ABSTRACT
Plant materials have been widely studied for their preventive and therapeutic effects for type 2 diabetes mellitus (T2DM) and obesity. The effect of a plant material arises from its constituents, and the study of these bioactive compounds is important to achieve a deeper understanding of its effect at the molecular level. In particular, the study of the effects of such bioactive compounds on various biological processes, from digestion to cellular responses, is required to fully understand the overall effects of plant materials in these health contexts. In this review, I summarize the bioactive compounds we have recently studied in our research group that target digestive enzymes, dipeptidyl peptidase-4, myocyte glucose uptake, and lipid accumulation in adipocytes.
Abbreviations: AC: adenylyl cyclase; AMPK: AMP-activated protein kinase; βAR: β-adrenergic receptor; CA: catecholamine; cAMP: cyclic adenosine monophosphate; cGMP: cyclic guanosine monophosphate; DPP-4: dipeptidyl peptidase-4; ERK: extracellular signal-regulated kinase; GC: guanylyl cyclase; GH: growth hormone; GLP-1: glucagon-like peptide-1; GLUT: glucose transporter; HSL: hormone-sensitive lipase; IR: insulin receptor; IRS: insulin receptor substrate; MAPK: mitogen-activated protein kinase; MEK: MAPK/ERK kinase; MG: maltase-glucoamylase; NP: natriuretic peptide; NPR: natriuretic peptide receptor; mTORC2: mechanistic target of rapamycin complex-2; PC: proanthocyanidin; PI3K: phosphoinositide 3-kinase; PKA: cAMP-dependent protein kinase; PKB (AKT): protein kinase B; PKG: cGMP-dependent protein kinase; PPARγ: peroxisome proliferator-activated receptor-γ; SGLT1: sodium-dependent glucose transporter 1; SI: sucrase-isomaltase; T2DM: type 2 diabetes mellitus; TNFα: tumor necrosis factor-α.
Graphical abstract
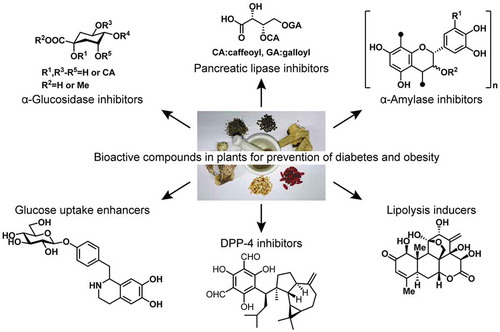
Bioactive compounds in plants show preventive effects for diabetes and obesity. The review summarizes our recent research on those compounds.
Type 2 diabetes mellitus (T2DM) is a disease characterized by hyperglycemia that results from impaired secretion of insulin and/or sensitivity to insulin, which impairs glucose metabolism. High circulating concentrations of glucose cause damage to blood vessels, leading to the development of diabetic complications, including cardiovascular disease, renal failure, blindness, and neurological disorders [Citation1]. Obesity is defined by a body mass index (BMI) above 30. The excess lipid accumulation in adipose tissue not only enlarges adipose depots, changing body appearance, but also influences the function of individual adipocytes, which increases the risk of various diseases, including T2DM, hyperlipidemia, and cardiovascular disease [Citation2]. T2DM and obesity both represent significant current global health problems. In 2017, there were estimated to be 425 million adults with diabetes (IDF Diabetes Atlas), and 650 million obese individuals in the world (WHO Fact Sheet). Accordingly, numerous studies are conducted to aid understanding of these health problems [Citation3,Citation4].
The study of novel drug substances assists the accumulation of knowledge regarding T2DM and has provided a variety of choices for the treatment of the disease. Inadequate insulin secretion can be treated using insulin injection, sulfonylureas, glucagon-like peptide-1 analogs, or dipeptidyl peptidase-4 (DPP-4) inhibitors, while insulin resistance is treated with biguanides in the first instance, and potentially also with peroxisome proliferator-activated receptor-γ (PPARγ) agonists, to improve insulin sensitivity. Inhibitors of carbohydrate digestive enzymes and glucose transporter-2 also improve glucose homeostasis by reducing the absorption of carbohydrate from the intestine and permitting the loss of glucose in urine [Citation5].
Although knowledge of the mechanisms of obesity has significantly expanded, anti-obesity medicines are less well developed. However, appetite suppressants such as lorcaserin (a serotonin 5-HT2C receptor agonist) and glucagon-like peptide-1 analogs are used, and inhibitors of lipid digestive enzymes represent an alternative [Citation6]. Although lipid metabolism is also being targeted for anti-obesity drug development, drugs with this mechanism are not yet available [Citation7]. However, despite many effective medications having been developed, the numbers of people with T2DM and/or obesity continue to rise, indicating the requirement for a wider range of modalities, not only to treat, but also to prevent these health problems arising.
Plant materials are widely used to maintain human health. Traditional medications, food supplements, and functional foods all utilize the effects of plant materials to prevent disease and/or improve health, and these include numerous substances that are believed to target T2DM and obesity. The preventive effects of plant-derived substances against T2DM and obesity can generally be explained by specific bioactive compounds contained within them. A variety of biological processes can be targeted by plant compounds, including the digestion and absorption of nutrients in the gut, the transport of absorbed nutrients to tissues, and the accumulation and metabolism of nutrients in cells [Citation8,Citation9]. However, unlike conventional medicinal compounds, plant materials may target multiple processes due to the presence of numerous compounds within them, making their effects more complex and difficult to study.
