ABSTRACT
Members of the casein kinase 1 (CK1) family are key regulators in numerous cellular signal transduction pathways and in order to prevent the development of certain diseases, CK1 kinase activity needs to be tightly regulated. Modulation of kinase activity by site-specific phosphorylation within the C-terminal regulatory domain of CK1δ has already been shown for several cellular kinases. By using biochemical methods, we now identified residues T161, T174, T176, and S181 within the kinase domain of CK1δ as target sites for checkpoint kinase 1 (Chk1). At least residues T176 and S181 show full conservation among CK1δ orthologues from different eukaryotic species. Enzyme kinetic analysis furthermore led to the hypothesis that site-specific phosphorylation within the kinase domain finally contributes to fine-tuning of CK1δ kinase activity. These data provide a basis for the extension of our knowledge about the role of site-specific phosphorylation for regulation of CK1δ and associated signal transduction pathways.
Graphical abstract
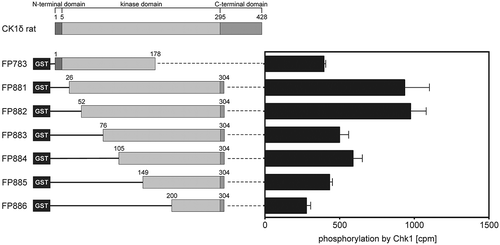
The kinase domain of CK1δ is phosphorylated by Chk1.
The family of protein kinases represents one of the largest enzyme families known for mediating reversible phosphorylation events, allowing the regulation of almost all essential cellular processes. Consequently, deregulation of protein kinases can lead to abnormal protein phosphorylation, finally resulting in deregulation of various processes and development of various diseases [Citation1,Citation2]. Casein kinase 1 (CK1) isoforms form a distinct family within the superfamily of serine/threonine-specific kinases. So far, six human isoforms (CK1α, γ1, γ2, γ3, δ, and ε) and their splice variants have been characterized. While all isoforms are highly conserved within the kinase domain, the lengths and sequences of their N- and C-terminal regulatory domains differ significantly. Members of the CK1 family act as central components in various cellular signal transduction networks and are able to regulate processes like circadian rhythm, DNA damage response, proliferation, differentiation, or apoptosis (reviewed in [Citation3]). Consequently, the expression and activity of CK1 isoforms need to be tightly regulated in order to prevent dysregulation. In this regard, it has been shown that CK1 activity and its interaction with certain substrate subsets can be regulated by subcellular compartmentation [Citation4–Citation14]. Furthermore, probably the most important mechanism is the regulation of CK1 kinase activity at the protein level mediated by posttranslational modifications including site-specific phosphorylation. Inhibitory autophosphorylation has been described for several CK1 isoforms and mainly takes place within the C-terminal regulatory domain [Citation15–Citation19], but also in the kinase domain [Citation20]. Apart from autophosphorylation, phosphorylation, and dephosphorylation of CK1 by cellular kinases or phosphatases, respectively, play an important role in regulating CK1 kinase activity. So far, site-specific phosphorylation of CK1δ by protein kinase A (PKA), protein kinase C α (PKCα), protein kinase B (Akt/PKB), CDC-like kinase 2 (CLK2), checkpoint kinase 1 (Chk1), cyclin-dependent kinase 2/cyclin E (CDK2/E), and cyclin-dependent kinase 5/p35 (CDK5/p35) has been reported, in many cases resulting in a remarkable regulatory effect on CK1δ activity [Citation21–Citation25].
In the context of DNA damage-associated signal transduction, p53-mediated signal transduction is of particular interest. The DNA damage-activated kinases ATR and ATM phosphorylate and activate Chk1 kinase and the p53 tumor suppressor, respectively [Citation26–Citation28]. In addition, CK1 isoforms α, δ, and ε site-specifically phosphorylate p53, thereby promoting its activation [Citation29–Citation33]. Forming an autoregulatory feedback loop, activated p53, in turn, can induce the expression of CK1δ [Citation32]. Activated Chk1 is also able to directly exercise regulatory effects on CK1δ. The kinase activity of CK1δ can be downregulated by site-specific phosphorylation by Chk1, thereby contributing to the fine-tuning of CK1δ activity after initiation of DNA damage-associated signal transduction [Citation21]. Recently, therapeutic strategies targeting CK1-p53-dependent pathways have also attracted attention for the treatment of hematological malignancies and hepatitis C virus infection [Citation34–Citation36].
Since Chk1-targeted phosphorylation sites in the C-terminal domain of CK1δ have previously been identified [Citation21], we now aimed to identify further phosphorylation sites within the kinase domain of CK1δ that can be phosphorylated by Chk1. By performing in vitro biochemical analysis several Chk1-targeted sites within the kinase domain of CK1δ were identified. By analyzing phosphorylation site-associated effects on CK1δ kinase activity, we furthermore provide information on possible regulatory interactions in order to deepen the understanding of the role of site-specific phosphorylation for CK1δ.
Materials and methods
Construction of expression vectors
CK1δ kinase domain fragments were amplified from the rat CK1δ reference sequence (NCBI accession ID: L07578; see [Citation18]) by PCR as indicated in , using the primer sequences given in . PCR products were ligated in pSC vector (Agilent Technologies) before finally being subcloned into pGEX vectors (GE Healthcare) to allow their expression as GST (glutathione S-transferase) fusion proteins. Mutations in fragments from CK1δ kinase domain were induced using mismatch-primers. Mutations in full-length human CK1δ transcription variant 1 (NCBI accession ID: NM_001893.4 [Citation37];) were introduced by site-directed mutagenesis (SDM; QuikChange II, Agilent Technologies) according to the manufacturer’s instructions. Sequences of primers used for PCR and site-directed mutagenesis are provided in . Sequence integrity of generated cloning and expression vectors was confirmed by DNA sequencing (Eurofins Genomics).
Table 1. Overview of the generation and nomenclature of all wild type or mutant CK1δ kinase domain fragments and full-length CK1δ fusion proteins.
Table 2. Sequences of primers used for amplification of CK1δ fragments and site-directed mutagenesis.
Expression and purification of GST fusion proteins
Fusion protein expression was induced by adding 0.5 mM IPTG to dilutions of overnight bacteria cultures (1:9) which were grown to a final OD of 0.7–0.9. After 2 h at 37°C (CK1δ protein fragments and other substrate proteins) or 18 h at 15°C (active kinase proteins) bacteria cultures were harvested by centrifugation. Lysis was performed by using lysozyme in bacteria lysis buffer (20 mM Tris-HCl [pH 7.6], 150 mM NaCl, 10% [v/v] glycerol, 0.5% [v/v] NP40, 2.5 mM DTT, 2.5 mM EDTA, 0.8 mM EGTA, 50 µM benzamidine, 25 µg/mL aprotinin) on ice for 30 min. Following fractionation of bacterial DNA by ultrasonic treatment, the lysate was cleared by centrifugation. Glutathione sepharose (50% [v/v] in PBS) was added to bind GST fusion proteins while rotating the lysate at 4°C for 2 h. Proteins bound to glutathione sepharose were washed three times with lysis buffer containing 300 mM NaCl and twice with washing buffer (20 mM Tris-HCl [pH 7.6], 50 mM NaCl, 10% [v/v] glycerol, 1 mM EDTA, 25 µg/mL aprotinin). Fusion proteins were eluted from the sepharose beads by incubation with elution solution (50 mM Tris-HCl [pH 7.0], 0.1% [w/v] reduced glutathione, 1 mM EDTA, 25 µg/mL aprotinin). High concentrations of reduced glutathione were subsequently removed by dialysis for a maximum of 2 h to ensure enzymatic activity, where appropriate. Finally, proteins were snap frozen in liquid nitrogen and stored at −80°C. In order to maintain stability, proteins were frozen as aliquots undergoing not more than three freeze-thaw cycles.
