22 was cultivated in YPGL medium and the time course of d-glucose- and lactose-oxidizing activities was measured, a similar trend was observed in the levels of these activities; both oxidizing activities peaked after 2 days of cultivation and then decreased upon longer cultivation [Citation9]. These results supported the hypothesis that m-GDH oxidized not only d-glucose but also lactose.
Figure 1. Comparison of d-glucose- and lactose-oxidizing activities of AABs.
LacA-production values are plotted against the d-glucose-oxidizing activity of AABs. The plotted values are from our previous work [Citation10]. The LacA production values of NBRC3281 and IFO3283 are 0.0003 and 0.0005%, respectively. The activities on d-glucose of NBRC3281 and IFO3283 are 0.0005 and 0.0006 U/mL, respectively.
![Figure 1. Comparison of d-glucose- and lactose-oxidizing activities of AABs.LacA-production values are plotted against the d-glucose-oxidizing activity of AABs. The plotted values are from our previous work [Citation10]. The LacA production values of NBRC3281 and IFO3283 are 0.0003 and 0.0005%, respectively. The activities on d-glucose of NBRC3281 and IFO3283 are 0.0005 and 0.0006 U/mL, respectively.](/cms/asset/f84fb023-0fb6-4a0f-a1ab-f1e02c2633e6/tbbb_a_1580136_f0001_b.gif)
Glucose-fructose oxidoreductase (GFOR) of Zymomonas mobilis is reported to possess lactose-oxidizing activity [Citation16]; GFOR oxidizes lactose by using fructose as an electron acceptor. Thus, we first disrupted A. orientalis KYG22 cells, prepared cell-free extracts through centrifugation, reacted the extracts in mixtures containing/lacking added fructose (125 mM lactose, McIlvaine buffer (pH 4.0 or 7.0), plus/minus 25 mM fructose) at 40°C for 1 h, and measured the amounts of LacA generated; our results demonstrated that fructose addition exerted no effect on LacA production (data not shown). Furthermore, we found that K. medellinensis NBRC3288 and Acetobacter pasteurianus IFO3283 showed lactose-oxidizing activity (); however, database analyses identified no gene sharing homology with the GFOR gene from Z. mobilis in the genome of either K. medellinensis NBRC3288 [Citation17] or A. pasteurianus IFO3283 [Citation18]. Next, when cell-free extracts of A. orientalis KYG22 were fractionated through ultracentrifugation and the supernatant and participate fractions were used as soluble and membrane fractions, respectively, d-glucose- and lactose-oxidizing activities were detected in the membrane fraction (8.8 and 1.2 U in 22 mg of protein, respectively), but not in the soluble fraction. These results indicated that the lactose-oxidizing enzyme was a membrane-bound enzyme like m-GDH and not a cytosolic enzyme like GFOR.
To identify the lactose-oxidizing enzyme of AABs, we selected K. medellinensis NBRC3288 for the experiments described below; this is because NBRC3288 showed high lactose-oxidizing activity and the strain’s entire genome sequence was available [Citation17]. The substrate specificities of the resting cells of NBRC3288 were investigated and compared with those of other AABs (). Similar substrate specificities were observed with NBRC3288 and KYG22: Both strains effectively oxidized d-glucose and D-allose, but their activities on d-galactose and d-mannose were much lower than those on d-glucose. Furthermore, similar to m-GDH purified from G. suboxydans IFO12528, NBRC3288, NBRC3285, and KYG22 exhibited lower activities on maltose and lactose than on d-glucose (). These results indicated that the lactose-oxidizing enzymes of NBRC3288 and KYG22 were potentially the same enzyme. Conversely, the oxidizing activity of NBRC3288 cells on cellobiose was substantially higher than that on maltose and lactose (); the reason for this high cellobiose-oxidizing activity is addressed in Discussion.
