ABSTRACT
Ascomycota and basidiomycota fungi are prolific sources of biologically active natural products. Recent genomic data and bioinformatic analysis indicate that fungi possess a large number of biosynthetic gene clusters for bioactive natural products but more than 90% are silent. Heterologous expression in the filamentous fungi as hosts is one of the powerful tools to expression of the silent gene clusters. This review introduces recent studies on the total biosynthesis of representative family members via common platform intermediates, genome mining of novel di- and sesterterpenoids including detailed cyclization pathway, and development of expression host for basidiomycota genes with efficient genome editing method. In addition, this review will discuss the several strategies, for the generation of structural diversity, which are found through these studies.
Graphical Abstract
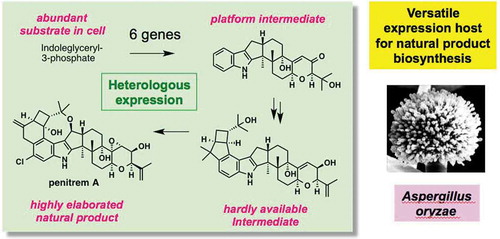
Recent studies on the heterologous expression of fungal bioactive natural products are reviewed. These include total biosynthesis, genome mining, and other related topics.
Microorganisms and plants are prolific producers of biologically active natural products (NPs) which are likely produced for maintenance of their life cycles and other purpose. For many years, humans have utilized these metabolites as pharmaceuticals, pesticides, and cosmetics. Biochemical and genetic knowledge of the NP biosynthesis, rapidly accumulated genomic data and bioinformatic analysis have enabled us to recognize that the biosynthetic genes of NPs in microorganisms are generally clustered, and only parts (<10%) of biosynthetic gene clusters (BGCs) are expressed to yield NPs [Citation1]. Therefore, many researchers are working hard to activate these silent BGCs [Citation2]. There are various approaches such as promoter exchange, epigenetic regulation, and activation of cluster-specific transcription factors have been used to artificially control expression in the source organism [Citation2].
Generally, the numbers of biosynthetic genes for fungal NPs are less than that of metabolites from actinomycetes [Citation3]. We rarely find the fungal BGCs consist of more than 20 genes; the BCG of aflatoxins was one of the largest with 17 genes [Citation4]. Therefore, reconstitution for biosynthetic machinery should be one of the practical strategy to elucidate whole pathway of fungal NPs, and thus the development of versatile heterologous expression system is important. For large-scale production and pathway engineering, promoter/terminator exchange to the host-specific pair is essential (). This refactoring approach is the same as the heterologous production of fungal NPs, suggesting that the refactoring is important for both elucidation and engineering of the pathway. Yeast-based expression of NP biosynthetic genes has been reviewed by Bond et al. [Citation5], and thus, in this review, the author focuses studies using the filamentous fungi such as Asergillus oryzae (AO) as host [Citation6,Citation7]. Usually, our technique and knowledge of NP biosynthesis are limited, and it is thus difficult to propose a detailed biosynthetic pathway of a specific NP. To validate the pathway step by step, we normally introduce the genes.
Figure 1. Outline of heterologous expression of biosynthetic genes for the production of fungal natural products.
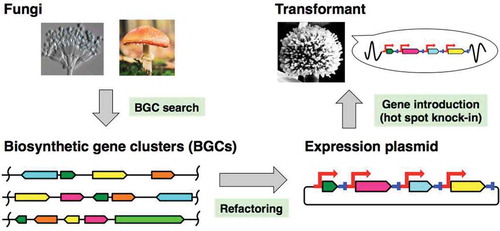
Compared with a functional analysis of a single gene, total biosynthesis approach is more difficult and there are limited number of the related studies. The first topic in this review is total biosynthesis of indole diterpenes (IDTs), describing six examples of highly elaborated IDTs. Next, genome mining of NPs is one of the important topic of heterologous expression of uncharacterized BGCs. The biosynthetic studies of 20 structurally unique di-/sesterterpenes will be reviewed. Then, the several strategies how fungi generate structural diversity, which we found through these topics, will be discussed. Finally, recent challenge on the expression of the genes (genomic DNA) from Basidiomycota fungi is introduced.
Total biosynthesis of fungal natural products
Indole diterpenes
IDTs are representative fungal natural products in fungal metabolites isolated as mycotoxins, antiinsectan, and metabolites with anti-MRSA activity [Citation8,Citation9]. This group member often accompanies less modified constituents as minor constituents. Our biosynthetic studies showed that a fraction of these constituents are biosynthetic intermediates, a finding that significantly facilitated the elucidation of the IDT biosynthetic pathway. Prior to 2010, many reports on the IDT biosynthesis had been published [Citation10], but the detailed function of individual genes and exact pathways were not established. For elucidating the biosynthetic mechanism of IDT core construction, the various AO-transformants (TFs) were prepared by the introduction of four essential genes in a stepwise manner to quadruple auxotrophic strain A. oryzae NSAR-1 [Citation11]. Based on the analysis of products from AO-TFs and subsequent in vitro and in vivo transformations, the biosynthetic pathway of paspaline (1) was determined as shown in [Citation12]. These results clearly showed that 1) prenyltransferase PaxC determines the chain length of oligoprenyl chains; 2) FAD-dependent monooxygenase PaxM is responsible to epoxidize the specific olefin in a stereoselective manner; 3) novel IDT-specific cyclase (PaxB) diversifies the core scaffold by using different cyclization modes. In the case of IDTs, additional enzyme GGPP synthase PaxG is required. This empirical rule can be applied to indole sesquiterpene and non-paspaline IDT derivatives including emindoles [Citation13] and petromindole [Citation14]. Therefore, initial diversification of indole terpenes are generated by these four enzymes homologous to PaxGCMB.