In vitro kinase reactions
In vitro phosphorylation reactions were performed in kinase buffer (25 mM Tris-HCl [pH 7.5], 10 mM MgCl2, 0.1 mM EDTA, and 10 µM ATP) containing 2 µCi of [γ-32P]-ATP. Various CK1δ kinase domain fragments were used as substrates (0.5 µg/reaction) and recombinant Chk1 (22 ng/reaction; Invitrogen) as enzyme. Kinase reactions were incubated at 30°C for 30 min and stopped by addition of SDS loading buffer. All experiments were performed in triplicate. After separation of phosphorylated proteins by SDS-PAGE, dried Coomassie-stained gels were exposed on X-ray films. Incorporation of 32P in the substrate was quantified by Cherenkov counting.
For determination of kinetic parameters, full-length GST-CK1δ wild-type and mutants were used as enzymes (2 ng/reaction), and α-casein as substrate in different concentrations (0.43, 1.74, 2.43, 3.47, 6.94, 9.72, 13.89, 19.44, and 27.78 µM). Evaluation of data from Cherenkov counting and calculation of kinetic parameters were performed using Prism 6 (GraphPad).
Kinase reactions with pre-incubation steps were performed by incubating CK1δΔC (CK1δ1−317, 35 ng/reaction; New England Biolabs) with Chk1 (22 ng/reaction; Invitrogen) in kinase buffer for 15 min at 30°C. Then, 2 µCi of [γ-32P]-ATP as well as 1 µg of α-casein were added to each reaction. After another incubation for 30 min at 30°C reactions were stopped and processed as described above.
Mass spectrometry
Fusion protein GST-CK1δ52−304 (FP882) was phosphorylated by Chk1 in vitro and subsequently separated on a NuPAGE Bis-Tris 4–12% gradient gel (Invitrogen). Coomassie-stained bands were pooled and digested in-gel with trypsin. LC-MS/MS was directly carried out with 10% of the digested sample while the left 90% peptide mixture was diluted with acetonitrile and the pH was adjusted to 2–3. Following dilution, titanium dioxide chromatography was used to enrich phosphopeptides. Thereafter, phosphopeptide elution from the beads was performed three times with 40% ammonium hydroxide solution (100 µL) in 60% acetonitrile at a pH of >10.5. Eluted peptides were analyzed on a Proxeon Easy-LC system (Proxeon Biosystems) coupled to a LTQ-Orbitrap-XL (Thermo Fisher Scientific) equipped with a nanoelectrospray ion source (Proxeon Biosystems). The five most intense precursor ions were fragmented by neutral loss ions activation at −32.6, −49, and −98 relative to the precursor ion.
Suite MaxQuant (version 1.014.3) software was used to analyze mass spectra, which were checked against a target-decoy E. coli database containing 4163 forward protein sequences. Trypsin was set as protease, and two missed cleavage sites were allowed. In addition, N-terminal acetylation, oxidation of methionine, and phosphorylation of serine, threonine, and tyrosine were set as variable modifications, while carbamidomethylation of cysteine was set as a fixed modification. Initial precursor mass tolerance at the precursor ion and the fragment ion level was set to 7 ppm (parts per million) and 0.5 Da. Modified peptide spectra were manually validated.
Two-dimensional phosphopeptide analysis
In vitro kinase reactions were performed as described above, using GST-CK1δ kinase domain fragments as substrates for Chk1. Reactions were separated by SDS-PAGE and blotted onto a PVDF membrane. Following autoradiography bands containing phosphorylated GST-CK1δ fusion proteins were excised from the membrane. Membrane pieces were incubated in 100 mM acetic acid with 0.5% [w/v] polyvinylpyrrolidone at 37°C for 30 min. After washing in ddH2O and 50 mM NH4HCO3 membrane-bound proteins were digested in a mixture of 50 mM NH4HCO3, 0.1 µg/µL TPCK-trypsin, and 0.1 µg/µL BSA at 30°C for 4 h. Digestion at 30°C was continued for another 90 min after the addition of another 0.1 µg/µL TPCK-trypsin. The samples were vacuum-dried, and the resulting pellets were oxidized in oxidation solution (10% H2O2 [v/v] in formic acid) for 2 h at room temperature in order to cleave disulfide bonds. Oxidation was stopped by the addition of ddH2O and afterward, samples were vacuum-dried. Peptides were loaded on TLC plates and electrophoresis was performed in pH 1.9 buffer (6% formic acid [v/v], 1.2% acetic acid [v/v], 0.25% pyridine [v/v]) at 1.5 kV for 30 min. Finally, in a second dimension, chromatography was performed in chromatography buffer (37.5% n-butanol [v/v], 7.5% acetic acid [v/v], 25% pyridine [v/v]) for 16 h. Thereafter, autoradiographs were created to visualize radioactively labeled phosphopeptides.
Bioinformatics
Sequences of human CK1: CSNK1A1 (P48729), CSNK1A1L (Q8N752), CSNK1G1 (Q9HCP0), CSNK1G3 (Q9Y6M4), CSNK1G2 (P78368), CSNK1D (P48730), CSNK1E (P49674); Saccharomyces cerevisiae CK1: HRR25 (P29295), YCK1 (P23291), YCK2 (P23292), YCK3 (P39962); Schizosaccharomyces pombe CK1: cki1 (P40233), cki2 (P40234), cki3 (O74135), hhp1 (P40235), hhp2 (P40236); Plasmodium falciparum CK1: PfCK1 (C6S3F7); Toxoplasma gondii CK1: TgCK1 (Q6QNM1); Trypanosoma brucei CK1: TbCK1.1 (B9VVJ7), TbCK1.2 (B9VVJ6); Trypanosoma cruzi CK1: TcCK1.1 (Q9NDD0), TcCK1.2 (Q9U8F8); Leishmania major CK1: LmjCK1.1 (Q9NHE2), LmjCK1.2 (Q9NHE1), LmjCK1.3 (O96999), LmjCK1.4 (E9ADF5), LmjCK1.5 (Q4Q9T3), LmjCK1.6 (Q4Q6U1) were obtained from Uniprot [Citation38]. Multiple sequence alignments were performed using the M-Coffee mode of the T-Coffee program [Citation39]. The resulting alignments were visualized using ClustalW alignment editor.
Statistical analysis
Results are presented as the mean of experiments at least performed in triplicate ± standard deviation. Statistical differences of enzyme kinetic data were calculated using GraphPad Prism 6 (GraphPad Software, USA) and the implemented extra sum-of-squares F-test. Statistical significance was furthermore tested by unpaired student’s t-test, calculated using Microsoft Excel (Microsoft, USA). P values <0.05 (shown as * for p < 0.05, ** for p < 0.01, or *** for p < 0.001) were considered to be statistically significant.