Effect of D-glucose on lactose oxidation by resting cells
If lactose oxidation is catalyzed by m-GDH, the oxidation should be inhibited by d-glucose, because d-glucose acts as a competitive substrate of the lactose-oxidizing enzyme. For this examination, A. orientalis KYG22 was found to be unsuitable because the lactose-oxidizing activity of this strain was low and a long incubation period (24 h) was required to detect LacA by using HPAEC-PAD. However, after the 24-h incubation, the added d-glucose was completely oxidized to d-gluconic acid. Next, we investigated NBRC3288 and G. frateurii NBRC3285, which showed relatively higher lactose-oxidizing activities. NBRC3288 and NBRC3285 cells were cultivated in YPGL medium, and the resting cells prepared from the cultures were incubated with lactose (138 mM) and d-glucose (0–111 mM) in 50 mM acetate buffer (pH 5.5) for 4 h. We found that LacA production by both strains was reduced by d-glucose addition (data not shown). However, the pH of the reaction mixtures was shifted to the acidic range (pH < 5.0), because the oxidation of d-glucose was considerably faster than that of lactose, and the production of d-gluconic acid is a response to the pH shift of the reaction mixture. To eliminate the pH-shift effect, we investigated reaction conditions that yielded LacA in amounts detectable through HPAEC-PAD while concurrently maintaining a suitable pH (5.0–5.5); 1-h reaction time generated detectable amounts of LacA, and 500 mM acetate buffer (pH 5.5) contributed toward maintaining a suitable pH (5.0–5.5). Under these conditions, LacA production by NBRC3288 and NBRC3285 cells was widely reduced by d-glucose addition ().
Figure 2. Effect of d-glucose addition on lactose oxidation by resting cells.
Inhibition of lactose oxidation by d-glucose was investigated. Resting cells of NBRC3285 (a) and NBRC3288 (b) were reacted with lactose (138 mM) and 0, 28, 56, or 111 mM d-glucose. After 1-h reaction, LacA production was measured using HPAEC-PAD (white bars). The pH values of the reaction mixtures were also measured in two independent experiments (squares). *: below detection limit.
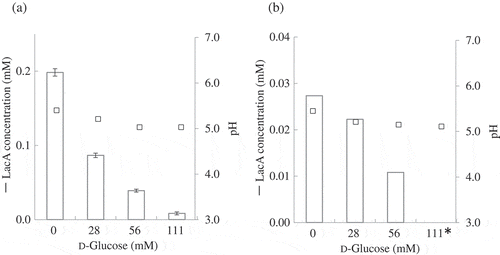
LacA production by m-GDH-overexpressing strains of AABs
Prior to this study, NADP-glucose dehydrogenase [Citation19] and m-GDH [Citation12] from AABs were reported to not exhibit lactose-oxidizing activity. However, numerous AAB strains (48 strains) were found to show lactose-oxidizing activity in our previous work [Citation10], and the properties of the lactose-oxidizing activities of the resting cells used in our studies were similar to those reported for m-GDH [Citation1,Citation10,Citation15]. Here, to identify the lactose-oxidizing enzyme of AABs as m-GDH, an E. coli-AAB shuttle vector was prepared; the m-GDH gene from NBRC3288 and its promoter sequence were ligated into a pMD20 cloning vector (pMD20-(-122)-GXL-05970), and the PCR product of pGXY070 extracted from NBRC3288 was introduced into pMD20-(-122)-GXL-05970 to generate the vector pMD20-(-122)-GXL-05970-pGXY070. The m-GDH plasmid was introduced into G. oxydans NBRC3244 and G. thailandicus NBRC3255 and the m-GDH-overexpressing transformants were obtained. The overexpressing and wild-type strains of these AABs were cultivated in SG medium; the growth (A660) of these strains after cultivation (48 h) was 1.5 (NBRC3255 wild-type), 1.5 (NBRC3255 transformant), 1.0 (NBRC3244 wild-type), and 1.1 (NBRC3244 transformant). The resting cells of the strains were prepared and LacA production was measured using HPAEC-PAD, which revealed that LacA production by the overexpressing strains of G. oxydans NBRC3244 and G. thailandicus NBRC3255 were higher than that by the corresponding wild-type strains (). Thus, m-GDH overexpression effectively increased the lactose-oxidizing activity of the AABs.