AO-paxGCMBPQ and AO-paxGCMBP prepared from paspaline producing TF generated paxilline (2) and its 13-deoxy analog, suggesting that two optional P450s PaxPQ modified oxidatively the paspaline core. These data provided further experimental evidence to the biogenetic proposal for various IDTs [Citation10]. PaxP catalyzed oxidative elimination of C30 methyl group to introduce the C11-C12 double bond, and PaxP further oxidized 1 at C10 to generate the enone in 2. On the other hand, PaxQ catalyzed allylic oxidation at C13. Orthologs of these two monooxygenases PaxPQ are found in other BCGs have an option to give paspalinine (3) and terpendole I (4). These three intermediates 2, 3, 4 become platforms to generate more elaborated congeners (). Total biosynthesis approach using heterologous expression enabled to show that 3 is converted into aflatrem [Citation15], shearinines (janthitremane) [Citation16], and 4 is a key intermediate of lolitrems (lolitremane) IDTs [Citation17]. In summary, P450s PaxPQ orthologs catalyze unusual oxidative elimination of C1-unit and allylic oxidations, respectively, to generate common core structures.
Penitrem A (5) is a challenging target for total biosynthesis because the penitrem BCG consisted of 17 genes except transcriptional regulator and transporter. To simplify the findings of this research, its biosynthesis is divided into three stages: Stage I: six genes from indole-3-glycerol phosphate to 2; II: six genes from 2 to PC-M4; III: five genes from PC-M4 to 5 (). Using the AO-TFs harboring ptm genes, the experimental results initially confirmed that 2 and PC-M4, isolated from penitrem producer strain, are intermediates in Stages I and III. In this study, screening of the mini-library of TFs carrying two or three genes was effective for narrowing down the candidates. Among the remaining genes, three genes were responsible for synthesizing prenylated product which was further oxidized by the flavoprotein oxidase PtmO to give PC-M5. Finally, we found that prenyltransferase PtmE catalyzed highly unusual cyclization [Citation18].
Similar prenyltransferase JanD and flavoprotein oxidase JanO install a bicyclic system in shearinine [Citation16]. In contrast to penitrem biosynthesis, diprenylation and oxidative cyclization took place in these modifications. Recently, the third mechanism for constructing the bicyclic system in lolitrems [Citation19] such as lolitriol (6) has recently been proposed [Citation20]. Based on the biosynthesis of terpendole [Citation21] and sespendole [Citation22] which has diterpene core and diprenyl indole moiety of lolitrem, we speculated homologous diprenyl transferase LtmE/SpdE and P450 LtmJ/SpdJ are responsible for the construction of the bicyclic system via epoxyalcohol. It was observed that AO-ltmJ converted diprenylterpenedole I into 6 via epoxyalcohol which is similar to that of sespendole. As in the case of three highly elaborated IDTs, decoration of indole ring with bicyclic ring system is another strategy for diversifying IDTs () [Citation17,Citation23].
As described above, AO-expression system have five important advantages compared with other expression systems; 1) rapid preparation of TFs using genomic DNA (based on common splicing system); 2) reliable expression of the introduced gene; 3) supplying sufficient amount of intermediates; 4) rare competing byproduct formation by host enzymes; 5) efficient microbial conversion of the intermediates. With these features, the biosynthetic machinery of IDT biosynthesis can be rapidly elucidated. Furthermore, the addition of extra supporting enzyme genes such as phosphopantetheinyl transferase for polyketides synthase/non-ribosomal peptide synthetase, the P450 reductase and the cognate transporter are not necessary for total biosynthesis. Ascomycetes fungi likely use similar supporting systems, and are resistant to various drugs.
Other examples of total biosynthesis
Total biosynthesis strategy is very effective not only in IDTs but also in polyketide–terpenoid hybrids such as pyripyropene A, andrastin A, anditomin [Citation24] and a number of other related meroterpenoids [Citation25], chevalone [Citation26], mycophenolic acid [Citation27]. In addition, we can find an excellent examples in terpenes (C15: abscisic acid [Citation28,Citation29], C20: aphidicolin [Citation30], C30: demethoxyviridin [Citation31]) and in polyketides (tenellin [Citation32], solanapyrone [Citation33,Citation34]), stipitatic acid [Citation35]). However, total biosynthesis approach is sometimes difficult to achieve for unknown reasons but effectively used to elucidate key reactions of the biosynthetic pathway for the elucidation of core biosynthesis of ustiloxin [Citation36], and squalestatin [Citation37] (). These biosynthetic studies not only provided the detailed information of whole pathway but also the reaction mechanism of unusual oxidative transformations.
Genome mining from silent BCGs
Previous studies on genome mining of fungal NPs
Genome mining is a useful method for the production of novel NPs by expression of silent gene clusters. One of the earliest works are expression type-I PKS genes by Fujii and Ebizuka group. In the genome of Alternaria solani 548, they found more than 10 type-I HR-PKS genes. They expressed them in A. oryzae and isolated four novel metabolites [Citation38,Citation39], including desmethylprosolanapyrone I which led to the identification of Diels-Alderase Sol5 [Citation33]. This was further extended to the transcriptome guided genome mining from the different A. solani strain A-17 to isolate marine NP didymellamide [Citation40].
Once the function of a previously unknown key biosynthetic gene is characterized, homologous BGCs are easily identified by bioinformatic analysis. Since this approach heavily relies on genomic data, there are good chances of finding novel metabolites by heterologous expression of these BGCs and constructing a focused library of a structurally related NPs. However, usually genome mining is far more difficult than normal BGC expression due to the following reasons:
Difficulty to find defect of individual genes from DNA sequences;
Unavailability of expression information to determine the BGC border;
Unavailability of information of NP chemical structure for speculating possible biochemical transformation.