Results
Chk1 phosphorylates the kinase domain of CK1δ in vitro
In order to investigate the ability of Chk1 to phosphorylate amino acid residues within the kinase domain of CK1δ, different GST-CK1δ fusion proteins were used as substrates for recombinant human Chk1. Each of these fusion proteins contains different, but overlapping parts of the kinase domain of CK1δ, encompassing amino acids 5 to 295 of CK1δ [Citation3]. GST-CK1δ26−304 (FP881) and GST-CK1δ52−304 (FP882) showed the highest degree of phosphate incorporation (). According to a published consensus motif for Chk1, seven serine or threonine residues of CK1δ display at least the minimal consensus sequence for recognition by Chk1 (Arg or Lys at position −3 relative to the residue to be phosphorylated [Citation40]). The positions of these residues, namely S17, S101, T174, S181, T220, S234, and T235, are shown in .
Table 3. Consensus sequences for Chk1-mediated phosphorylation within the kinase domain of CK1δ.
Figure 1. The kinase domain of CK1δ is phosphorylated by Chk1.
The rat CK1δ kinase domain ranges from amino acid 5 to 295 [Citation3] and is covered by the selected GST-CK1δ fusion proteins FP783 and FP881-886. Purified fusion proteins were phosphorylated by Chk1 in vitro. Results are displayed as mean values of triplicates with standard deviation. Phosphate incorporation was quantified by Cherenkov counting and values are provided as counts per minute (cpm).
![Figure 1. The kinase domain of CK1δ is phosphorylated by Chk1.The rat CK1δ kinase domain ranges from amino acid 5 to 295 [Citation3] and is covered by the selected GST-CK1δ fusion proteins FP783 and FP881-886. Purified fusion proteins were phosphorylated by Chk1 in vitro. Results are displayed as mean values of triplicates with standard deviation. Phosphate incorporation was quantified by Cherenkov counting and values are provided as counts per minute (cpm).](/cms/asset/72ecf677-5ee0-4354-b998-fd1de57d604d/tbbb_a_1617105_f0001_b.gif)
GST-CK1δ52−304 (FP882) was equally phosphorylated as GST-CK1δ26−304 (FP881) despite covering a smaller part of CK1δ. Therefore, GST-CK1δ52−304 (FP882) was further analyzed by mass spectrometry in order to identify phosphorylated amino acids. This analysis revealed four residues within the kinase domain of CK1δ being phosphorylated after exposing GST-CK1δ52−304 (FP882) to Chk1 in vitro. Interestingly, two of these were Tyr residues (Y167 and Y179), although tyrosine is not a typical amino acid targeted by Chk1. The two other phosphorylated sites were T161 and S181. For all of these four residues, the phosphorylation probability predicted by the analysis was at least 50% ().
Table 4. Phosphorylated amino acid residues in Chk1-phosphorylated GST-CK1δ52−304 as detected by mass spectrometry.
Chk1 phosphorylates residues T161, T174, T176, and S181 in the CK1δ kinase domain
As shown above, major phosphorylation of CK1δ by Chk1 was detected within amino acids 52 to 304. Therefore, subsequent analysis focused on this region. To identify the sites targeted by Chk1, this region was further divided into four new protein fragments. Additionally, phosphorylation site mutants (Ser/Thr/Tyr to Ala) were created for the predicted sites and those identified by mass spectrometry. Comparison of phosphate incorporation into the new wild-type kinase domain fragments GST-CK1δ52−98 (FP1542), GST-CK1δ98−178 (FP1544), GST-CK1δ178−227 (FP1549), and GST-CK1δ227−304 (FP1553) after Chk1-mediated phosphorylation revealed that the major phosphorylation sites are located between amino acids 98 to 227 ()). Analysis of the phosphorylation site mutants provide evidence that T161 and S181 are phosphorylation sites for Chk1. Phosphorylation of the mutants GST-CK1δ98−178 T161A (FP1545) and GST-CK1δ178−227 S181A (FP1551) was reduced by 51% and 70%, respectively, compared to wild-type fusion proteins ()). For the predicted target site T174, phosphorylation intensity by Chk1 was reduced by 30% for the mutant GST-CK1δ98−178 T174A (FP1547) compared to its respective wild-type CK1δ fragment. The predicted target sites S101 and T220 also seem to be phosphorylated by Chk1 since for both mutant fragments a reduction of the phosphorylation intensity by 34% (GST-CK1δ98−178 S101A; FP1608) and 41% (GST-CK1δ178−227 T220A; FP1609) was observed in comparison to the respective wild-type fragments ()). Information about Chk1-mediated phosphorylation of Y167 and Y179 could not be found by analyzing phosphorylation site mutants GST-CK1δ98−178 Y167A (FP1546) and GST-CK1δ178−227 Y179A (FP1550).
Figure 2. Phosphorylation of GST-CK1δ kinase domain fragments and mutants by Chk1 in vitro.
(a) GST-CK1δ kinase domain fragments were phosphorylated by Chk1 in vitro. Phosphate incorporation was quantified by Cherenkov counting and values are provided as counts per minute (cpm). Results are shown as mean values of triplicates with standard deviation. (b) Wild-type and phosphorylation site mutant GST-CK1δ kinase domain fragments were phosphorylated by Chk1 in vitro. Phosphate incorporation was quantified by Cherenkov counting and results were normalized to the respective GST-CK1δ wild-type kinase domain fragments in (a) and are presented as mean values of triplicates with standard deviation.
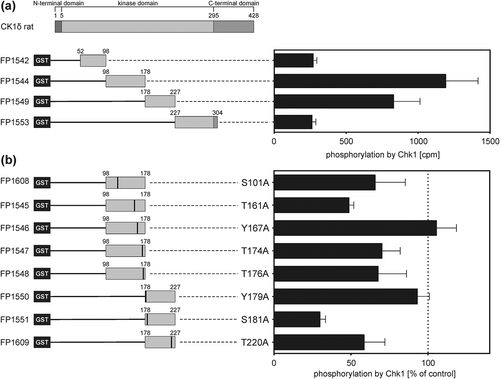
Quantitative data from in vitro kinase assays were validated by two-dimensional phosphopeptide analysis of wild-type or mutant GST-CK1δ kinase domain fragments after phosphorylation by Chk1. The autoradiograph of GST-CK1δ98−178 (FP1544) shows one major phosphopeptide and several minor signals in the surrounding of this peptide ()). For GST-CK1δ98−178 S101A (FP1608) no differences in the analysis of Chk1-phosphorylated wild-type and mutant CK1δ fragment could be observed, leading to the conclusion that S101 is no target for Chk1-mediated phosphorylation (Supplementary Figure 1(a)). In GST-CK1δ98−178 T161A (FP1545), the loss of three minor phosphopeptides compared to the wild-type GST-CK1δ fusion protein was observed ()). Two-dimensional phosphopeptide analysis was also conducted with phosphorylation site mutant GST-CK1δ98−178 T174A (FP1547). Here, the autoradiograph of GST-CK1δ98−178 T174A (FP1547) showed a reduction in the signal intensity of the major phosphopeptide compared to the autoradiograph of GST-CK1δ98−178 (FP1544). Its intensity increased again when the mutant sample was mixed with wild-type sample on the same plate ()). When T174 is mutated to alanine, the signal intensity of the major phosphopeptide was significantly reduced. However, it not completely disappeared, indicating that another phosphorylated residue might be located on the same tryptic peptide. Therefore, also T176 was analyzed for Chk1-mediated phosphorylation. When T176 is mutated to alanine, the phosphorylation intensity of GST-CK1δ98−178 T176A (FP1548) is reduced by 32% compared to the wild-type fragment GST-CK1δ98−178 (FP1544) ()). In subsequent phosphopeptide analysis reduced intensity of the major phosphopeptide was also detected for GST-CK1δ98−178 T176A (FP1548) ()). These results not only confirm phosphorylation of T174 by Chk1 but also lead to the identification of T176 as an additional residue phosphorylated by Chk1.