Characterization of the bioactive compounds within plant materials is essential to improve our understanding of their anti-diabetic and anti-obesity potential. In this review, I summarize the bioactive compounds that we have recently identified in plant materials, which have potential for the prevention of T2DM and/or obesity. Pancreatic lipase inhibitors (compounds 1–5) inhibit the digestion of triglycerides, which reduces the absorption of lipids from food. Intestinal α-glucosidase and pancreatic α-amylase inhibitors (6–19) inhibit the digestion of polysaccharides and delay their absorption, which can reduce post-prandial hyperglycemia. Dipeptidyl peptidase inhibitors (20–24) extend the half-life of incretin hormones, which increases insulin secretion from the pancreas, whereas glucose uptake enhancers (25 and 26) either have an additive effect to that of insulin, or stimulate additional glucose disposal into muscle cells, which can ameliorate hyperglycemia. Finally, lipolytic compounds (27 and 28) stimulate lipolysis in adipocytes, reducing the size of the intracellular lipid droplets (). Such mechanistic information aids our understanding of the effect of plant materials at the molecular level and may promote their appropriate use in the prevention of T2DM and obesity
Inhibitors of digestive enzymes
Pancreatic lipase inhibitors
Pancreatic lipase is a well-known target for prevention of obesity [Citation10]. Food-derived lipids (triacylglycerol) form an emulsion with bile acids, and are then hydrolyzed by pancreatic lipase to produce free fatty acids and monoacylglycerol, which is efficiently absorbed from the intestine. The exact absorption mechanism of these hydrolysis products remain unclear, but the inhibition of pancreatic lipase reduces the absorption of lipids, which is an effective means of preventing obesity [Citation11].
Our search for pancreatic lipase inhibitors, employing a model system using porcine pancreatic lipase as the enzyme and triolein emulsion as the substrate, resulted in the identification of hydroxychavicol (1), together with its dimers (2,3), from Eugenia polyantha [Citation12], and phenolcarboxylic acid esters of L-threonic acid (4,5) from Filipendula kamtschatica [Citation13].
E. polyantha (synonym Syzygium polyanthum) is a deciduous tropical tree, the leaves of which are aromatic, have a sour taste, and have been used as a spice in Indonesia. The inhibitory activity of 1 is moderate (half maximal inhibitory concentration [IC50] 1.0 mM), but it is present at a high concentration in the plant (1.83% w/w in dried leaves), giving it potential for use in the prevention of obesity.
F. kamtschatica is a plant that distributed from northern Japan, through the Kuril Islands, to the Kamchatka peninsula, and which is employed by the Ainu people as a traditional medicine for eczema and hives, or as an antidiarrheal agent. The yields from the isolation of 4 and 5 are 0.073% and 0.036%, and 5 shows potent inhibitory activity (IC50 26 µM). In contrast, the inhibitory activity of 4 is relatively low (IC50 246 µM), indicating the importance of the position of the caffeoyl moiety for the activity. The structures of 4 and 5 resemble that of diacylglycerol, which is an intermediate product of lipid digestion by pancreatic lipase. Therefore, substrate recognition by the lipase be at least partially responsible for the difference in inhibitory activity of the two compounds.
The inhibition of pancreatic lipase by plant components has been well studied and a variety of polyphenols, saponins, and triterpenes have been reported to have an inhibitory effect [Citation10,Citation14]. Further study of the lipase inhibitors present in plant materials will help us to understand the anti-obesity effects of such natural remedies.
Carbohydrate digestive enzyme inhibitors
When consumed, polysaccharides are initially hydrolyzed by salivary and pancreatic α-amylase to produce di- or trisaccharides, and then hydrolyzed to monosaccharides by intestinal α-glucosidase. Intestinal epithelial cells express several glucose transporters, including sodium-dependent glucose transporter 1 (SGLT1) and glucose transporter 2 (GLUT2), which efficiently transport the monosaccharides generated out of the gut lumen [Citation15]. The inhibition of α-amylase and/or α-glucosidases delays the absorption of carbohydrates by reducing the production of monosaccharides, thereby attenuating the rapid post-prandial increase in blood glucose concentration [Citation16,Citation17]. Because high post-prandial blood glucose concentrations are known to predispose toward the development of T2DM, inhibitors of α-amylase and α-glucosidases may be effective in the prevention of T2DM.
Intestinal α-glucosidase inhibitors
We have searched for α-glucosidase inhibitors in plant materials using rat intestinal α-glucosidase as a model enzyme, and identified caffeoylquinic acids (6–13) from Pluchea indica and Achillea millefolium [Citation18,Citation19]. The inhibitory activity of these compounds ranges from as low as 2.0 µM for 3,4,5-tri-O-caffeoylquinic acid methyl ester (11) to >2,000 µM for chlorogenic acid (6), depending on the position and the number of caffeoyl groups.
α-glucosidase inhibitors have been quite widely identified in plant materials, with a number of plant-derived compounds (terpenes, alkaloids, quinones, flavonoids, phenylpropanoids, hydrolysable tannins, and azasugars) having been reported to inhibit intestinal α-glucosidase [Citation17,Citation20]. Some of these show quite high potency, for example deoxynojirimycin (IC50 = 0.36 µM [Citation21]) from Morus alba (mulberry tree) and salacinol (IC50 = 6.0 µM [Citation22]) from Salacia plants. However, to understand the potential utility of intestinal α-glucosidase inhibitors in greater depth, it should be appreciated that intestinal α-glucosidase is not a single enzyme but comprises two enzyme complexes, maltase-glucoamylase (MG) and sucrase-isomaltase (SI). Although their names indicate their major substrate, SI also recognizes maltose, which is the major disaccharide produced by the digestion of starch in the intestine. Thus, the hydrolysis of maltose is performed by both MG and SI, but the predominant enzyme differs, depending on the substrate concentration. MG has a higher affinity for maltose and is the predominant hydrolase at low substrate concentration, but is inhibited by the accumulation of products. Therefore, at high concentration, SI may be more significant for the digestion of maltose [Citation23].
In most of the in vitro studies of the inhibitory activities of plant-derived compounds, a crude enzyme solution prepared from rodent intestine has been used. Therefore, The data obtained may reflect the inhibition of either MG, SI, or both. Thus, although the data give an indication of the effectiveness of the compound, to understand the properties of a candidate compound more clearly its inhibition of MG and SI should be separately assessed. One way to accomplish this is to employ recombinant α-glucosidases [Citation24], but for a more practical method we developed a single-step purification of MG from a crude enzyme solution by employing a feature of the non-competitive α-glucosidase inhibitor, 2-aminoresorcinol (14). 2-Aminoresorcinol (14) is a potent MG/SI inhibitor that was produced by the structural modification of baicalein [Citation25]. Its mode of inhibition is non-competitive, which means that it targets the enzyme-substrate complex for inhibition, forming an enzyme-substrate-inhibitor complex. This also means that in the absence of the substrate, the inhibitor does not bind to the enzyme.