Figure 3. LacA production by m-GDH-overexpressing variant of AABs. Expression vectors harboring the m-GDH gene from K. medellinensis NBRC3288 and its promoter sequence were introduced into G. oxydans NBRC3244 (a) and G. thailandicus NBRC3255 (b).
Lactose and d-glucose were oxidized by resting cells of wild-type and mutant strains. Aldonic acid production was measured using HPAEC-PAD (n = 3–5).
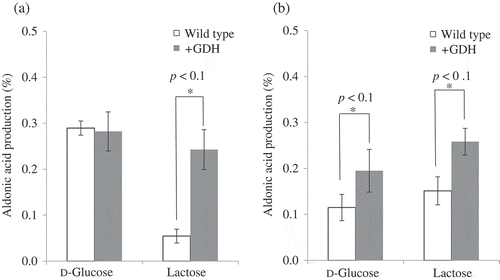
Effect of m-GDH deletion on LacA production
The m-GDH-deficient strain of K. medellinensis NBRC3288 was prepared, and the mutant and wild-type strains were cultivated in SG medium; the growth of the cells (A660) after 2 days of cultivation was 7.2 ± 1.8 (wild-type) and 8.2 ± 1.5 (m-GDH-deficient strain). When resting cells of the strains were reacted with d-glucose, lactose, and cellobiose and the production of aldonic acid was measured using HPAEC-PAD, the deficient strain was found to generate no oxidized products (); however, the wild-type strain produced d-gluconic acid, LacA, and cellobionic acid from each substrate. This result indicated that m-GDH was the lactose- and cellobiose-oxidizing enzyme of NBRC3288, and that the m-GDH-deficient variant of NBRC3288 expressed no other lactose- and cellobiose-oxidizing enzyme under these conditions.
Figure 4. Oxidation of d-glucose, lactose, and cellobiose by m-GDH-deficient variant of NBRC3288. Resting cells of NBRC3288 wild-type and m-GDH-deficient variant were prepared, and sugar-oxidizing activities were measured using HPAEC-PAD (n = 3–5).
The graph shows the production of d-gluconic acid, LacA, and cellobionic acid by the wild-type strain (white bars) and mutant strain (gray bars).
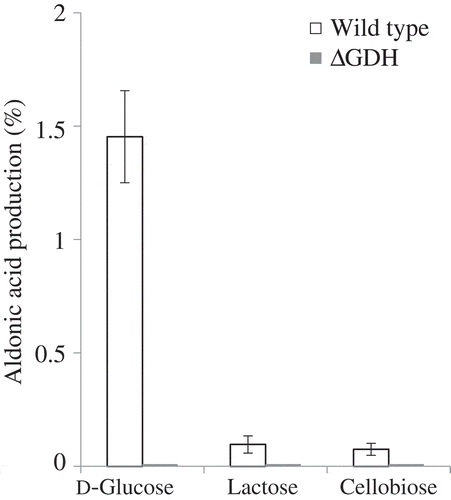
Comparison of LacA production by A. orientalis in Caspian Sea yogurt with that by other AAB strains
Before the preparation of Caspian Sea yogurt, we evaluated the growth of NBRC3285, NBRC3288, KYG22, and the m-GDH-deficient variant of NBRC3288 in milk. The growth of all of these AABs was similar (1.0–2.1 × 108 cells/mL, n = 1/strain).
Caspian Sea yogurt was prepared through co-fermentation of lactic acid bacteria and A. orientalis [Citation20]. Previously, we tested L. lactis subsp. cremoris KYG33 and A. orientalis KYG22 and found that only A. orientalis KYG22 produced LacA in the yogurt [Citation1]. The lactose-oxidizing activities of NBRC3288 and NBRC3285 were considerably higher than that of KYG22 (). Thus, to prepare lactose-rich Caspian Sea yogurt, we investigated the difference in LacA production by AABs in yogurts; the yogurts were fermented by NBRC3288, NBRC3285, and KYG22 added with KYG33. The pH values of all the yogurts were approximately 4.7 and 4.2 after 8 and 24 h of fermentation, respectively. Moreover, LacA production measured using HPAEC-PAD revealed that the yogurts fermented by NBRC3288, NBRC3285, and KYG22 contained 30, 15, and 69 mg of LacA per 100 g, respectively. Under these conditions, the relatively higher lactose-oxidizing activities of NBRC3288 and NBRC3285 did not contribute to LacA production in the yogurts.