Comparison of homologous genomic data sometimes provided helpful information of defective BGCs including determination of the BGC border. Gibberellin producer Gibberella fujikuroi has a gibberellin BGC and a number of Gibberella sp. also possess gibberellin BGCs. However, it is known that some of the BGC genes are missing or functionally inactive, indicating silent gene clusters may be inactive [Citation41]. In the study of genome mining, one should keep in mind this observation and some of BGCs might be damaged.
Genome mining of di-/sesterterpenes produced by CPF-TS
Recently, we and others succeeded to identify novel type of fungal di-/sesterterpenes which are biosynthesized by novel type of cyclopentane forming terpene synthases (CPF-TSs) [Citation42]. In the biosynthetic study of fusicoccin (7), Sassa, Toyomasu, and coworkers found highly unusual TS (PaFS) with two catalytic domains of prenyltransferase GGPP synthase and diterpene synthase [Citation43]. At the same time, this fusicoccin-producing strain had a similar bifunctional terpene synthase (BFTS) that yielded tetracyclic diterpene methyl phomopsenate [Citation44]. Later, both of fusicoccin and phomopsenate are biosynthesized via the same bicyclic intermediate (8+) [Citation45,Citation46], suggesting that bifunctional TS may generate unique molecular skeletons containing 8-membered ring or multicyclic systems (). Based on this finding, we applied genome mining approach with BFTS genes to find novel diterpenes. When we expressed a BFTS gene AcOS from A. clavatus using the AO expression system, the resultant TF successfully yielded terpene alcohol (). To our surprise, the product was not a diterpene but a sesterterpene which is identical to ophiobolin F [Citation47]. This result suggested that the PT domain of BFTS can supply either C20- or C25-precursor GGPP/GFPP and TS domain produce either C20- or C25-terpenes ().
Phylogenetic analysis of the TS domains of BFTSs found in the public database allowed us to categorize more than 100 BFTSs into two clades I and II which are further divided into six subclades. Intriguingly, four functionally characterized terpene synthases until 2014 fall into clade IIB. This empirical rule implies that the amino acid sequences of fungal CPF-TS are relevant to the cyclization mechanism. Assuming that the amino acid sequence reflects the cyclization mechanism, terpene synthases in all other clades may synthesize novel di-/sesterterpenes. We selected a clade IA-BFTS gene NfFS from Neosartorya fischeri and employed its expression with AO system. In the extracts of AO-NfFS, we obtained a novel sesterterpene named sesterfisherol (9) which has a unique 5-6-8-5 ring system [Citation48]. Its detailed cyclization mechanism was studied and found that the molecular skeleton of 9 was constructed via the bicyclic intermediate 10+ (type-A cyclization, 5–15 membered ring system) similar to 8+ (type-B cyclization, 5–12 membered ring system). Based on common cyclization mechanism of di-/sesterterpenes putatively derived from type-A and type-B cyclizations, we proposed unified biogenesis for these CPF-TSs. Density functional theory (DFT) calculations on the sesterfisherol formation revealed two alternative cyclization mechanisms that were thermodynamically and kinetically favorable. Actually, two metabolites have been found, which are derived via both mechanisms [Citation49,Citation50] ().
To explore terpene synthases producing sesterfisherol-related sesterterpenes, we selected four clade IA-BFTS genes, BmTS1, BmTS2, BmTS3 and PbTS1 from Bipolaris maydis and Phoma betae, which showed moderate identities (35 − 38%) with NfSS. Applying the same strategy as for functional analysis of NfSS, we obtained four sesterterpenes Bm1, Bm2, Bm3, and Pb1 whose structures were closely related with the carbocation intermediates in the cyclization giving 9 [Citation51]. The structure of Bm2 including its absolute configuration is same as that of the tetracyclic carbocation whereas other three products are diastereomers of the bicyclic and tricyclic carbocations. This observation suggested that clade IA CPF-TSs can generate various enantiomeric and diastereomeric cation intermediates that give rise to a diverse array of structural derivatives.
This genome mining approach was attracted by several research groups and nearly 20 CPF-TSs were characterized; Clade IIB: mangicdiene (FgMS) [Citation52], asterifadiene (EvAS) [Citation53], stellatatriene (EvSS) [Citation54], PaFS, AbFS, PcBS, PaPS, and AcOS; Clade IIC: fusaterpenol (FgFS) [Citation55], variediene (EvVS) [Citation56]; Clade IID: conidiogenone (PrDS), PchDTS [Citation57]; Clade IA: NfSS, BmTS1, BmTS2, BmTS3 and PbTS1 [Citation58]; Clade IE: sesterbrasiliatriene synthase (PbSS) [Citation59], preaspterpenacid (AtPS); Clade IF: quiannulatene (EvQS) [Citation60] ().
Figure 7. Di-/sesterterpenes produced by Clade II-terpene synthases and unusual oxidative rearrangement found in the brassicicene biosynthesis.
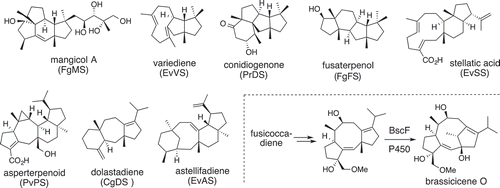
Heterologous expression of genes encoding modification enzymes led to produce putative NPs. With the exception of the BCGs for fusicoccin (14 genes) [Citation61] and brassicicene (>10 genes) [Citation62], BCGs of di-/sesterterpenes belonging in this class usually consist of fewer than 5 genes. To date, the function of these terpenoids remains unknown. Although some of them show strong phytotoxicity (ophiobolin [Citation63]) and anti-viral/antiangiogenic activity (terpestacin [Citation64]), the remaining terpenes do not exhibit any obvious activities. However, conidiation-inducing activity of conidiogenone [Citation57] suggested that these terpenoids act as a signaling molecule in the fungal life cycle. Interestingly, CPF-TSs producing di-/sesterterpenes that have similar molecular skeletons are also found in bacteria [Citation65] and plants [Citation50]. These observations allowed us to propose divergent evolution in these organisms.