By employing the characteristic mode of inhibition of 14, we designed and tested a novel method for the single-step affinity purification of the enzyme. A derivative of 14 is conjugated to sepharose gel and the crude enzyme, mixed with maltose, is passed through the gel. In the presence of maltose, the MG-maltose complex is trapped by 14 on the gel, while the other components of the solution are washed out. The trapped enzyme is then easily eluted using an elution buffer that does not contain maltose. Removal of the maltose decomposes the enzyme-substrate-inhibitor complex, such that the trapped enzyme is easily recovered, with no requirement for a change in salt concentration or pH, which can also elute non-selectively trapped proteins; or the use of an inhibitor in solution, which would have to be removed before using the purified enzyme.
The initial attempt at this method succeeded in purifying MG [Citation26]. However, several problems, including the capacity, stability of the affinity gel, and the lack of purification of SI remained. By improving the method, we should have easier access to pure MG or SI for use in evaluating the specific activity of a candidate inhibitor against each enzyme complex, and therefore should be able to gain a better understanding of the inhibitory activity of plant-derived α-glucosidase inhibitors.
Pancreatic α-amylase inhibitors
As for the inhibition of α-glucosidase, many plant extracts have been shown to inhibit pancreatic α-amylase [Citation17], including flavonoids, catechins, hydrolysable tannins, and proteins [Citation27–Citation30]. Our research group has also identified lupenone (15), a triterpene from Abrus precatorius, and corilagin (16) and macatannin B (17), elagitannins from Phyllanthus urinaria, as α-amylase inhibitors, by employing porcine pancreatic α-amylase as the model enzyme [Citation31,Citation32].
Knowledge regarding the ability of triterpenes to inhibit pancreatic α-amylase inhibition is limited. Oleanolic acid, ursolic acid, and lupeol have been reported to inhibit α-amylase [Citation33], but compared with these compounds, 15 was more potent, achieving 84% inhibition at 50 µM, in comparison to 32% for ursolic acid, and nearly no inhibition by other related triterpenes at the same concentration. When the differences in the structures of the tested triterpenes were analyzed, the lupine skeleton and C-3 ketone group seemed to be most important for the inhibitory activity [Citation31].
Several reports have suggested that α-amylase is inhibited by plant extracts containing ellagitannins, but knowledge regarding individual ellagitannins is limited to those isolated from Rubus suavissimus [Citation34]. Ellagitannins from R. suavissimus caused 15–61% inhibition of salivary α-amylase when present at 10 µg/mL, but the inhibitory activities of 16 and 17 were much lower (21% and 32%, respectively, when present at 1.0 mM), which may be due to the differences between salivary and pancreatic α-amylase. Furthermore, although several compounds isolated from plant materials have been identified to be α-amylase inhibitors, we have frequently faced difficulties in the identification process, creating doubt regarding the bioactive principal responsible for the α-amylase inhibition.
α-Amylase is an enzyme that specifically hydrolyzes polysaccharides by recognizing multiple sugar units in the substrate-binding region using “subsites” [Citation35,Citation36]. Because a single subsite recognizes a single sugar unit, it is expected that small molecules that interact with a single subsite will bind with low affinity and show poor inhibitory activity. Therefore, we speculated that the standard isolation methodology employed to identify small molecule inhibitors would not be suitable for the identification of α-amylase inhibitors in plant materials.
To confirm the importance of molecular size for the inhibition of α-amylase, an artificial α-amylase inhibitor comprising deoxynojirimycin and glucose, conjugated through an alkyl linker, was designed and synthesized. Deoxynojirimycin is a potent α-glucosidase inhibitor that does not inhibit α-amylase. However, α-amylase and α-glucosidase show similarity in their active centers [Citation37], implying that deoxynojirimycin would occupy the subsite near the active center of α-amylase, and that the reason for the lack of inhibition of α-amylase demonstrated is low affinity due to interaction with a single subsite. Addition of a glucose moiety to deoxynojirimycin should increase this affinity by permitting occupation of an additional subsite, and the conjugate should function as an α-amylase inhibitor. The molecular size of the conjugate is controlled by modifying the length of the linker. Indeed, in practice the synthetic deoxynojirimycin-glucose conjugates (18) exhibited α-amylase inhibitory activity and there was a positive correlation between the length of the linker and their inhibitory activity (). This finding implies that the size of an molecule is important for its α-amylase inhibitory efficacy [Citation38].
Figure 2. α-Amylase inhibitory activity of artificial inhibitors (18). The numbers indicate the length of the alkyl linker between deoxynojirimycin and the glucose moiety indicated by “n” in structure of compound 18 ().
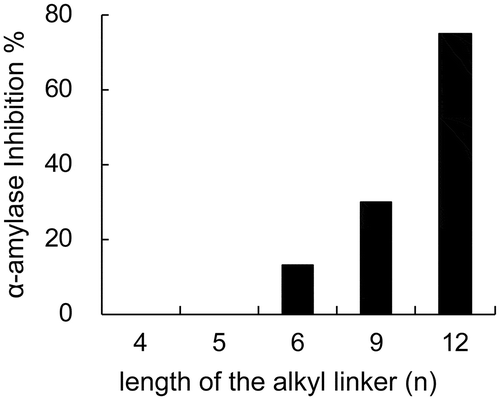
In addition to the above, we suspected a contribution of large molecules in plant materials to α-amylase inhibition. Therefore, large molecules were isolated and a highly condensed proanthocyanidin (PC) in the root of Astilbe thunbergii, named AT-P (19), was identified to be a fairly potent α-amylase inhibitor () [Citation39]. However, the identification of PC as an α-amylase inhibitor was not novel. PCs from acacia bark [Citation40], persimmon peel [Citation41], sapodilla [Citation42], and Polygonum multiflorum [Citation43] have been previously reported to inhibit α-amylase (). Moreover, PCs in apple [Citation44], grape skin [Citation45], persimmon leaf [Citation46], and almond seed skin [Citation47], have also been suggested to be the bioactive principal responsible for α-amylase inhibition, giving the strong impression that the study of PCs is important for our understanding of the α-amylase inhibitory properties of plant materials.
Table 1. α-Amylase inhibitory activity of proanthocyanidins isolated from plants.