To identify the enzyme that produced LacA in the yogurt, NBRC3288 and the m-GDH-deficient mutant of NBRC3288 were cultivated in milk together with KYG33. The pH values of the yogurts were almost the same as those mentioned above. Notably, measurement of LacA in the yogurts by using HPAEC-PAD () revealed that LacA was present in the yogurt prepared using NBRC3288 as the AAB, but that no LacA was detected in the yogurt prepared using the m-GDH-deficient strain. These results indicated that the LacA-producing enzyme in the yogurt was m-GDH.
Figure 5. HPAEC-PAD analysis of LacA production in yogurts fermented by wild-type and m-GDH-deficient variant of NBRC3288.
Yogurts were fermented by NBRC3288 wild-type strain (KYG33 + Wild) or m-GDH-deficient variant (KYG33 + ΔGDH) added with L. lactis subsp. cremoris KYG33, or by KYG33 alone (without AAB addition; KYG33). LacA in the yogurts was measured using HPAEC-PAD.
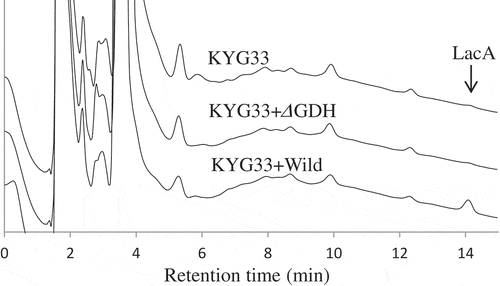
Discussion
Soluble quinoprotein glucose dehydrogenase (EC 1.1.99.17, s-GDH) from Proteobacteria reportedly exhibits high lactose-oxidizing activity [Citation21,Citation22], and the enzyme is not embedded in the membrane. Furthermore, no s-GDH was detected in AABs through immunological analysis [Citation23]. NADP-dependent d-glucose dehydrogenase (EC 1.1.1.47) has also been reported as a cytosolic sugar-oxidizing enzyme in AABs [Citation19]. However, we showed here that the soluble fraction containing the cytosolic enzyme did not oxidize lactose. Moreover, this lack of lactose-oxidizing activity in the soluble fraction indicated that the lactose-oxidizing enzyme was not cytosolic GFOR. Conversely, the quinoprotein m-GDH was reported to be present in the membrane fraction of AABs [Citation12], and m-GDH effectively oxidizes d-glucose. However, not only the m-GDH from AABs but also the m-GDH enzymes purified from Acinetobacter calcoaceticus [Citation24] and E. coli [Citation25] were reported to lack lactose-oxidizing ability. Thus, the lactose-oxidizing enzyme of A. orientalis and other AABs has remained unknown. To identify this enzyme, we investigated various properties of the lactose-oxidizing activities of AABs, and we found that several characteristics of the lactose-oxidizing enzymes of KYG22, NBRC3285, and NBRC3288 were similar to those reported for m-GDH from AABs. For example, the lactose-oxidizing enzyme of these 3 AABs strains and purified m-GDH use DCIP as an electron acceptor and show a similar pH optimum (5.0–6.0) [Citation1,Citation15]. Moreover, we found that the lactose-oxidizing enzyme from KYG22 was a membrane-bound enzyme, and further that lactose oxidation by NBRC3288 and NBRC3285 was strongly suppressed by d-glucose (), probably through competitive inhibition. Notably, the lactose-oxidizing activities of the AAB variants overexpressing the m-GDH gene from NBRC3288 were higher than those of the corresponding wild-type strains (), whereas the m-GDH-deficient strain of NBRC3288 showed no oxidizing activity against d-glucose, lactose, or cellobiose (). These results indicate that LacA and cellobionic acid were produced by m-GDH.