Compared with the screening of conventional biological activity-guided natural products, this genome mining approach is very attractive in view of rapid production of target terpenoids and construction of a focused library of structurally unique polycyclic di/sesterterpenoids using genomic data in the absence of strains actively producing these NPs. Based on the genome mining studies, we and other researchers characterized nearly 20 CPF-TS genes and their diversification mechanism has been extensively investigated using heterologous expressions, in vitro analysis and quantum chemical calculations [Citation49]. Including recently characterized nine plant sesterterpene synthases, we expanded the original hypothesis [Citation50] of convergent evolution of terpene synthases from various organisms [Citation41].
Approach to the heterologous expression of basidiomycota genes
Examination of expression of basidiomycota genes with genomic DNA in AO
Mushroom-forming basidiomycete fungi are known to be prolific producers of structurally diverse, bioactive NPs [Citation66]. Recent project [Citation67] has resulted in a number of basidiomycete genomic data from hundreds of basidiomycete taxa that can now be searched in silico for NP BGCs. However, despite their incredible potential for NP discovery, Basidiomycota fungi are a largely unexplored territory for drug discovery compared to Ascomycota fungi. Many Basidiomycota strains are difficult to grow under laboratory conditions, requiring the determination of suitable growth conditions and often long fermentation times. Even if a gene is expressed and the corresponding cDNA can be prepared, nonfunctional alternative splice variants are often produced [Citation68]. Unfortunately, Basidiomycota have very intron-rich genomes and genes that contain very small and unpredictable exons, especially in cytochrome P450 monooxygenase genes, and thus currently no reliable tool for automated intron prediction is available. This hampers the use of synthetic cDNA for expression although several heterologous expression studies with correct cDNA have afforded target products [Citation69–Citation72] (). Being able to directly express Basidiomycota biosynthetic genes from genomic DNA in a suitable fungal surrogate host would therefore greatly accelerate the functional characterization of NP pathways.
Currently, AO expression system covers all ascomycete fungal genera to yield various type of NPs [Citation6,Citation8]. However, there is no report on heterologous production of metabolites from basidiomycota fungi. Comparison of splicing mechanism between ascomycete and basidiomycota fungi suggested two fungi have similar but different from that of yeasts [Citation73]. To examine a possibility that AO is a suitable host for Basidiomycota gene expression, cDNA expression of pleuromutilin gene cluster in AO was initially examined. Pleuromutilin (11) is the widely used livestock antibiotic and is approved for human use. Its gene cluster consisted of seven genes which are responsible for core construction (ple3/4), oxidative core modifications (ple5/6/7), and side chain introduction (ple1/2). HPLC profiles of three AO-TFs allowed us to confirm the entire biosynthetic pathway [Citation74] ().
In this biosynthetic study, it was observed that the native AO NADPH cytochrome P450 reductase (CPR) is sufficient for showing the activity of the Basidiomycota P450 monooxygenases [Citation74]. In addition, the cloning of functional genes from Basidiomycota cDNA was difficult and tedious. Therefore, heterologous expression of biosynthetic genes for 11 was examined by using genomic DNAs. Two genes ple3/4 for core construction were correctly spliced and the corresponding TF AO-ple3/4 produced premutilin. Three intron-rich P450 genes ple1/5/6 were mostly spliced in three single gene-TFs (2 non-spliced introns remained out of 34 introns) [Citation75]. Based on these promising results, it was speculated that gene splicing in basidiomycetes resembles that in ascomycete fungi [Citation73].
To establish the versatility of basidiomycete heterologous expression using A. oryzae, the exhaustive expression of sesquiterpene synthases (STSs) were examined because their genes are prolific in basidiomycete genomes and their expression are readily detected by GC-MS analysis of volatile products. In this work, 30 STS genes were selected for characterization from two basidiomycete fungi: pleuromutilin producer Clitopilus pseudo-pinsitus and Stereum hirsutum, which was previously investigated and found only five genes were expressed [Citation76].
To ensure the expression of target genes, the recently developed technique, a fungal clustered regularly interspaced short palindromic repeat (CRISPR)-Cas9 system specifically optimized for A. oryzae was used [Citation77]. In addition, the STS genes were introduced (knocked-in) to the hot spot (HS) loci where target genes were integrated in the highly yielding TFs found in our previous study. GC-MS analysis of products from the resultant TFs revealed that 16 TFs successfully gave the products indicating expression of STS genes correctly spliced. Sequence analysis of cDNAs recovered from the TFs revealed the remaining 14 TFs contained non-spliced introns (13%, 17 introns/132 total introns) which were simply skipped and no complicated missplicing was found. Following the removal of non-spliced introns (1 or 2 introns/gene) in amplified cDNA by PCR-based techniques, the fixed cDNAs were expressed by E. coli which successfully yielded terpene products except a single TF [Citation75].
Total biosynthesis of erinacine Q with genomic DNA
Finally, to confirm the versatility of the AO expression system, total biosynthesis of a typical Basidiomycota metabolite erinacine Q (12) was conducted, which is known as a potent stimulating activator for nerve growth-factor synthesis. In 2017, an erinacine BCG that contained of eriEG (core construction), eriACI (three P450 hydroxylases), and eriJ (glycosyltransferase) was identified in the genome of Hericium erinaceus. Considering other essential transformations (acetylation and oxidation), two more genes eriKL whose homologues are present in other erinacine/cyathin gene clusters were included for the transformation [Citation78]. Initially, we prepared AO-transformants carrying the genomic DNA sequences of eriA-C, and eriH-L by the HS-knock-in, and examined their expressions and sequences of the resultant cDNA. Among 65 introns in total, only six non-spliced introns (9.2%) remained in the corresponding genes. The introns that remained were removed by simple PCR methods [Citation79] ().