PCs are often a mixture of condensed flavan-3-ol with different degrees of polymerization. It is difficult to determine the structure of PCs and therefore, assessment of their contribution to the α-amylase inhibition by plant materials might remain superficial. However, by separating PCs from the various plant materials and evaluating their individual α-amylase inhibitory activities, the α-amylase inhibitory properties of plant materials would be better understood.
Dipeptidyl peptidase-4 inhibitors
The secretion of insulin by pancreatic β-cells is a key step in the control of post-prandial blood glucose concentration. Pancreatic β-cells sense an elevation in blood glucose, which results in the secretion of insulin, and glucagon-like peptide-1 (GLP-1) enhances this response. GLP-1 is mainly secreted by intestinal L-cells upon stimulation by food components, but is rapidly degraded by DPP-4. Therefore, inhibitors of DPP-4 extend the half-life of GLP-1 in the circulation and are effective at ameliorating hyperglycemia and T2DM [Citation48].
The search for DPP-4 inhibitors in plant materials using human recombinant DPP-4 has resulted in the identification of rugosin A (20) (IC50 25.8 µM) and rugosin B (21) (IC50 28.5 µM), along with related hydrolyzable tannins from rose (Rosa gallica) flower [Citation49]. This research also identified macrocarpal A-C (22–24) from Eucalyptus globulus as a potent inhibitor of DPP-4 [Citation50]. The activity of 24 was characteristic, showing a rapid increase in inhibition between 30 and 35 µM, which is likely due to an aggregation of the compound in solution. Furthermore, a variety of other plant-derived compounds have been reported to be DPP-4 inhibitors, including peptides, flavonoids, resveratrol, cyanidins, and triterpenes [Citation51–Citation53].
Myocyte glucose uptake stimulators
Myocytes and adipocytes are insulin-sensitive cells that increase their uptake of glucose in response to insulin stimulation and thereby contribute to the insulin-stimulated reduction in blood glucose. Insulin resistance and insufficient insulin secretion are the two main defects in T2DM; therefore, enhancers of glucose uptake by myocytes and adipocytes can be effective for the prevention or treatment of T2DM [Citation54]. Two types of plant-derived glucose uptake enhancer have been reported, one of which is an insulin-sensitizer. This type of compound typically has its effects by activating peroxisome proliferator-activated receptor-γ (PPARγ).
PPARγ is a nuclear receptor that is highly expressed in both white and brown adipose tissue. It is a master regulator of adipogenesis and modulates lipid metabolism by enhancing the transcription of genes that contain PPAR response elements (PPRE) [Citation55]. It modulates insulin sensitivity by increasing the expression of insulin signaling pathway intermediates, including insulin receptor substrates (IRS), phosphoinositide 3-kinase (PI3K), and glucose transporter 4 (GLUT4) [Citation55]. Greater expression of these proteins enhances the response of adipocytes to insulin, including insulin-stimulated glucose uptake.
Isoflavones and flavonoids are the major plant components with PPARγ agonist activity [Citation56]. However, glycosylated isoflavones and flavonoids, which are common components of plant materials, are not reported to possess PPARγ agonist activity, with the exception of puerarin (25), the 8C-glucosylated daidzein that is found in in Pueraria iobata [Citation57]. We have studied the difference between molecules containing O- and C-linkage and evaluated the role of C-glucoside using 3T3-L1 adipocytes. A structural study revealed that O-glucosides reduce the potency of isoflavones in the enhancement of insulin induced glucose uptake into 3T3-L1 cells, but C-glucoside does not, but instead supports this activity by increasing the solubility of 25 in aqueous media [Citation58].
Another type of glucose uptake enhancer is a compound that directly stimulates the translocation of GLUT4 to the plasma membrane and enhances glucose uptake, independent of insulin. The L6 muscle cell line expresses GLUT4 and is a suitable model in which to evaluate this type of compound. Our search of plant materials for stimulators of glucose uptake resulted in the identification of higenamine 4ʹ-O-β-D-glucoside (26) from lotus (Nelumbo nucifera) plumule, a plant component used to prepare tea in one region of Japan and employed in Kampo medicine [Citation59]. The aglycon version of higenamine (norcoclaurine) exists in the leaf and embryo, but the glucoside was first form to be reported in the lotus plant. The configuration of higenamine varies between parts of the lotus plant, with the S-enantiomer being found in the leaves and the R-enantiomer in the embryo [Citation60,Citation61]. Our careful analysis of the configuration of 26, involving stereoselective synthesis of R/S-isomers, showed it to be present as 3/2 mixture of diastereomers [Citation62]. An additional study of 26 using a PI3K inhibitor (LY294002), AMPK inhibitor (dorsomorphin), and β2-adrenergic receptor antagonist (ICI118,551), all of which are specific inhibitors of proteins involved in GLUT4 translocation, revealed the β2-adrenergic receptor to be the target protein () [Citation59]. In addition, a study of the relationship between structure and activity revealed higenamine (synonym: norcoclauline) to be the core structure responsible for the activity, and showed that the S-enantiomer is more active than the R-enantiomer [Citation63].
Figure 3. The mechanism of the higher glucose uptake induced by higenamine 4ʹ-O-β-D-glucoside (26). Compound 26 activates the β2AR to increase glucose uptake. The proteins in boxes were evaluated for their involvement and the other major pathways colored gray were not implicated. β2AR: β2-adrenergic receptor, AC: adenylyl cyclase, cAMP: cyclic adenosine monophosphate, PKA: cAMP-activated protein kinase A, mTORC2: mechanistic target of rapamycin complex-2, GLUT4: glucose transporter 4, IR: insulin receptor, IRS: insulin receptor substrate, PI3K: phosphoinositide 3-kinase, PKB (AKT): protein kinase B, AMPK: AMP-activated protein kinase.
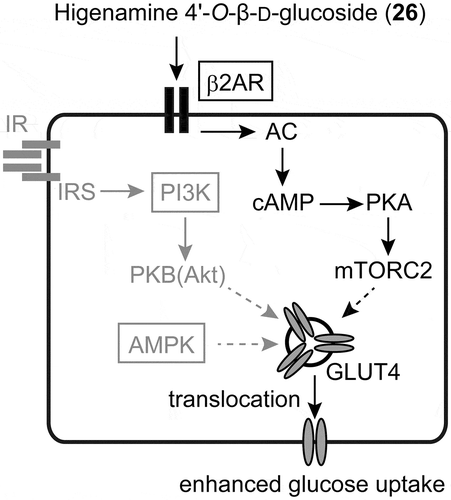
The signaling mechanism connecting β2-adrenergic receptor and GLUT4 translocation has been studied in detail and can provide an explanation for the effect of 26. Activation of the β2-adrenergic receptor activates adenylyl cyclase (AC), which generates cyclic adenosine monophosphate (cAMP), activating cAMP-dependent protein kinase (PKA). PKA phosphorylates the mechanistic target of rapamycin complex-2 (mTORC2), which stimulates the translocation of GLUT4 and enhances glucose uptake into muscle cells [Citation64].