Both P. graveolens and B. cepacia [Citation4] produce LacA [Citation3,Citation4]. While it is known that the oxidizing enzymes of these bacteria are membrane bound [Citation3,Citation4], the enzymes themselves and their underlying genes remain unidentified. The properties of the enzyme from P. graveolens were similar to those of m-GDH. Unlike m-GDH, however, the enzyme (particulate fraction) showed relatively high lactose-oxidizing activity (about 20% of d-glucose) [Citation3]. Thus, it was difficult to determine whether this enzyme is m-GDH. If this enzyme is m-GDH, a value of 20% of d-glucose is not too high, as we found that the relative activity of NBRC3288 on lactose was about 5% of d-glucose (). In contrast, the substrate specificities of the enzyme from B. cepacia differed from those of m-GDH. Although this bacterium does not possess a soluble lactose-oxidizing enzyme like s-GDH, its relative activities on lactose and d-glucose were 100% and 107%, respectively [Citation4].
Although the m-GDHs of AABs have been reported not to oxidize lactose [Citation12], we detected lactose oxidation by AABs ( and Table 1). The substrate specificities of NBRC3288 on d-glucose and lactose were measured using 0.017 U/mL cells in a 6-min reaction and 0.68 U/mL cells in a 30 min reaction (). While a larger number of cells and longer reaction time were adopted for the lactose-oxidizing assay, reduction of DCIP was very weak. Thus, 250 mM lactose was adopted for the assay using membrane fraction of KYG22. AABs and m-GDHs effectively oxidize d-glucose. Thus the d-glucose concentration used to measure activity is usually 0.1–25 mM. For example, in the study of m-GDH from G. suboxidans, 100 µM d-glucose was used in the enzyme assay [Citation12]. A relatively lower substrate concentration probably resulted in undetectable lactose oxidation. Furthermore, a refractive index (RI) detector is usually used in high-performance liquid chromatography analysis of oxidation products. We also used an RI detector to measure LacA production by B. cepacia [Citation4]. In this previous study, the RI detector was effective because the lactose oxidation activity was sufficiently high. Although we attempted to measure minimal LacA production by resting AAB cells using an RI detector, this method was not sufficiently sensitive. Compared with that of the RI detector, the sensitivity of the PAD detector was 100–1000 times higher. This high sensitivity of the PAD detector enabled us to detect the lactose oxidation of AABs.
Our results showed that LacA production was effectively increased in m-GDH-overexpressing strains (). Unlike in the case of lactose oxidation, the amounts of d-gluconic acid produced by the NBRC3244 wild-type and m-GDH-overexpressing strains were similar (). The electrons that are produced by the oxidation of sugars are ultimately accepted by molecular oxygen through the electron transport chain, although molecular oxygen is not the direct electron acceptor in the reaction catalyzed by m-GDH [Citation26]. The oxidation of d-glucose appeared to be considerably faster than reduction of molecular oxygen in the electron transport chain, which was thus the rate-limiting step in the oxidation of d-glucose in m-GDH-overexpressing NBRC3244 cells. However, introduction of ubiquinol oxidases that participate in the electron transport chain might further accelerate d-glucose oxidation.
We have investigated the oxidation of oligosaccharides for application in industrial oligoaldonic acid production. In industrial production, the substrate is not unfailingly a single component and is occasionally a mixture of saccharides. For example, maltooligosaccharide and isomaltooligosaccharide are not purified and contain appreciable amounts of d-glucose, and the d-glucose in these oligosaccharides reduces the production of maltobionic acid and isomaltobionic acid. However, for industrial use, we have obtained these oligosaccharides from which d-glucose has been removed. Furthermore, resting cells exhibit hydrolase activity toward oligosaccharides containing d-glucose residues and substrate hydrolysis produces d-glucose in the reaction mixture, and the resulting reduction of the product by d-glucose is a serious drawback. We previously showed that NBRC3288, NBRC3285, and KYG22 did not assimilate lactose [Citation10], and our results indicated that these AABs did not hydrolyze lactose or produce d-glucose. Moreover, AABs have been reported to express β-glucosidase, which is a recognized cellobiose-hydrolyzing enzyme [Citation27], and our previous study also indicated that the resting cells of NBRC3285 can potentially hydrolyze cellobiose. Because cellobionic acid production probably negatively affects d-glucose release from cellobiose by β-glucosidase, a hydrolase-deficient variant must be generated for developing the industrial production of cellobionic acid and other gluco-oligoaldonic acids.