Figure 3. Two-dimensional phosphopeptide analysis of GST-CK1δ98−178 and phosphorylation site mutants after phosphorylation by Chk1.
GST-CK1δ98−178 (FP1544) and mutants GST-CK1δ98−178 T161A (FP1545) (a), GST-CK1δ98−178 T174A (FP1547) (b), and GST-CK1δ98−178 T176A (FP1548) (c) were phosphorylated by Chk1 in vitro and subsequently processed for two-dimensional phosphopeptide analysis. Sample loading points are indicated by black crosses, the polarity of electrophoretic separation (first dimension) is indicated by (+) and (-). Arrows, as well as the dotted rectangle, indicate positions of phosphopeptides with altered intensity.
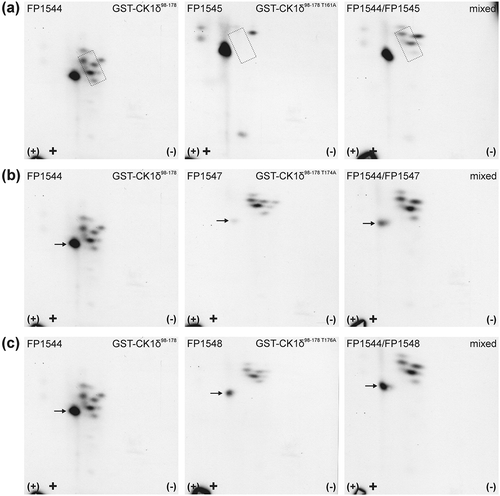
Analysis of GST-CK1δ178−227 (FP1549) showed a pattern of three major phosphopeptides: the peptide migrating furthest in the second dimension appears in different oxidation states which are vertically arranged (). The peptide closest to the loading point exists in two phosphorylated states (single or double phosphorylated), thereby appearing in right top diagonal arrangement. For the peptide marked with the arrow, the signal was lost in the analysis of GST-CK1δ178−227 S181A (FP1551). Hence, S181 could be confirmed as a target for phosphorylation by Chk1.
Figure 4. Two-dimensional phosphopeptide analysis of GST-CK1δ178−227 and phosphorylation site mutant GST-CK1δ178−227 S181A after phosphorylation by Chk1.
GST-CK1δ178−227 (FP1549) and GST-CK1δ178−227 S181A (FP1551) were phosphorylated by Chk1 in vitro and subsequently processed for two-dimensional phosphopeptide analysis. Sample loading points are indicated by black crosses, the polarity of electrophoretic separation (first dimension) is indicated by (+) and (-). Arrows indicate positions of a phosphopeptide with altered intensity. The peptide marked with the asterisk (*) is supposed to be an oxidation artifact of the lower peptide.
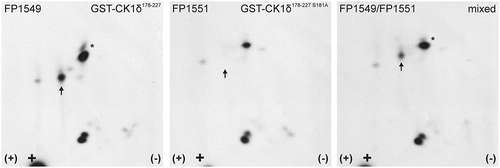
Phosphorylation of T220 could not be confirmed by two-dimensional phosphopeptide analysis, and the phosphopeptide pattern of GST-CK1δ178−227 T220A (FP1609) was comparable to the pattern observed for GST-CK1δ178−227 (FP1549) (Supplementary Figure 1(b)). However, the exchange of T220 to alanine seems to have an effect on phosphorylation of CK1δ by Chk1 in general since phosphorylation intensity of GST-CK1δ178−227 (FP1549) was significantly reduced when the non-targeted residue T220 was mutated to alanine (see data presented in )). Therefore, effects of T220 were also analyzed in subsequent analysis, although phosphorylation of T220 by Chk1 could not be confirmed.
Although the sequences surrounding S17, S234, and T235 also fit the published consensus sequence, phosphorylation of these residues by Chk1 was not further analyzed. GST-CK1δ1−178 (FP783) as well as GST-CK1δ227−305 (FP1553) were only very weakly phosphorylated, indicating that no major phosphorylation site for Chk1 can be found within this part of the kinase domain of CK1δ. Furthermore, phosphorylation of S234 and T235 has not been detected by mass spectrometric analysis. In summary, phosphorylation by Chk1 could be demonstrated for amino acid residues T161, T174, T176, and S181 of CK1δ by comparing phosphorylation intensity and phosphopeptide patterns of CK1δ wild-type and mutant fragments.
Identified Chk1-targeted phosphorylation sites are highly conserved in most eukaryotic CK1 sequences
In order to determine the importance of CK1δ residues T161, T174, T176, S181 and T220 for the regulation of CK1δ, we next investigated whether those residues were conserved in CK1δ sequences in other eukaryotes. We selected CK1 orthologues from human, yeast (Saccharomyces cerevisiae and Schizosaccharomyces pombe), and human parasites (Plasmodium falciparum, Toxoplasma gondii, Trypanosoma brucei, Trypanosoma cruzi, and Leishmania major). Exploring CK1s from human parasites could be particularly informative, as several of these kinases have been shown to be exported from the parasites with the potential to exert their function in their mammalian host cell by replacing or complementing the functions of cellular CK1 isoforms [Citation41,Citation42]. Therefore, we expect that the important regulatory mechanisms identified for CK1δ could be conserved in human parasites as well, as it was previously demonstrated for Leishmania CK1.2 [Citation43]. To this end, we obtained the protein sequences of all available CK1 orthologues from Uniprot [Citation38] and identified the corresponding residues of CK1δ T161, T174, T176, S181, and T220 by performing sequence alignment using T-Coffee [Citation39]. The resulting alignment is presented in Supplementary Figure 2, and the extracted data is presented in and .
Table 5. Analysis of the conservation of CK1δ residues T161, T174, T176, and S181 in different eukaryotes. Neutral substitutions are shown in italic, phospho-mimetic substitutions in bold letters.
Table 6. Analysis of the conservation of CK1δ residue T220 in different eukaryotes. Neutral substitutions are shown in italic, phospho-mimetic substitutions in bold letters.
Interestingly, only two sites, T176 and S181, are conserved in CK1 sequences of all eukaryotes, suggesting that these residues could be crucial for CK1 activity or functions. CK1δ T174 is conserved in all eukaryotes except for two Leishmania orthologues, where it is substituted to valine (amino acid V309) in LmjCK1.4 and to leucine in LmjCK1.3 (amino acid L189). Both substituted amino acids are hydrophobic, contrary to threonine. Nevertheless, this substitution is considered as neutral and therefore might only affect the ability of those two kinases to be regulated by phosphorylation through upstream kinases [Citation44].
It was expected that CK1δ T161, which is part of the T-loop located within the activation loop, would be highly conserved in all eukaryotes, but we found three exceptions among Leishmania CK1s. Threonine at position 161 is substituted to lysine in LmjCK1.5 (K219) and LmjCK1.4 (K296). This substitution is surprising, as it could abrogate phosphorylation and thus affect kinase activity. In LmjCK1.3, the threonine has been substituted by an aspartate (D176). As such substitution is usually mimicking phosphorylation, it suggests that LmjCK1.3 could be constitutively phosphorylated at position 176, although this kind of substitutions is not always perfect phosphorylation mimicry [Citation45].