β2-adrenergic receptor agonists are widely used as bronchodilators to treat asthma but have also been studied for their potential to treat hyperglycemia [Citation65]. In addition to 26, p-synephrine from the orange flower (Citrus aurantium) has been identified as a glucose uptake enhancer by our group, as already published by Hong et al. [Citation66]. In this article, the involvement of β2-adrenergic receptor was not confirmed, but p-synephrine is known to be a β-adrenergic receptor agonist [Citation67,Citation68], and we have also confirmed the involvement of the receptor using specific inhibitors (unpublished data). Furthermore, several other plant compounds, including osthole, gramine, and hordenine, are also β2-adrenergic receptor agonists [Citation69], suggesting these compounds are also worthy of further investigation, to develop understanding of the therapeutic effects of plant materials against diabetes.
Pro-lipolytic compounds that reduce adipocyte size
Enlargement of adipocytes through the accumulation of excess lipid is the cause of obesity, and is also associated with insulin resistance. Therefore, the control of lipid accumulation in adipocytes is likely to be beneficial in the prevention of T2DM, as well as obesity.
3T3-L1 cells are a widely accepted adipocyte cell line, and the accumulation of lipids in these cells can easily be evaluated by staining the lipid droplets with oil Red-O or Nile Red. Screening of medicinal plants for candidates that reduce lipid accumulation in 3T3-L1 adipocytes yielded Eurycoma longifolia Jack [Citation70]. Two quassinoids from this plant, eurycomanone (27) (EC50 14.6 µM) and 13β,21-epoxyeurycomanone (28) (EC50 8.6 µM), were identified as bioactive principals that enhance lipolysis, thereby reducing cellular lipid content [Citation71]. Many other plant extracts have also been reported to induce lipolysis, and several compounds have been identified as active compounds, including flavones and polymethoxyflavones [Citation72–Citation74], pterostilbene [Citation75], arylbutanoid glycosides [Citation76], aculeatin [Citation77], (3, 3-dimethylallyl)halfordinol [Citation78], and arecoline [Citation79]. However, although knowledge of plants containing pro-lipolytic compounds is gradually increasing, the exact mechanisms of action involved require clarification.
Adipocyte lipolysis is stimulated by a range of agents, including catecholamines, natriuretic peptides, TNF-α, and growth hormone [Citation80]. Catecholamines stimulate lipolysis via the β3-adrenergic receptor, which signals through AC, cAMP, and PKA to phosphorylate hormone-sensitive lipase (HSL) and perilipin [Citation80]. Natriuretic peptides act through natriuretic peptide receptors, which signal through guanylyl cyclase, cGMP, and cGMP-dependent protein kinase (PKG), which phosphorylates the same targets as PKA [Citation80]. Finally, TNF-α and growth hormone bind to their specific receptors, which results in activation of mitogen-activated protein kinase (MAPK) pathways, including extracellular signal-regulated kinase (ERK), to downregulate perilipin and enhance lipolysis () [Citation81,Citation82].
Figure 4. Lipolytic pathways and the target of eurycomanone (27) and its epoxide (28). Compounds 27 and 28 have their effect through the activation of PKA. The involvement of ERK in this activity has been ruled out, but other proteins involved in lipolysis have not been studied in detail. AC: adenylyl cyclase, βAR: β-adrenergic receptor, CA: catecholamine, cAMP: cyclic adenosine monophosphate, cGMP: cyclic guanosine monophosphate, ERK: extracellular signal-regulated kinase, GC: guanylyl cyclase, GH: growth hormone, HSL: hormone-sensitive lipase, MEK: MAPK/ERK kinase, NP: natriuretic peptide, NPR: natriuretic peptide receptor, PKA: cAMP-activated protein kinase, PKG: cGMP-activated protein kinase, TNFα: tumor necrosis factor-α.
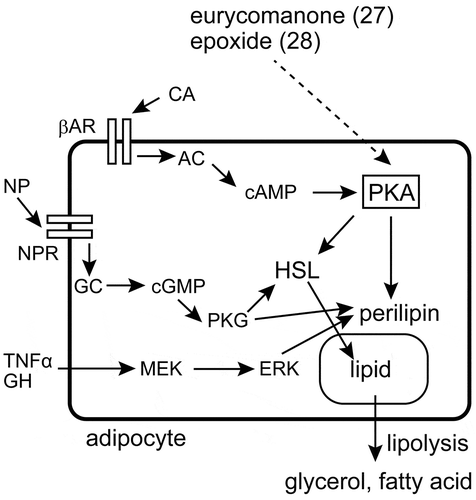
To identify the signaling pathway involved in the pro-lipolytic effect of eurycomanone (27), a PKA inhibitor (H-89) and an ERK inhibitor (PD98059) were co-incubated with this compound. PD98059 did not significantly affect the lipolytic activity of 27 and 28, but H-89 diminished their activity. The contribution of PKA to the pro-lipolytic activity of 27 and 28 was also confirmed by the greater phosphorylation of the catalytic subunit of PKA. However, activation of the β-adrenergic receptor, upstream of PKA, was prevented by co-incubation with propranolol (a non-selective β-adrenergic receptor antagonist), meaning that the precise target protein of these compounds has yet to be identified () [Citation71].
In summary, many of the pro-lipolytic compounds in plants are only known for their activity, while neither their direct targets, nor the mechanisms involved, have been studied. Therefore, a great deal of study remains to achieve understanding of the pro-lipolytic effects of the plant materials and their bioactive constituents.
Conclusion and future perspectives
Plant materials contain numerous compounds with bioactivity against a variety of biological processes related to the development of T2DM and obesity. From the efforts of many researchers, knowledge of the anti-diabetic and anti-obesity activities of plant components is accumulating. However, it is still necessary to explore and study the bioactive compounds in plant materials in greater depth, to understand their effects more clearly.