We found that m-GDH played a major role in lactose oxidation in Caspian Sea yogurt. The lactose-oxidizing activities of NBRC3288 and NBRC3285 were markedly higher than that of A. orientalis () [Citation10], and thus the yogurts fermented by NBRC3285 and NBRC3288 were expected to contain high amounts of LacA. However, LacA production by NBRC3288 and NBRC3285 in the yogurts was lower than that by A. orientalis. Two reasons could account for the unexpected results of LacA production in the yogurts: first, the effect of pH on lactose-oxidizing activities; and second, the proliferation of the AABs in the yogurts. The lactose-oxidizing activities shown in were measured at pH 5.5, the optimal pH, but the pH of the yogurts were shifted to <4.7 after 8 h of fermentation. Differences in m-GDH activity and/or stability at the lower pH values probably affected LacA production. Furthermore, A. orientalis has long been subcultured in Caspian Sea yogurt [Citation20], and the growth of this bacterium has adapted well to the extended subculture period necessary. However, the lactose-oxidizing activity of A. orientalis is low. Thus, further screening of AABs should enable us to obtain strains that produce higher amounts of LacA in Caspian Sea yogurt.
Author contributions
T.Kiryu and M.H. designed the study. T.Kiryu, T.Kiso, and H.M. discussed the results. T.Kiryu performed the experiments and analyzed the data. T.Kiryu wrote the manuscript with the help of all the other authors. All authors reviewed the manuscript.
Disclosure statement
No potential conflict of interest was reported by the authors.
Additional information
Funding
References
- Kiryu T, Kiso T, Nakano H, et al. Involvement of Acetobacter orientalis in the production of lactobionic acid in Caucasian yogurt (“Caspian Sea yogurt”) in Japan. J Dairy Sci. 2009;92:25–34.
- Brommage R, Binacua C, Antille S, et al. Intestinal calcium absorption in rats is stimulated by dietary lactulose and other resistant sugars. J Nutr. 1993;123:2186–2194.
- Nishizuka Y, Hayaishi O. Enzymic formation of lactobionic acid from lactose. J Biol Chem. 1962;237:2721–2728.
- Murakami H, Kawano J, Yoshizumi H, et al. Screening of lactobionic acid producing microorganisms. J Appl Glycosci. 2002;49:469–477.
- Murakami H, Seko A, Azumi M, et al. Fermentative production of lactobionic acid by Burkholderia cepacia. J Appl Glycosci. 2003;50:117–120.
- Murakami H, Seko A, Azumi M, et al. Microbial conversion of lactose to lactobionic acid by resting cells of Burkholderia cepacia No. 24. J Appl Glycosci. 2006;53:7–11.
- Xu F, Golightly EJ, Fuglsang CC, et al. A novel carbohydrate: acceptoroxidoreductase from Microdochium nivale. Eur J Biochem. 2001;268:1136–1142.
- Kiryu T, Nakano H, Kiso T, et al. Purification and characterization of a carbohydrate: acceptoroxidoreductase from Paraconiothyrium sp. that produces lactobionic acid efficiently. Biosci Biotechnol Biochem. 2008;72:833–841.
- Kiryu T, Yamauchi K, Masuyama A, et al. Optimization of lactobionic acid production by Acetobacter orientalis isolated from Caucasian fermented milk, “Caspian Sea yogurt”. Biosci Biotechnol Biochem. 2012;76:361–363.