Using various TFs prepared by HS-knock-in, a newly developed genome editing method, diterpene formation (EriEG) and the first C14-hydroxylation (EriI) were confirmed by the analysis of the products from the TFs. When the sequential hydroxylations at C11 and C15 of the seven-membered ring was examined by the TFs carrying three P450 genes eriAC, it was found that byproduct formation occurred in EriC-catalyzed C15-hydroxylation and no conversion took place in the EriA-catalyzed C11-hydroxylation. These unexpected problems were addressed by the introduction of the all remaining genes eriBHKL to the five gene-TF AO-eriEGACIJ. The resultant 10 gene-TF (AO-eriEGACIJKLBH) produced acetate and its oxidized product. After extensive analysis, it was found that a short chain dehydrogenase/reductase (SDR) EriH is responsible for assisting P450 reactions catalyzed by EriAC. Alternatively, cognate P450 reductase (CPR) from H. erinaceus also helped P450 reactions. The function of SDR is currently unknown but this phenomenon is likely mushroom specific due to the presence of EriH homologs in other basidiomycete terpene BCGs [Citation80].
Although the 10 gene-TF AO-eriEGACIJKLBH did not give final product 12, it yielded glucosylated product erinacine Q2, suggesting that UDP-xylose is absent, but its precursor UDP-glucose is sufficient in AO. This was supported by in vitro analysis of glycosyltransferase EriJ which catalyzed glycosylation of acetate with UDP-xylose eight times faster than that of UDP-glucose. To complete total biosynthesis of 12, plant enzyme genes AtUGD1 and AtUXS3 were introduced to supply UDP-xylose [Citation81]. For this purpose, a forced recycling system of Cas9 plasmids was applied, thereby allowing repeated HS-knock-in for the introduction of novel genes [Citation82]. Ten gene-TF AO-eriEGACIJL/AtUGD1/AtUXS3 successfully produced 12 together with acetate and erinacine Q2. Overall, the complete elucidation of the erinacine biosynthetic pathway was achieved.
Conclusion and perspective
Since 2010, heterologous expression studies of fungal metabolites increased dramatically. Expression hosts correctly spliced genomic DNAs of NP BGCs from most genera of fungi to yield various NPs including polyketides, terpenes, peptides, and alkaloids. This approach usually gives a sufficient amount for the characterization of metabolites from cultures (0.1–1.0 L). However, for large scale-production of, for instance, artemisinin and its precursor artemisinic acid, proper engineering of the heterologous host (yeast) suggested to improve its titer for industrial use [Citation83]. Currently, heterologous expression is recognized as one of the powerful methods for NP production.
To date, most of the biosynthetic studies of fungal NPs have focused specific targets. In this review, the author introduced the exhaustive expression of BCGs for specific fungal metabolite families such as IDTs and di-/sesterterpenes. These studies presented herein provided information on the strategy of fungi for generating structural diverse NPs. For example, in IDT biosynthesis, three stages (1: core scaffold synthesis; 2: common intermediate synthesis; 3: final oxidative modifications) are involved in the diversification. In the case of TSs for the di-/sesterterpenes, among various options available for cyclizations, fungi prefer initial cyclopentane formation to reduce the conformational freedom and generate 11- or 12-membered ring with diene and carbocation, just like the intermediates of STSs. Intriguingly, bacteria and plants adopt the same strategy, suggesting the occurrence of convergent evolution. These data are only available from exhaustive expression of BCGs, thus, this focused approach is new and is totally different from conventional bioassay-guided screening.
As described in the section of heterologous expression of Basidiomycota genes, genome editing techniques have revolutionized heterologous expression. Recycling of Cas9 plasmid enables unlimited introduction of biosynthetic genes. HS knock-in transformation guarantees a good expression of an introduced gene. Thus, the combination of HS knock-in and plasmid recycling enabled us to avoid a time-consuming screening process. In the case of erinacine biosynthesis, we encountered unexpected observations on the mushroom P450 supporting systems. In this example, genome mining still involved many problems to solve. Therefore, we need to gain more experience to ensure success in heterologous expressions. Basically, enzymatic synthesis of biologically active NPs solely depends on the information of the DNA sequence of the gene. In the future, automated systems may generate TFs with the plasmids carrying genes derived from synthetic DNA. Once established these systems, we can use them to produce novel NPs by the TFs expressing whole genes of unidentified BGCs. For this purpose, we need to gain more knowledge on gene regulation for the cooperation of primary metabolism, avoiding the toxicity of the generated NPs and ensuring a sufficient supply of the starting material.
Acknowledgments
The studies described in this review were performed at the Department of Chemistry, Faculty of Science, Hokkaido University. The author would like to thank all the contributors, students, postdoctoral fellows, staffs, and colleagues of Hokkaido University. I am most grateful to all of my collaborators, particularly to Dr. Takeshi Sassa, Professor Emeritus of the University of Yamagata, for his collaboration in the early stage of fungal metabolite biosynthesis. I am also grateful to the late Dr. Akitami Ichihara, and Dr. Sadao Sakamura, Professor Emeritus of the Hokkaido University, for their warm encouragement. The studies described here were financially supported by JSPS and MEXT.
Disclosure statement
No potential conflict of interest was reported by the author.
References
- Brakhage AA. Regulation of fungal secondary metabolism. Nat Rev Microbiol. 2013;11:21–32.
- Zhang JJ, Tang X, Moore BS. Genetic platforms for heterologous expression of microbial natural products. Nat Prod Rep. 2019;36:1313–1332.