In many cases, the effects of plant materials can be explained by study of the bioactive compounds they contain, which target well-studied biological processes, such as digestion. However, there are many processes that have not been extensively studied, but may be targets of unidentified bioactive compounds in plant materials. Therefore, analysis of a wide variety of biological processes as potential targets of the bioactive constituents of plant materials is important to fully understand the effects of those materials. In addition, the exact mechanisms and target proteins of these bioactive compounds should be explored more thoroughly to improve knowledge of how these bioactive compounds have their effects at a molecular level.
Acknowledgments
The author thanks Professor Jun Kawabata and the members of the Laboratory of Food Biochemistry for their support in the studies described. The author thanks Yosuke Inagaki (Q’sai Co. Ltd., current Self Medication Japan Inc.), and Mihoko Kurokawa (Q’sai Co. Ltd.) for collaborating in part of the research described. I also thank Mark Cleasby, PhD, from Edanz Group (www.edanzediting.com/ac) for editing a draft of this manuscript.
Disclosure statement
No potential conflict of interest was reported by the author.
Additional information
Funding
References
- Chatterjee S, Khunti K, Davies MJ. Type 2 diabetes. Lancet. 2017;389:2239–2251.
- Heymsfield SB, Wadden TA. Mechanisms, pathophysiology, and management of obesity. N Engl J Med. 2017;376:254–266.
- Hameed I, Masoodi SR, Mir SA, et al. Type 2 diabetes mellitus: from a metabolic disorder to an inflammatory condition. World J Diabetes. 2015;6:598–612.
- Ashcroft FM, Rorsman P. Diabetes mellitus and the beta cell: the last ten years. Cell. 2012;148:1160–1171.
- Thrasher J. Pharmacologic management of type 2 diabetes mellitus: available therapies. Am J Med. 2017;130:S4–S17.
- MacDaniels J, Schwartz T. Effectiveness, tolerability and practical application of the newer generation anti-obesity medications. Drugs Context. 2016;5:212291.
- Joharapurkar A, Jain M, Dhanesha N. Inhibition of the methionine aminopeptidase 2 enzyme for the treatment of obesity. Diabetes, Metab Syndr Obes Targets Ther. 2014;7:73.
- Ríos J, Francini F, Schinella G. Natural products for the treatment of type 2 diabetes mellitus. Planta Med. 2015;81:975–994.
- Fu C, Jiang Y, Guo J, et al. Natural products with anti-obesity effects and different mechanisms of action. J Agric Food Chem. 2016;64:9571–9585.
- de la Garza A, Milagro F, Boque N, et al. Natural inhibitors of pancreatic lipase as new players in obesity treatment. Planta Med. 2011;77:773–785.
- Shi Y, Burn P. Lipid metabolic enzymes: emerging drug targets for the treatment of obesity. Nat Rev Drug Discov. 2004;3:695–710.
- Kato E, Nakagomi R, Gunawan-Puteri MDPT, et al. Identification of hydroxychavicol and its dimers, the lipase inhibitors contained in the Indonesian spice, Eugenia polyantha. Food Chem. 2013;136:1239–1242.
- Kato E, Yama M, Nakagomi R, et al. Substrate-like water soluble lipase inhibitors from Filipendula kamtschatica. Bioorg Med Chem Lett. 2012;22:6410–6412.
- Birari RB, Bhutani KK. Pancreatic lipase inhibitors from natural sources: unexplored potential. Drug Discov Today. 2007;12:879–889.
- Chen L, Tuo B, Dong H. Regulation of intestinal glucose absorption by ion channels and transporters. Nutrients. 2016;8:43.
- Joshi SR, Standl E, Tong N, et al. Therapeutic potential of α-glucosidase inhibitors in type 2 diabetes mellitus: an evidence-based review. Expert Opin Pharmacother. 2015;16:1959–1981.
- Tundis R, Loizzo MR, Menichini F. Natural products as alpha-amylase and alpha-glucosidase inhibitors and their hypoglycaemic potential in the treatment of diabetes: an update. Mini Rev Med Chem. 2010;10:315–331.
- Arsiningtyas IS, Gunawan-Puteri MDPT, Kato E, et al. Identification of α-glucosidase inhibitors from the leaves of Pluchea indica (L.) Less., a traditional Indonesian herb: promotion of natural product use. Nat Prod Res. 2014;28:1350–1353.
- Noda K, Kato E, Kawabata J. Intestinal α-Glucosidase Inhibitors in Achillea millefolium. Nat Prod Commun. 2017;12:1259–1261.
- Yin Z, Zhang W, Feng F, et al. α-Glucosidase inhibitors isolated from medicinal plants. Food Sci Hum Wellness. 2014;3:136–174.
- Asano N, Oseki K, Kizu H, et al. Nitrogen-in-the-ring pyranoses and furanoses: structural basis of inhibition of mammalian glycosidases. J Med Chem. 1994;37:3701–3706.
- Muraoka O, Morikawa T, Miyake S, et al. Quantitative analysis of neosalacinol and neokotalanol, another two potent α-glucosidase inhibitors from Salacia species, by LC-MS with ion pair chromatography. J Nat Med. 2011;65:142–148.
- Quezada-Calvillo R, Robayo-Torres CC, Ao Z, et al. Luminal substrate ‘brake’ on mucosal maltase-glucoamylase activity regulates total rate of starch digestion to glucose. J Pediatr Gastroenterol Nutr. 2007;45:32–43.
- Sim L, Quezada-Calvillo R, Sterchi EE, et al. Human intestinal maltase-glucoamylase: crystal structure of the N-terminal catalytic subunit and basis of inhibition and substrate specificity. J Mol Biol. 2008;375:782–792.
- Gao H, Kawabata J. 2-Aminoresorcinol is a potent α-glucosidase inhibitor. Bioorg Med Chem Lett. 2008;18:812–815.
- Kato E, Tsuji H, Kawabata J. Selective purification of intestinal maltase complex by affinity chromatography employing an uncompetitive inhibitor as the ligand. Tetrahedron. 2015;71:1419–1424.