Residue T220 is remarkable because it is not as conserved as the other residues. We observed four types of substitutions. First, in Plasmodium falciparum CK1, the threonine is substituted to serine. Although it could be considered as neutral, the structural properties of serine are different from those of threonine. Therefore, this substitution could change the structural conformation of the kinase [Citation44]. Second, in four yeast CK1s (YCK1, YCK2, YCK3, and cki2), the threonine residue is substituted to an asparagine. This substitution can be considered as structurally equivalent. However, regulation by phosphorylation of this site is no longer possible. Third, in Leishmania LmjCK1.6, the threonine is substituted to lysine (K230). Finally, the most remarkable substitution could be found in LmjCK1.5 (D283), LmjCK1.4 (D296), and LmjCK1.3 (D234), where the threonine is substituted with aspartate. Regulatory mechanisms mediated by upstream kinases could be bypassed by this phospho-mimicking substitution which imitates constitutive phosphorylation.
CK1δ kinase activity depends on the integrity of Chk1-targeted phosphorylation sites
In order to investigate influences of phosphorylation site mutations on CK1δ kinase activity in vitro, GST fusion proteins of full-length CK1δ with amino acid exchanges T161A, T174A, T176A, S181A, and T220A were designed to analyze the kinetic parameters of these proteins. Kinetic properties of the different fusion proteins were studied by in vitro kinase assays using full-length wild-type and mutant GST-CK1δ fusion proteins to phosphorylate α-casein. Subsequently, kinetic parameters were determined according to the Michaelis-Menten model, and in order to compare the activity of the different mutants, their catalytic efficiency (kcat) was calculated. Mutants GST-CK1δT161A (FP1519), GST-CK1δT174A (FP1521), and GST-CK1δS181A (FP1522) showed catalytic efficiency comparable to wild-type GST-CK1δ (FP1417). However, two mutants differed most significantly from wild-type: GST-CK1δT176A (FP1540) and GST-CK1δT220A (FP1622) showed markedly reduced kinase activity compared to wild-type GST-CK1δ ()). Compared to wild-type GST-CK1δ the catalytic efficiencies of GST-CK1δT176A (FP1540) and GST-CK1δT220A (FP1622) were reduced by 61% and 87%, respectively.
Figure 5. CK1δ kinase activity is affected by modulation of phosphorylation sites targeted by Chk1.
(a) Kinetic analysis of full-length GST-CK1δ fusion proteins. Results of in vitro kinetic analysis using full-length wild-type and mutant GST-CK1δ as kinases and increasing concentrations of α-casein as substrate are provided as catalytic efficiency (kcat) of GST-CK1δ mutants normalized toward wild-type GST-CK1δ. Results are shown as mean values of triplicates with standard deviation. Statistical differences of kcat in comparison to wild-type GST-CK1δ were tested by extra sum-of-squares F-test with *** for p < 0.001 and ns indicating no statistical significance. (b) Analysis of CK1δΔC kinase activity after pre-incubation with Chk1. The activity of CK1δΔC was assayed by analyzing the phosphorylation of α-casein. CK1δΔC without pre-incubation with Chk1 served as control. Results are shown as mean values of triplicates with standard deviation and normalized toward phosphorylation of α-casein by CK1δΔC alone. Statistical significance was tested by unpaired student’s t-test with ns indicating no statistical significance.
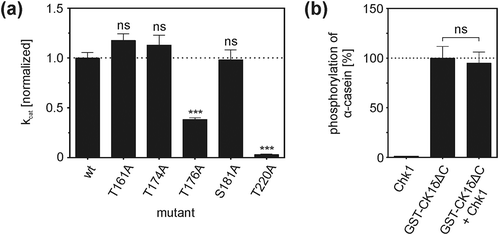
Kinase activity of CK1δΔC is not affected by Chk1-mediated phosphorylation
Co-incubation kinase assays using Chk1 and CK1δ were performed to investigate whether site-specific phosphorylation in the kinase domain of CK1δ shows an impact on its in vitro kinase activity. Because modulation of CK1δ kinase activity by Chk1-mediated phosphorylation of its C-terminal domain has been described previously [Citation21], a CK1δ variant lacking the regulatory C-terminus was used in this experiment (CK1δΔC: a truncated monomer of CK1δ comprising amino acids 1–317). Pre-incubation of CK1δΔC with Chk1 was conducted prior to CK1δΔC-mediated phosphorylation of α-casein. Compared to reactions performed with CK1δΔC only (without pre-incubation with Chk1) no remarkable changes in phosphorylation of α-casein could be observed and kinase activity of CK1δΔC in these reactions was only reduced to 95% ()). Chk1 alone phosphorylated α-casein only to a minor extent when compared to CK1δΔC control reactions (1%).
Discussion
Members of the CK1 family are crucially involved in the regulation of numerous cellular pathways. Therefore, tight regulation of kinase activity of CK1 family members is necessary. We previously demonstrated that CK1δ is C-terminally phosphorylated and regulated by several cellular kinases, including PKA, Chk1, CDKs, and PKCα [Citation21,Citation23–Citation25]. While site-specific phosphorylation within the C-terminal regulatory domain of CK1δ is known to significantly influence kinase activity [Citation21–Citation25], investigation of site-specific phosphorylation within the kinase domain has been neglected. In the present study, we report on Chk1-mediated phosphorylation within the kinase domain of CK1δ, which was analyzed by mass spectrometry and further well-established in vitro techniques.
In 2002, O’Neill et al. proposed a consensus sequence for Chk1 highlighting preferred amino acids relative to a serine or threonine residue to be phosphorylated [Citation40]. Interestingly, not all sites in the CK1δ kinase domain matching the published Chk1 consensus sequence were confirmed by the performed analysis and vice versa, not all sites which were detected by the performed in vitro approaches match the consensus sequence. Especially the examples of T174 and T176 show that sites which not ideally or not at all fit the consensus sequence nevertheless could be targeted by Chk1 in vitro. This shows that apart from the surrounding amino acid sequence also other determinants like protein conformation and resulting protein-protein-interactions are of importance and can lead to a preference also for suboptimal target sites. This hypothesis is supported by previous investigations of C-terminal phosphorylation of CK1δ by Chk1, in which the consensus sequence-based prediction of phosphorylation sites could also only partially be verified by in vitro data [Citation21]. The additional detection of phosphorylation of T174 and T176 shows that by mass spectrometry, often considered to be the gold standard for proteomic analysis, not all phosphorylated sites can be detected. This might be due to technical limitations like small peptide size resulting from tryptic digest, incomplete phosphorylation of the analyzed protein, or phosphorylation loss during sample preparation. Therefore, results obtained from mass spectrometry should always be seen in the context of multiple analysis also involving conventional biochemical approaches like the techniques performed in the course of the present study.
The interpretation of two-dimensional phosphopeptide analysis, however, is challenging – especially when several phosphorylated residues occur within one tryptic peptide, as it is the case for T174 and T176. Here, mutation of either site to alanine does not result in the loss of the phosphopeptide signal in the resulting peptide pattern but in a weaker signal indicating that other phosphorylated sites are present within this peptide. In the case of the analysis of GST-CK1δ178−227 T161A, not only a single but several directly adjacent peptides disappeared compared to the wild-type fragment. Although at least three peptides disappeared when T161 is exchanged to alanine, this can still be an effect mediated only on one peptide and one single phosphorylation site. Here, the diagonal arrangement of the affected peptides indicates that one peptide might contain additional arginine and lysine residues representing peptide variants originating from tryptic digest [Citation46]. In fact, the surrounding sequence of T161 contains multiple cleavage sites for trypsin (lysine and arginine residues). As a result of incomplete digestion, the peptide carrying T161 could be extended by additional sequences from shorter adjacent peptides which contain no additional phosphorylation site and which might lead to the observed phosphopeptide pattern.