- Medema MH, Kottmann R, Yilmaz P, et al. Minimum information about a biosynthetic gene cluster. Nat Chem Biol. 2015;11:625–631. https://mibig.secondarymetabolites.org/
- Yu JJ. Current understanding on aflatoxin biosynthesis and future perspective in reducing aflatoxin contamination. Toxins (Basel). 2012;4:1024–1057.
- Bond C, Tang Y, Li L. Saccharomyces cerevisiae as a tool for mining, studying and engineering fungal polyketide synthases. Fungal Genet Biol. 2016;89:52–61.
- Fujii I. Heterologous expression systems for polyketide synthases. Nat Prod Rep. 2009;26:155–169.
- Lazarus CM, Williams K, Bailey AM. Reconstructing fungal natural product biosynthetic pathways. Nat Prod Rep. 2014;31:1339–1347.
- Minami A, Liu CW, Oikawa H. Total biosynthesis of fungal indole diterpenes using cell factories. Heterocycles. 2016;92:397–421.
- Comprehensive natural products III: chemistry and Biology. In: Begley TP, Liu H–W, editors. Biosynthesis of indole diterpenes. Vol. 2. Elsevier Science; in press.
- Saikia S, Nicholson MJ, Young C, et al. The genetic basis for indole-diterpene chemical diversity in filamentous fungi. Mycol Res. 2008;112:184–199.
- Jin FJ, Maruyama J, Juvvadi PR, et al. Development of a novel quadruple auxotrophic host transformation system by argB gene disruption using adeA gene and exploiting adenine auxotrophy in Aspergillus oryzae. FEMS Microbiol Lett. 2004;239:79–85.
- Tagami K, Liu C, Minami A, et al. Reconstitution of biosynthetic machinery for indole-diterpene paxilline in Aspergillus oryzae. J Am Chem Soc. 2013;135:1260–1263.
- Tang MC, Lin HC, Li DH, et al. Discovery of unclustered fungal indole diterpene biosynthetic pathways through combinatorial pathway reassembly in engineered yeast. J Am Chem Soc. 2015;137:13724–13727.
- Xiong QB, Zhu XW, Wilson WK, et al. Enzymatic synthesis of an indole diterpene by an oxidosqualene cyclase: mechanistic, biosynthetic, and phylogenetic implications. J Am Chem Soc. 2003;125:9002–9003.
- Tagami K, Minami A, Fujii R, et al. Rapid reconstitution of biosynthetic machinery for fungal metabolites in Aspergillus oryzae: total biosynthesis of aflatrem. ChemBioChem. 2014;15:2076–2080.
- Liu C, Minami A, Dairi T, et al. Biosynthesis of shearinine: diversification of a tandem prenyl moiety of fungal indole diterpenes. Org Lett. 2016;18:5026–5029.
- Motoyama T, Hayashi T, Hirota H, et al. Terpendole E, a Kinesin Eg5 inhibitor, is a key biosynthetic intermediate of indole-diterpenes in the producing fungus Chaunopycnis alba. Chem Biol. 2012;19:1611–1619.
- Liu C, Tagami K, Minami A, et al. Reconstitution of biosynthetic machinery for the synthesis of the highly elaborated indole diterpene penitrem. Angew Chem Int Ed. 2015;54:5748–5752.
- Saikia S, Takemoto D, Tapper BA, et al. Functional analysis of an indole-diterpene gene cluster for lolitrem B biosynthesis in the grass endosymbiont Epichloe festucae. FEBS Lett. 2012;586:2563–2569.
- Huang XH, Nishida H, Tomoda H, et al. Terpendoles, novel acat inhibitors produced by Albophoma yamanashiensis 2. structure elucidation of Terpendole-A, Terpendole-B, Terpendole-C and Terpendole-D. J Antibiot. 1995;48:5–11.
- Uchida R, Kim YP, Nagamitsu T, et al. Structure elucidation of fungal sespendole, an inhibitor of lipid droplet synthesis in macrophages. J Antibiot. 2006;59:338–344.
- Jiang U, Ozaki T, Liu C, et al., unpublished results.
- Kudo K, Liu CW, Matsumoto T, et al. Heterologous biosynthesis of fungal indole sesquiterpene sespendole. ChemBioChem. 2018;19:1492–1497.
- Matsuda Y, Abe I. Biosynthesis of fungal meroterpenoids. Nat Prod Rep. 2016;33:26–53.
- Matsuda Y, Bai TX, Phippen CBW, et al. Novofumigatonin biosynthesis involves a non-heme iron-dependent endoperoxide isomerase for orthoester formation. Nat Commun. 2018;9:2587.
- Wang WG, Du LQ, Sheng SL, et al. Genome mining for fungal polyketide-diterpenoid hybrids: discovery of key terpene cyclases and multifunctional P450s for structural diversification. Org Chem Front. 2019;6:571–578.
- Hansen BG, Mnich E, Nielsen KF, et al. Involvement of a natural fusion of a cytochrome P450 and a hydrolase in mycophenolic acid biosynthesis. Appl Environ Microbiol. 2012;78:4908–4913.
- Takino J, Kozaki T, Sato Y, et al. Unveiling biosynthesis of the phytohormone abscisic acid in fungi: unprecedented mechanism of core scaffold formation catalyzed by an unusual sesquiterpene synthase. J Am Chem Soc. 2018;140:12392–12395.
- Takino J, Kozaki T, Ozaki T, et al. Elucidation of biosynthetic pathway of a plant hormone abscisic acid in phytopathogenic fungi. Biosci Biotech Bioch. 2019;83:1642–1649.
- Fujii R, Minami A, Tsukagoshi T, et al. Total biosynthesis of diterpene aphidicolin, a specific inhibitor of DNA polymerase alpha: heterologous expression of four biosynthetic genes in Aspergillus oryzae. Biosci Biotechnol Biochem. 2011;75:1813–1817.