- Lo Piparo E, Scheib H, Frei N, et al. Flavonoids for controlling starch digestion: structural requirements for inhibiting human α-amylase. J Med Chem. 2008;51:3555–3561.
- Hara Y, Honda M. The inhibition of α-amylase by tea polyphenols. Agric Biol Chem. 1990;54:1939–1945.
- Sales PM, Souza PM, Simeoni LA, et al. α-Amylase inhibitors: a review of raw material and isolated compounds from plant source. J Pharm Pharm Sci. 2012;15:141.
- Svensson B, Fukuda K, Nielsen PK, et al. Proteinaceous α-amylase inhibitors. Biochim Biophys Acta - Proteins Proteomics. 2004;1696:145–156.
- Yonemoto R, Shimada M, Gunawan-Puteri MDPT, et al. α-Amylase inhibitory triterpene from Abrus precatorius leaves. J Agric Food Chem. 2014;62:8411–8414.
- Gunawan-Puteri MDPT, Kato E, Kawabata J. α-Amylase inhibitors from an Indonesian medicinal herb, Phyllanthus urinaria. J Sci Food Agric. 2012;92:606–609.
- Ali H, Houghton PJ, Soumyanath A. α-Amylase inhibitory activity of some Malaysian plants used to treat diabetes; with particular reference to Phyllanthus amarus. J Ethnopharmacol. 2006;107:449–455.
- Li H, Tanaka T, Zhang Y-J, et al. Rubusuaviins A—F, monomeric and oligomeric ellagitannins from Chinese sweet tea and their α-amylase inhibitory activity. Chem Pharm Bull. 2007;55:1325–1331.
- Robyt JF, French D. The action pattern of porcine pancreatic α-amylase in relationship to the substrate binding site of the enzyme. J Biol Chem. 1970;245:3917–3927.
- Qian M, Haser R, Buisson G, et al. The active center of a mammalian alpha-amylase. Structure of the complex of a pancreatic alpha-amylase with a carbohydrate inhibitor refined to 2.2-A resolution. Biochemistry. 1994;33:6284–6294.
- Svensson B. Regional distant sequence homology between amylases, α-glucosidases and transglucanosylases. FEBS Lett. 1988;230:72–76.
- Kato E, Iwano N, Yamada A, et al. Synthesis and α-amylase inhibitory activity of glucose-deoxynojirimycin conjugates. Tetrahedron. 2011;67:7692–7702.
- Kato E, Kushibiki N, Inagaki Y, et al. Astilbe thunbergii reduces postprandial hyperglycemia in a type 2 diabetes rat model via pancreatic alpha-amylase inhibition by highly condensed procyanidins. Biosci Biotechnol Biochem. 2017;81:1699–1705.
- Kusano R, Ogawa S, Matsuo Y, et al. α-Amylase and lipase inhibitory activity and structural characterization of acacia bark proanthocyanidins. J Nat Prod. 2011;74:119–128.
- Lee YA, Cho EJ, Tanaka T, et al. Inhibitory activities of proanthocyanidins from persimmon against oxidative stress and digestive enzymes related to diabetes. J Nutr Sci Vitaminol (Tokyo). 2007;53:287–292.
- Wang H, Liu T, Song L, et al. Profiles and α-amylase inhibition activity of proanthocyanidins in unripe Manilkara zapota (Chiku). J Agric Food Chem. 2012;60:3098–3104.
- Wang H, Song L, Feng S, et al. Characterization of proanthocyanidins in stems of polygonum multiflorum thunb as strong starch hydrolase inhibitors. Molecules. 2013;18:2255–2265.
- Yanagida A, Kanda T, Tanabe M, et al. Inhibitory effects of apple polyphenols and related compounds on cariogenic factors of mutans streptococci. J Agric Food Chem. 2000;48:5666–5671.
- Lavelli V, Sri Harsha PSC, Ferranti P, et al. Grape skin phenolics as inhibitors of mammalian α-glucosidase and α-amylase – effect of food matrix and processing on efficacy. Food Funct. 2016;7:1655–1663.
- Kawakami K, Aketa S, Nakanami M, et al. Major water-soluble polyphenols, proanthocyanidins, in leaves of persimmon (Diospyros kaki) and their α-amylase inhibitory activity. Biosci Biotechnol Biochem. 2010;74:1380–1385.
- Tsujita T, Shintani T, Sato H. α-amylase inhibitory activity from nut seed skin polyphenols. 1. Purification and characterization of almond seed skin polyphenols. J Agric Food Chem. 2013;61:4570–4576.
- Nauck M. Incretin therapies: highlighting common features and differences in the modes of action of glucagon-like peptide-1 receptor agonists and dipeptidyl peptidase-4 inhibitors. Diabetes Obes Metab. 2016;18:203–216.
- Kato E, Uenishi Y, Inagaki Y, et al. Isolation of rugosin A, B and related compounds as dipeptidyl peptidase-IV inhibitors from rose bud extract powder. Biosci Biotechnol Biochem. 2016;80:2087–2092.
- Kato E, Kawakami K, Kawabata J. Macrocarpal C isolated from Eucalyptus globulus inhibits dipeptidyl peptidase 4 in an aggregated form. J Enzyme Inhib Med Chem. 2018;33:106–109.
- Lacroix IME, Li-Chan ECY. Food-derived dipeptidyl-peptidase IV inhibitors as a potential approach for glycemic regulation - Current knowledge and future research considerations. Trends Food Sci Technol. 2016;54:1–16.
- Kozuka M, Yamane T, Nakano Y, et al. Identification and characterization of a dipeptidyl peptidase IV inhibitor from aronia juice. Biochem Biophys Res Commun. 2015;465:433–436.
- Saleem S, Jafri L, Haq IU, et al. Plants Fagonia cretica L. and Hedera nepalensis K. Koch contain natural compounds with potent dipeptidyl peptidase-4 (DPP-4) inhibitory activity. J Ethnopharmacol. 2014;156:26–32.
- Upadhyay J, Polyzos SA, Perakakis N, et al. Pharmacotherapy of type 2 diabetes: an update. Metabolism. 2018;78:13–42.
- Ahmadian M, Suh JM, Hah N, et al. PPARγ signaling and metabolism: the good, the bad and the future. Nat Med. 2013;99:557–566.