- Kiryu T, Kiso T, Nakano H, et al. Lactobionic and cellobionic acid production profiles of the resting cells of acetic acid bacteria. Biosci Biotechnol Biochem. 2015;79:1712–1718.
- Kiryu T, Kiso T, Nakano F, et al. Development of disaccharide with aldonic acid such as lactobionic acid by resting cells and enzymes. Kagaku to Kogyo. 2013;87:55–59. Japanese
- Ameyama M, Shinagawa E, Matsushita K, et al. D-Glucose dehydrogenase of Gluconobacter suboxydans: solubilization, purification and characterization. Agric Biol Chem. 1981;45:851–861.
- De Ley J, Schell J. Oxidation of several substrates by Acetobacter aceti. J Bacteriol. 1959;77:445–451.
- King TE, Cheldelin VH. Glucose oxidation and cytochromes in solubilized particulate fractions of Acetobacter suboxydans. J Biol Chem. 1957;224:579–590.
- Kiryu T, Ooe K, Kimura T, et al. Sugar oxidation profiles of membrane-bound dehydrogenase from Acetobacter orientalis. Biocat Agric Biotech. 2012;1:262–263.
- Zachariou M, Scopes RK. Glucose-fructose oxidoreductase, a new enzyme isolated from Zymomonas mobilis that is responsible for sorbitol production. J Bacteriol. 1986;167:863–869.
- Ogino H, Azuma Y, Hosoyama A, et al. Complete genome sequence of NBRC 3288, a unique cellulose-nonproducing strain of Gluconacetobacter xylinus isolated from vinegar. J Bacteriol. 2011;193:6997–6998.
- Azuma Y, Hosoyama A, Matsutani M, et al. Whole-genome analyses reveal genetic instability of Acetobacter pasteurianus. Nucleic Acids Res. 2009;37:5768–5783.
- Avigad G, Alroy Y, Englard S. Purification and properties of a nicotinamide adenine dinucleotide phosphate-linked aldohexose dehydrogenase from Gluconobacter cerinus. J Biol Chem. 1968;243:1936–1941.
- Ishida T, Yokota A, Umezawa Y, et al. Identification and characterization of Lactococcal and Acetobacter strains isolated from traditional Caucasusian fermented milk. J Nutr Sci Vitaminol. 2005;51:187–193.
- Dokter P, Frank J, Duine JA. Purification and characterization of quinoprotein glucose dehydrogenase from Acinetobacter calcoaceticus L.M.D. 79.41. Biochem J. 1986;239:163–167.
- Southall SM, Doel JJ, Richardson DJ, et al. Soluble aldose sugar dehydrogenase from Escherichia coli: a highly exposed active site conferring broad substrate specificity. J Bio Chem. 2006;281:30650–30659.
- Matsushita K, Toyama H, Ameyama M, et al. Soluble and membrane-bound quinoprotein D-glucose dehydrogenases of the Acinetobacter calcoaceticus: the binding process of PQQ to the apoenzymes. Biosci Biotechnol Biochem. 1995;59:1548–1555.
- Matsushita K, Shinagawa E, Adachi O, et al. Quinoprotein d-glucose dehydrogenases in Acinetobacter calcoaceticus LMD 79.41: purification and characterization of the membrane-bound enzyme distinct from the soluble enzyme. Antonie Van Leeuwenhoek. 1989;56:63–72.
- Ameyama M, Nonobe M, Shinagawa E, et al. Purification and characterization of the quinoprotein d-glucose dehydrogenase apoenzyme from Escherichia coli. Agric Biol Chem. 1986;50:49–57.
- De Muynck C, Pereira CS, Naessens M, et al. The genus Gluconobacter oxydans: comprehensive overview of biochemistry and biotechnological applications. Crit Rev Biotechnol. 2007;27:147–171.
- Tonouchi N, Tahara N, Kojima Y, et al. A beta-glucosidase gene downstream of the cellulose synthase operon in cellulose-producing Acetobacter. Biosci Biotechnol Biochem. 1997;61:1789–1790.