- Wang GQ, Chen GD, Qin SY, et al. Biosynthetic pathway for furanosteroid demethoxyviridin and identification of an unusual pregnane side-chain cleavage. Nat Commun. 2018;9:1838.
- Heneghan MN, Yakasai AA, Halo LM, et al. First heterologous reconstruction of a complete functional fungal biosynthetic multigene cluster. ChemBioChem. 2010;11:1508–1512.
- Kasahara K, Miyamoto T, Fujimoto T, et al. Solanapyrone synthase, a possible Diels-Alderase and iterative type I polyketide synthase encoded in a biosynthetic gene cluster from Alternaria solani. ChemBioChem. 2010;11:1245–1252.
- Fujii R, Ugai T, Ichinose H, et al. Reconstitution of biosynthetic machinery of fungal polyketides: unexpected oxidations of biosynthetic intermediates by expression host. Biosci Biotech Bioch. 2016;80:426–431.
- Davison J, Al Fahad A, Cai M, et al. Genetic, molecular, and biochemical basis of fungal tropolone biosynthesis. Proc Natl Acad Sci USA. 2012;109:7642–7647.
- Ye Y, Minami A, Igarashi Y, et al. Unveiling the biosynthetic pathway of the ribosomally synthesized and post-translationally modified peptide Ustiloxin B in filamentous fungi. Angew Chem Int Ed. 2016;55:8072–8075.
- Bonsch B, Belt V, Bartel C, et al. Identification of genes encoding squalestatin S1 biosynthesis and in vitro production of new squalestatin analogues. Chem Commun. 2016;52:6777–6780.
- Fujii I, Yoshida N, Shimomaki S, et al. An iterative Type I polyketide synthase PKSN catalyzes synthesis of the decaketide alternapyrone with regiospecific octa-methylation. Chem Biol. 2005;12:1301–1309.
- Kasahara K, Fujii I, Oikawa H, et al. Expression of Alternaria solani PKSF generates a set of complex reduced-type polyketides with different carbon-lengths and cyclization. ChemBioChem. 2006;7:920–924.
- Ugai T, Minami A, Gomi K, et al. Genome mining approach for harnessing the cryptic gene cluster in Alternaria solani: production of PKS-NRPS hybrid metabolite, didymellamide B. Tetrahedron Lett. 2016;57:2793–2796.
- Minami A, Ozaki T, Liu C, et al. Cyclopentane-forming di/sesterterpene synthases: widely distributed enzymes in bacteria, fungi, and plants. Nat Prod Rep. 2018;35:1330–1346.
- Wiemann P, Sieber CM, von Bargen KW, et al. Deciphering the cryptic genome: genome-wide analyses of the rice pathogen Fusarium fujikuroi reveal complex regulation of secondary metabolism and novel metabolites. PLoS Pathog. 2013;9:e1003475.
- Toyomasu T, Tsukahara M, Kaneko A, et al. Fusicoccins are biosynthesized by an unusual chimera diterpene synthase in fungi. Proc Natl Acad Sci USA. 2007;104:3084–3088.
- Toyomasu T, Kaneko A, Tokiwano T, et al. Biosynthetic gene-based secondary metabolite screening: a new diterpene, methyl phomopsenonate, from the fungus Phomopsis amygdali. J Org Chem. 2009;74:1541–1548.
- Toyomasu T, Tsukahara M, Kenmoku H, et al. Transannular proton transfer in the cyclization of geranylgeranyl diphosphate to fusicoccadiene, a biosynthetic intermediate of fusicoccins. Org Lett. 2009;11:3044–3047.
- Shinde SS, Minami A, Chen Z, et al. Cyclization mechanism of phomopsene synthase: mass spectrometry based analysis of various site-specifically labeled terpenes. J Antibiot (Tokyo). 2017;70:632–638.
- Chiba R, Minami A, Gomi K, et al. Identification of ophiobolin F synthase by a genome mining approach: a sesterterpene synthase from Aspergillus clavatus. Org Lett. 2013;15:594–597.
- Ye Y, Minami A, Mandi A, et al. Genome mining for sesterterpenes using bifunctional terpene synthases reveals a unified intermediate of di/sesterterpenes. J Am Chem Soc. 2015;137:11846–11853.
- Sato H, Narita K, Minami A, et al. Theoretical study of sesterfisherol biosynthesis: computational prediction of key amino acid residue in terpene synthase. Sci Rep. 2018;8:2473.
- Huang AC, Kautsar SA, Hong YJ, et al. Unearthing a sesterterpene biosynthetic repertoire in the Brassicaceae through genome mining reveals convergent evolution. Proc Natl Acad Sci USA. 2017;114:E6005–E6014.
- Narita K, Sato H, Minami A, et al. Focused genome mining of structurally related sesterterpenes: enzymatic formation of enantiomeric and diastereomeric products. Org Lett. 2017;19:6696–6699.
- Bian G, Han Y, Hou A, et al. Releasing the potential power of terpene synthases by a robust precursor supply platform. Metab Eng. 2017;42:1–8.
- Matsuda Y, Mitsuhashi T, Lee S, et al. Astellifadiene: structure determination by NMR spectroscopy and crystalline sponge method, and elucidation of its biosynthesis. Angew Chem Int Ed. 2016;55:5785–5788.
- Matsuda Y, Mitsuhashi T, Quan Z, et al. Molecular basis for stellatic acid biosynthesis: a genome mining approach for discovery of sesterterpene synthases. Org Lett. 2015;17:4644–4647.
- Rinkel J, Steiner S, Bian G, et al. A family of related fungal and bacterial di- and sesterterpenes: studies on fusaterpenol and variediene. ChemBioChem. 2019.