- Wang L, Waltenberger B, Pferschy-Wenzig E-M, et al. Natural product agonists of peroxisome proliferator-activated receptor gamma (PPARγ): a review. Biochem Pharmacol. 2014;92:73–89.
- Hsu F-L, Liu I-M, Kuo D-H, et al. Antihyperglycemic effect of puerarin in streptozotocin-induced diabetic rats. J Nat Prod. 2003;66:788–792.
- Kato E, Kawabata J. Glucose uptake enhancing activity of puerarin and the role of C-glucoside suggested from activity of related compounds. Bioorg Med Chem Lett. 2010;20:4333–4336.
- Kato E, Inagaki Y, Kawabata J. Higenamine 4′-O-β-D-glucoside in the lotus plumule induces glucose uptake of L6 cells through β2-adrenergic receptor. Bioorg Med Chem. 2015;23:3317–3321.
- Koshiyama H, Ohkuma H, Kawaguchi H, et al. Isolation of 1-(p-Hydroxybenzyl)-6, 7-dihydroxy-1, 2, 3, 4-tetrahydroisoquinoline (demethylcoclaurine), an active alkaloid from Nelumbo nucifera. Chem Pharm Bull. 1970;18:2564–2568.
- Kashiwada Y, Aoshima A, Ikeshiro Y, et al. Anti-HIV benzylisoquinoline alkaloids and flavonoids from the leaves of Nelumbo nucifera, and structure-activity correlations with related alkaloids. Bioorg Med Chem. 2005;13:443–448.
- Kato E, Iwata R, Kawabata J. Synthesis and detailed examination of spectral properties of (S) and (R)-Higenamine 4ʹ-O-β-D-Glucoside and HPLC analytical conditions to distinguish the diastereomers. Molecules. 2017;22:1450.
- Kato E, Kimura S, Kawabata J. Ability of higenamine and related compounds to enhance glucose uptake in L6 cells. Bioorg Med Chem. 2017;25:6412–6416.
- Sato M, Dehvari N, Öberg AI, et al. Improving type 2 diabetes through a distinct adrenergic signaling pathway involving mTORC2 that mediates glucose uptake in skeletal muscle. Diabetes. 2014;63:4115–4129.
- Mukaida S, Evans BA, Bengtsson T, et al. Adrenoceptors promote glucose uptake into adipocytes and muscle by an insulin-independent signaling pathway involving mechanistic target of rapamycin complex 2. Pharmacol Res. 2017;116:87–92.
- Hong NY, Cui ZG, Kang HK, et al. P-Synephrine stimulates glucose consumption via AMPK in L6 skeletal muscle cells. Biochem Biophys Res Commun. 2012;418:720–724.
- Jordan R, Midgley JM, Thonoor CM, et al. β-Adrenergic activities of octopamine and synephrine stereoisomers on guinea-pig atria and trachea. J Pharm Pharmacol. 1987;39:752–754.
- Haaz S, Fontaine KR, Cutter G, et al. Citrus aurantium and synephrine alkaloids in the treatment of overweight and obesity: an update. Obes Rev. 2006;7:79–88.
- Chikazawa M, Sato R. Identification of functional food factors as β2-Adrenergic receptor agonists and their potential roles in skeletal muscle. J Nutr Sci Vitaminol (Tokyo). 2018;64:68–74.
- Lahrita L, Kato E, Kawabata J. Uncovering potential of Indonesian medicinal plants on glucose uptake enhancement and lipid suppression in 3T3-L1 adipocytes. J Ethnopharmacol. 2015;168:229–236.
- Lahrita L, Hirosawa R, Kato E, et al. Isolation and lipolytic activity of eurycomanone and its epoxy derivative from Eurycoma longifolia. Bioorg Med Chem. 2017. DOI:10.1016/j.bmc.2017.07.032
- Wang Q, Wang S, Yang X, et al. Myricetin suppresses differentiation of 3 T3-L1 preadipocytes and enhances lipolysis in adipocytes. Nutr Res. 2015;35:317–327.
- Kang S, Shin H, Kim S. Sinensetin enhances adipogenesis and lipolysis by increasing cyclic adenosine monophosphate levels in 3T3-L1 adipocytes. Biol Pharm Bull. 2015;38:552–558.
- Saito T, Abe D, Sekiya K. Nobiletin enhances differentiation and lipolysis of 3T3-L1 adipocytes. Biochem Biophys Res Commun. 2007;357:371–376.
- Gomez-Zorita S, Belles C, Briot A, et al. Pterostilbene inhibits lipogenic activity similar to resveratrol or caffeine but differently modulates lipolysis in adipocytes. Phyther Res. 2017;31:1273–1282.
- Huh JY, Lee S, Ma E-B, et al. The effects of phenolic glycosides from Betula platyphylla var. japonica on adipocyte differentiation and mature adipocyte metabolism. J Enzyme Inhib Med Chem. 2018;33:1167–1173.
- Watanabe A, Kato T, Ito Y, et al. Aculeatin, a coumarin derived from Toddalia asiatica (L.) Lam., enhances differentiation and lipolysis of 3T3-L1 adipocytes. Biochem Biophys Res Commun. 2014;453:787–792.
- Saravanan M, Pandikumar P, Saravanan S, et al. Lipolytic and antiadipogenic effects of (3,3-dimethylallyl) halfordinol on 3T3-L1 adipocytes and high fat and fructose diet induced obese C57/BL6J mice. Eur J Pharmacol. 2014;740:714–721.
- Hsu H-F, Tsou T-C, Chao H-R, et al. Effects of arecoline on adipogenesis, lipolysis, and glucose uptake of adipocytes—A possible role of betel-quid chewing in metabolic syndrome. Toxicol Appl Pharmacol. 2010;245:370–377.
- Frühbeck G, Méndez-Giménez L, Fernández-Formoso J-A, et al. Regulation of adipocyte lipolysis. Nutr Res Rev. 2014;27:63–93.
- Souza SC, Palmer HJ, Kang Y-H, et al. TNF-alpha induction of lipolysis is mediated through activation of the extracellular signal related kinase pathway in 3T3-L1 adipocytes. J Cell Biochem. 2003;89:1077–1086.
- Bergan-Roller HE, Sheridan MA. The growth hormone signaling system: insights into coordinating the anabolic and catabolic actions of growth hormone. Gen Comp Endocrinol. 2018;258:119–133.