Although Chk1 is a serine/threonine-specific protein kinase, two tyrosine residues in GST-CK1δ52−304 were identified as phosphorylation sites by mass spectrometry (Y167 and Y179). However, phosphorylation of these residues could neither be verified by in vitro kinase reactions nor by two-dimensional phosphopeptide analysis (data not shown). Therefore, we consider these to be false-positive results as artifacts arising from sample preparation, measurement, or mathematical analysis of mass spectrometry data.
Sequence alignment of CK1 amino acid sequences of different eukaryotic organisms revealed that Chk1 target sites T176 and S181 are fully conserved, which supports the hypothesis of their importance for CK1 activity and structure. Threonine residues at positions 161 and 174 are conserved among human CK1 isoforms, but are exchanged to serine in Plasmodium (T161) and several CK1 orthologues in yeast (T174). Apart from these conservative exchanges, we identified three orthologues exclusively from Leishmania that diverge from the Chk1 target sequences detected in human CK1δ: LmjCK1.5, LmjCK1.4, and LmjCK1.3. Some of the substitutions we uncover could be neutral and without consequences on the activity and the structure of the kinase, but we do not favor this hypothesis as those sites are rigorously conserved in all the other eukaryotes. Others, such as the threonine to asparagine substitution could have a dramatic impact on CK1 activity as it could bypass the need for regulatory mechanisms. Further investigations will be required to decipher the functions of LmjCK1.5 and LmjCK1.3 as they have not been extensively studied yet [Citation43]. Basic information is available for LmjCK1.4, which is shed into the extracellular environment by the parasite without evidence of export into the host cell. It seems to regulate a key differentiation process for the parasite essential for survival in the insect vector [Citation47].
So far, Chk1 has not been identified in Leishmania making it difficult to conclude whether the regulation mechanisms described for human CK1δ [Citation21] also exist in Leishmania or whether the presence of the aspartate in the Leishmania orthologue bypasses regulation by Chk1. Consistent with the latest hypothesis, a similar phenomenon was previously described for Hsp90, a heat shock protein. An essential threonine residue in trypanosomatides and yeast Hsp90 (T223) was substituted to an aspartate in the human and the mouse orthologues, suggesting that similar to Hsp90 a species-specific regulation of CK1 could be achieved through post-translational modifications despite the high conservation of the protein sequence [Citation48]. Altogether, our findings suggest that contrary to most CK1s, the three kinases LmjCK1.5, LmjCK1.4, and LmjCK1.3 could display Leishmania-specific regulation of CK1.
In order to analyze potential regulatory effects of site-specific phosphorylation, the kinetic parameters of wild-type and phosphorylation site mutant GST-CK1δ full-length fusion proteins were determined. For mutants GST-CK1δT161A (FP1519), GST-CK1δT174A (FP1521), and GST-CK1δS181A (FP1522) no remarkable differences in kinase activity compared to wild-type GST-CK1δ could be observed. Slight differences between the tested kinases are inevitable and may be caused by minor variations in the amount of kinase although protein amounts were carefully adjusted prior to performing the kinetic analysis. However, the major discrepancy between wild-type GST-CK1δ and the mutant GST-CK1δT220A is most striking, although phosphorylation by Chk1 could not be confirmed by phosphopeptide analysis of GST-CK1δ178−227 T220A. Interestingly, the exchange of T220 to alanine seems to have clear consequences as indicated by the altered kinetic parameters of the mutant and it also seems to significantly affect phosphorylation intensity of CK1δ by Chk1 in general. This observation could be explained by changes in the primary amino acid sequence introduced by the T220A exchange and by subsequent conformational changes, which might result from this exchange. After all, the integrity of T220 seems to be important for correct structure and function of the CK1δ protein. Compared to wild-type GST-CK1δ also GST-CK1δT176A showed significantly reduced kinase activity. In general, the integrity of the highly conserved sequence range between amino acids 170 to 180, which is involved in substrate binding, is crucial for the enzymatic function of CK1δ. The amino acid exchange N172D has already been linked to impaired CK1δ kinase activity [Citation49], and furthermore, analysis of a T176I kinase mutant even revealed no residual kinase activity at all [Citation50].
Finally, the direct effects of Chk1-mediated phosphorylation on CK1δ kinase activity were analyzed in vitro. Chk1-mediated phosphorylation events in the CK1δ C-terminal domain can modulate kinase activity [Citation21] and these modulating effects might mask the effects of Chk1-mediated phosphorylation within the CK1δ kinase domain. Therefore, a CK1δ variant lacking the regulatory C-terminal domain (CK1δΔC) was used for this set of experiments. After pre-incubation of CK1δΔC with Chk1 and subsequent phosphorylation of α-casein by CK1δΔC, no remarkable differences in kinase activity could be observed for CK1δΔC pre-incubated with Chk1 in comparison to control reactions without Chk1. This finding indicates that Chk1-mediated effects on CK1δ kinase activity are specific to the respective domain of the kinase protein: site-specific phosphorylation within the C-terminal domain might influence its ability to act as an inhibitory pseudosubstrate and therefore results in downregulation of CK1δ kinase activity [Citation21] while phosphorylation within the kinase domain might modulate the affinity of the kinase to specific substrates, cofactors, or interaction partners in general. As an example, phosphorylation events within the kinase domain of mTOR have already been demonstrated to alter its interaction with the regulatory protein raptor [Citation51]. Also, intramolecular interaction of the kinase domain with the regulatory C-terminal domain might be regulated by site-specific phosphorylation occurring within the kinase domain. This would explain why Chk1-mediated phosphorylation of C-terminally truncated CK1δ (CK1δΔC) shows no effect on kinase activity while mutation of T176 to alanine in full-length CK1δ results in remarkably reduced enzymatic activity. Consequently, phosphorylation of T176 (but also T220) might not directly influence kinase activity but might modulate interaction of the C-terminal regulatory domain with the CK1δ kinase domain resulting in modulation of regulatory effects mediated by the C-terminal domain. Site-specific phosphorylation events within the kinase domain thereby potentially contribute to fine-tuning of CK1δ kinase activity.
In summary, in this study several phosphorylation sites within the kinase domain of CK1δ targeted by Chk1 were identified in vitro, namely T161, T174, T176, and S181. Some of these sites are located at crucial positions for CK1δ kinase activity and are conserved among eukaryotic CK1 sequences. However, the exact mechanism how phosphorylation events within its kinase domain can modulate CK1δ-specific functions and the relevance of this mechanism in vivo remain to be confirmed. The presented results therefore represent a well-founded base for further investigations in appropriate cell culture models and in vivo systems, helping to gain further insights into the regulation of this important group of kinases and thereby broadening the understanding of the pathogenesis of disorders in which dysregulation of CK1 isoforms can be involved.
Author contribution
JB and UK designed the experiments and supervised the study. TB, ZM, PH, and NR performed experiments. JB, UK, and NR evaluated the data sets. JB, UK, DH-B, and NR wrote and revised the manuscript based on input provided by the other authors. All authors were involved in proofreading and the manuscript was submitted after final approval by all authors.
Supplementary_Material_B_hm_et_al.pdf
Download PDF (688.3 KB)Acknowledgments
The authors would like to thank Sebastian Wiese (Core Unit mass spectrometry and Proteomics (CUMP), Ulm University) for performing the mass spectrometry analysis and Sonja Wolff for generation of CK1δ fusion proteins used in the present study.