- Qin B, Matsuda Y, Mori T, et al. An unusual chimeric diterpene synthase from Emericella variecolor and its functional conversion into a sesterterpene synthase by domain swapping. Angew Chem Int Ed. 2016;55:1658–1661.
- Shiina T, Nakagawa K, Fujisaki Y, et al. Biosynthetic study of conidiation-inducing factor conidiogenone: heterologous production and cyclization mechanism of a key bifunctional diterpene synthase. Biosci Biotech Bioch. 2019;83:192–201.
- Gao L, Narita K, Ozaki T, et al. Identification of novel sesterterpenes by genome mining of phytopathogenic fungi Phoma and Colletotrichum sp. Tetrahedron Lett. 2018;59:1136–1139.
- Mitsuhashi T, Rinkel J, Okada M, et al. Mechanistic characterization of two chimeric sesterterpene synthases from Penicillium. Chem Eur J. 2017;23:10053–10057.
- Okada M, Matsuda Y, Mitsuhashi T, et al. Genome-based discovery of an unprecedented cyclization mode in fungal sesterterpenoid biosynthesis. J Am Chem Soc. 2016;138:10011–10018.
- Noike M, Ono Y, Araki Y, et al. Molecular breeding of a fungus producing a precursor diterpene suitable for semi-synthesis by dissection of the biosynthetic machinery. Plos One. 2012;7:e42090.
- Tazawa A, Ye Y, Ozaki T, et al. Total biosynthesis of brassicicenes: identification of a key enzyme for skeletal diversification. Org Lett. 2018;20:6178–6182.
- Narita K, Chiba R, Minami A, et al. Multiple oxidative modifications in the ophiobolin biosynthesis: P450 oxidations found in genome mining. Org Lett. 2016;18:1980–1983.
- Narita K, Minami A, Ozaki T, et al. Total biosynthesis of antiangiogenic agent (-)-terpestacin by artificial reconstitution of the biosynthetic machinery in Aspergillus oryzae. J Org Chem. 2018;83:7042–7048.
- Rinkel J, Steiner ST, Dickschat JS. Diterpene biosynthesis in actinomycetes: studies on cattleyene synthase and phomopsene synthase. Angew Chem Int Ed. 2019;58:9230–9233.
- Masuya T, Tsunematsu Y, Hirayama Y, et al. Biosynthesis of lagopodins in mushroom involves a complex network of oxidation reactions. Org Biomol Chem. 2019;17:234–239.
- Brandt P, Garcia-Altares M, Nett M, et al. Induced chemical defense of a mushroom by a double-bond-shifting polyene synthase. Angew Chem Int Ed. 2017;56:5937–5941.
- Nofiani R, de Mattos-shipley K, Lebe KE, et al. Strobilurin biosynthesis in basidiomycete fungi. Nature Commun. 2018;9:3940.
- Tang MC, Fischer CR, Chari JV, et al. Thioesterase-catalyzed aminoacylation and thiolation of polyketides in fungi. J Am Chem Soc. 2019;141:8198–8206.
- Lorenzen K, Anke T. Basidiomycetes as a source for new bioactive natural products. Curr Org Chem. 1998;2:329–364.
- Martin F, Cullen D, Hibbett D, et al. Sequencing the fungal tree of life. New Phytol. 2011;190:818–821.
- Quin MB, Flynn CM, Schmidt-Dannert C. Traversing the fungal terpenome. Nat Prod Rep. 2014;31:1449–1473.
- Kupfer DM, Drabenstot SD, Buchanan KL, et al. Introns and splicing elements of five diverse fungi. Eukaryot Cell. 2004;3:1088–1100.
- Yamane M, Minami A, Liu C, et al. Biosynthetic machinery of diterpene pleuromutilin isolated from basidiomycete fungi. ChemBioChem. 2017;18:2317–2322.
- Nagamine S, Liu C, Nishishita J, et al. Ascomycete Aspergillus oryzae is an efficient expression host for production of basidiomycete terpenes by using genomic DNA sequences. Appl Environ Microb. 2019;85:e00409–19.
- Quin MB, Flynn CM, Wawrzyn GT, et al. Mushroom hunting by using bioinformatics: application of a predictive framework facilitates the selective identification of sesquiterpene synthases in basidiomycota. ChemBioChem. 2013;14:2480–2491.
- Katayama T, Tanaka Y, Okabe T, et al. Development of a genome editing technique using the CRISPR/Cas9 system in the industrial filamentous fungus Aspergillus oryzae. Biotechnol Lett. 2016;38:637–642.
- Yang YL, Zhang SS, Ma K, et al. Discovery and characterization of a new family of diterpene cyclases in bacteria and fungi. Angew Chem Int Ed. 2017;56:4749–4752.
- Liu C, Minami A, Ozaki T, et al. Efficient reconstitution of Basidiomycota diterpene erinacine gene cluster in Ascomycota host Aspergillus oryzae based on genomic DNA sequences. J Am Chem Soc. 2019;141:15519–15523.
- Wick J, Heine D, Lackner G, et al. A fivefold parallelized biosynthetic process secures chlorination of Armillaria mellea (Honey Mushroom) Toxins. Appl Environ Microb. 2016;82:1196–1204.
- Oka T, Jigami Y. Reconstruction of de novo pathway for synthesis of UDP-glucuronic acid and UDP-xylose from intrinsic UDP-glucose in Saccharomyces cerevisiae. Febs J. 2006;273:2645–2657.
- Katayama T, Nakamura H, Zhang Y, et al. Forced recycling of an AMA1-based genome-editing plasmid allows for efficient multiple gene deletion/integration in the industrial filamentous fungus Aspergillus oryzae. Appl Environ Microb. 2019;85:e01896–18.
- Paddon CJ, Westfall PJ, Pitera DJ, et al. High-level semi-synthetic production of the potent antimalarial artemisinin. Nature. 2013;496:528–532.