Disclosure statement
No potential conflict of interest was reported by the authors.
Supplementary material
Supplemental data for this article can be accessed here.
Additional information
Funding
References
- Ardito F, Giuliani M, Perrone D, et al. The crucial role of protein phosphorylation in cell signaling and its use as targeted therapy (Review). Int J Mol Med. 2017 Aug;40(2):271–280. PubMed PMID: 28656226; PubMed Central PMCID: PMCPMC5500920. eng.
- Cohen P. Protein kinases - the major drug targets of the twenty-first century? Nat Rev Drug Discov. 2002 Apr;1(4):309–315. . PubMed PMID: WOS:000178777600020; English.
- Knippschild U, Kruger M, Richter J, et al. The CK1 family: contribution to cellular stress response and its role in carcinogenesis. Front Oncol. 2014;4:96. . PubMed PMID: 24904820; PubMed Central PMCID: PMCPMC4032983. eng.
- Behrend L, Stoter M, Kurth M, et al. Interaction of casein kinase 1 delta (CK1delta) with post-Golgi structures, microtubules and the spindle apparatus. Eur J Cell Biol. 2000 Apr;79(4):240–251. PubMed PMID: 10826492; eng.
- Brockman JL, Gross SD, Sussman MR, et al. Cell cycle-dependent localization of casein kinase I to mitotic spindles. Proc Natl Acad Sci U S A. 1992 Oct 15;89(20):9454–9458. PubMed PMID: 1409656; PubMed Central PMCID: PMCPMC50150. eng.
- Elmore ZC, Guillen RX, Gould KL. The kinase domain of CK1 enzymes contains the localization cue essential for compartmentalized signaling at the spindle pole. Mol Biol Cell. 2018 Jul 1;29(13):1664–1674. . PubMed PMID: 29742018; PubMed Central PMCID: PMCPMC6080649. eng.
- Fulcher LJ, Bozatzi P, Tachie-Menson T, et al. The DUF1669 domain of FAM83 family proteins anchor casein kinase 1 isoforms. Sci Signal. 2018 May 22;11(531). DOI:10.1126/scisignal.aao2341. PubMed PMID: 29789297; PubMed Central PMCID: PMCPMC6025793. eng.
- Greer YE, Rubin JS. Casein kinase 1 delta functions at the centrosome to mediate Wnt-3a-dependent neurite outgrowth. J Cell Biol. 2011 Mar 21;192(6):993–1004. . PubMed PMID: 21422228; PubMed Central PMCID: PMCPMC3063129. eng.
- Greer YE, Westlake CJ, Gao B, et al. Casein kinase 1delta functions at the centrosome and Golgi to promote ciliogenesis. Mol Biol Cell. 2014 May;25(10):1629–1640. PubMed PMID: 24648492; PubMed Central PMCID: PMCPMC4019494. eng.
- Ho Y, Mason S, Kobayashi R, et al. Role of the casein kinase I isoform, Hrr25, and the cell cycle-regulatory transcription factor, SBF, in the transcriptional response to DNA damage in Saccharomyces cerevisiae. Proc Natl Acad Sci U S A. 1997 Jan 21;94(2):581–586. PubMed PMID: 9012827; PubMed Central PMCID: PMCPMC19556. eng.
- Lee C, Etchegaray JP, Cagampang FR, et al. Posttranslational mechanisms regulate the mammalian circadian clock. Cell. 2001 Dec 28;107(7):855–867. PubMed PMID: 11779462; eng.
- Sillibourne JE, Milne DM, Takahashi M, et al. Centrosomal anchoring of the protein kinase CK1delta mediated by attachment to the large, coiled-coil scaffolding protein CG-NAP/AKAP450. J Mol Biol. 2002 Sep 27;322(4):785–797. PubMed PMID: 12270714; eng.
- Wang X, Hoekstra MF, DeMaggio AJ, et al. Prenylated isoforms of yeast casein kinase I, including the novel Yck3p, suppress the gcs1 blockage of cell proliferation from stationary phase. Mol Cell Biol. 1996 Oct;16(10):5375–5385. PubMed PMID: 8816449; PubMed Central PMCID: PMCPMC231536. eng.
- Zyss D, Ebrahimi H, Gergely F. Casein kinase I delta controls centrosome positioning during T cell activation. J Cell Biol. 2011 Nov 28;195(5):781–797. PubMed PMID: 22123863; PubMed Central PMCID: PMCPMC3257584. eng.
- Carmel G, Leichus B, Cheng X, et al. Expression, purification, crystallization, and preliminary x-ray analysis of casein kinase-1 from Schizosaccharomyces pombe. J Biol Chem. 1994 Mar 11;269(10):7304–7309. PubMed PMID: 8125945; eng.
- DeMaggio AJ, Lindberg RA, Hunter T, et al. The budding yeast HRR25 gene product is a casein kinase I isoform. Proc Natl Acad Sci U S A. 1992 Aug 1;89(15):7008–7012. PubMed PMID: 1495994; PubMed Central PMCID: PMCPMC49634. eng.
- Fish KJ, Cegielska A, Getman ME, et al. Isolation and characterization of human Casein Kinase I-Epsilon (Cki), a novel member of the Cki gene family. J Biol Chem. 1995 Jun 23;270(25):14875–14883. PubMed PMID: WOS:A1995RE66600009; English.
- Graves PR, Haas DW, Hagedorn CH, et al. Molecular cloning, expression, and characterization of a 49-kilodalton casein kinase I isoform from rat testis. J Biol Chem. 1993 Mar 25;268(9):6394–6401. PubMed PMID: 8454611; eng.
- Zhai LM, Graves PR, Robinson LC, et al. Casein kinase I-gamma subfamily - molecular-cloning, expression, and characterization of 3 mammalian isoforms and complementation of defects in the Saccharomyces-cerevisiae Yck genes. J Biol Chem. 1995 May 26;270(21):12717–12724. PubMed PMID: WOS:A1995QZ71100061; English.
- Gietzen KF, Virshup DM. Identification of inhibitory autophosphorylation sites in casein kinase I epsilon. J Biol Chem. 1999 Nov 5;274(45):32063–32070. PubMed PMID: 10542239.
- Bischof J, Randoll SJ, Sussner N, et al. CK1 delta kinase activity is modulated by Chk1-mediated phosphorylation. PLoS One. 2013 Jul 4;8(7):e68803. PubMed PMID: WOS:000323350700103; English.
- Eng GWL, Edison, Virshup DM. Site-specific phosphorylation of casein kinase 1 delta (CK1delta) regulates its activity towards the circadian regulator PER2. PLoS One. 2017;12(5):e0177834. . PubMed PMID: 28545154; PubMed Central PMCID: PMCPMC5435336. eng.
- Giamas G, Hirner H, Shoshiashvili L, et al. Phosphorylation of CK1delta: identification of Ser370 as the major phosphorylation site targeted by PKA in vitro and in vivo. Biochem J. 2007 Sep 15;406(3):389–398. PubMed PMID: 17594292; eng.
- Ianes C, Xu PF, Werz N, et al. CK1 delta activity is modulated by CDK2/E- and CDK5/p35-mediated phosphorylation. Amino Acids. 2016 Feb;48(2):579–592. PubMed PMID: WOS:000369307700023; English.
- Meng ZG, Bischof J, Ianes C, et al. CK1 delta kinase activity is modulated by protein kinase C alpha (PKC alpha)-mediated site-specific phosphorylation. Amino Acids. 2016 May;48(5):1185–1197. PubMed PMID: WOS:000374375300006; English.
- Banin S, Moyal L, Shieh S, et al. Enhanced phosphorylation of p53 by ATM in response to DNA damage. Science. 1998 Sep 11;281(5383):1674–1677. PubMed PMID: 9733514; eng.
- Canman CE, Lim DS, Cimprich KA, et al. Activation of the ATM kinase by ionizing radiation and phosphorylation of p53. Science. 1998 Sep 11;281(5383):1677–1679. PubMed PMID: 9733515; eng.
- Tapia-Alveal C, Calonge TM, O’Connell MJ. Regulation of chk1. Cell Div. 2009 Apr 29;4:8. . PubMed PMID: 19400965; PubMed Central PMCID: PMCPMC2685127. eng.
- Dumaz N, Milne DM, Meek DW. Protein kinase CK1 is a p53-threonine 18 kinase which requires prior phosphorylation of serine 15. FEBS Lett. 1999 Dec 17;463(3):312–316. PubMed PMID: 10606744; eng.
- Elyada E, Pribluda A, Goldstein RE, et al. CKIalpha ablation highlights a critical role for p53 in invasiveness control. Nature. 2011 Feb 17;470(7334):409–413. PubMed PMID: 21331045; eng.
- Knippschild U, Milne D, Campbell L, et al. p53 N-terminus-targeted protein kinase activity is stimulated in response to wild type p53 and DNA damage. Oncogene. 1996 Oct 3;13(7):1387–1393. PubMed PMID: 8875976; eng.
- Knippschild U, Milne DM, Campbell LE, et al. p53 is phosphorylated in vitro and in vivo by the delta and epsilon isoforms of casein kinase 1 and enhances the level of casein kinase 1 delta in response to topoisomerase-directed drugs. Oncogene. 1997 Oct 2;15(14):1727–1736. PubMed PMID: 9349507.
- Venerando A, Marin O, Cozza G, et al. Isoform specific phosphorylation of p53 by protein kinase CK1. Cell Mol Life Sci. 2010 Apr;67(7):1105–1118. PubMed PMID: 20041275; eng.
- Kronke J, Fink EC, Hollenbach PW, et al. Lenalidomide induces ubiquitination and degradation of CK1alpha in del(5q) MDS. Nature. 2015 Jul 9;523(7559):183–188. PubMed PMID: 26131937; PubMed Central PMCID: PMCPMC4853910. eng.
- Minzel W, Venkatachalam A, Fink A, et al. Small molecules co-targeting CKIalpha and the transcriptional kinases CDK7/9 control AML in preclinical models. Cell. 2018 Sep 20;175(1):171–185.e25. PubMed PMID: 30146162; eng.
- Shen S, Li C, Dai M, et al. Induction of Huh7 cell apoptosis by HCV core proteins via CK1alphap53Bid signaling pathway. Mol Med Rep. 2018 Jun;17(6):7559–7566. PubMed PMID: 29620268; PubMed Central PMCID: PMCPMC5983949. eng.
- Kusuda J, Hidari N, Hirai M, et al. Sequence analysis of the cDNA for the human casein kinase I delta (CSNK1D) gene and its chromosomal localization. Genomics. 1996 Feb 15;32(1):140–143. PubMed PMID: WOS:A1996TW55800022; English.
- UniProt Consortium T. UniProt: the universal protein knowledgebase. Nucleic Acids Res. 2018 Mar 16;46(5):2699. . PubMed PMID: 29425356; PubMed Central PMCID: PMCPMC5861450. eng.
- Notredame C, Higgins DG, Heringa J. T-Coffee: A novel method for fast and accurate multiple sequence alignment. J Mol Biol. 2000 Sep 8;302(1):205–217. . PubMed PMID: 10964570; eng.
- O’Neill T, Giarratani L, Chen P, et al. Determination of substrate motifs for human Chk1 and hCds1/Chk2 by the oriented peptide library approach. J Biol Chem. 2002 May 3;277(18):16102–16115. PubMed PMID: 11821419; eng.
- Dorin-Semblat D, Demarta-Gatsi C, Hamelin R, et al. Malaria parasite-infected erythrocytes secrete PfCK1, the plasmodium homologue of the pleiotropic protein kinase casein kinase 1. PLoS One. 2015;10(12):e0139591. PubMed PMID: 26629826; PubMed Central PMCID: PMCPMC4668060. eng.
- Silverman JM, Clos J, de’Oliveira CC, et al. An exosome-based secretion pathway is responsible for protein export from Leishmania and communication with macrophages. J Cell Sci. 2010 Mar 15;123(Pt 6):842–852. PubMed PMID: 20159964; eng.
- Rachidi N, Taly JF, Durieu E, et al. Pharmacological assessment defines Leishmania donovani casein kinase 1 as a drug target and reveals important functions in parasite viability and intracellular infection. Antimicrob Agents Chemother. 2014;58(3):1501–1515. . PubMed PMID: 24366737; PubMed Central PMCID: PMCPMC3957854. eng.
- Betts MJ, Russell RB. Amino acid properties and consequences of substitutions. In: Barnes MR, Gray IC, editors. Bioinformatics for geneticists. Hoboken: John Wiley & Sons, Ltd; 2003. p. 289–316.
- Dissmeyer N, Nowack MK, Pusch S, et al. T-loop phosphorylation of arabidopsis CDKA;1 is required for its function and can be partially substituted by an aspartate residue. Plant Cell. 2007 Mar;19(3):972–985. PubMed PMID: 17369369; PubMed Central PMCID: PMCPMC1867360. eng.
- van der Geer P, Hunter T. Phosphopeptide mapping and phosphoamino acid analysis by electrophoresis and chromatography on thin-layer cellulose plates. Electrophoresis. 1994 Mar-Apr;15(3–4):544–554. PubMed PMID: 8055882; eng.
- Dan-Goor M, Nasereddin A, Jaber H, et al. Identification of a secreted casein kinase 1 in Leishmania donovani: effect of protein over expression on parasite growth and virulence. PLoS One. 2013;8(11):e79287. . PubMed PMID: 24260187; PubMed Central PMCID: PMCPMC3829951. eng.
- Morales MA, Watanabe R, Dacher M, et al. Phosphoproteome dynamics reveal heat-shock protein complexes specific to the Leishmania donovani infectious stage. Proc Natl Acad Sci U S A. 2010 May 4;107(18):8381–8386. PubMed PMID: 20404152; PubMed Central PMCID: PMCPMC2889574. eng.
- Hirner H, Gunes C, Bischof J, et al. Impaired CK1 delta activity attenuates SV40-induced cellular transformation in vitro and mouse mammary carcinogenesis in vivo. PLoS One. 2012 Jan 3;7(1):e29709. PubMed PMID: WOS:000301123400093; English.
- Milne DM, Looby P, Meek DW. Catalytic activity of protein kinase CK1 delta (casein kinase 1delta) is essential for its normal subcellular localization. Exp Cell Res. 2001 Feb 1;263(1):43–54. PubMed PMID: 11161704.
- Ekim B, Magnuson B, Acosta-Jaquez HA, et al. mTOR kinase domain phosphorylation promotes mTORC1 signaling, cell growth, and cell cycle progression. Mol Cell Biol. 2011 Jul;31(14):2787–2801. PubMed PMID: 21576368; PubMed Central PMCID: PMCPMC3133410. eng.