ABSTRACT
Red kidney beans (Phaseolus vulgaris L.) contain bioactive compounds that are known to exhibit antidiabetic effects via inhibition of α-glucosidase. However, information on the nonpolar components that exhibit antidiabetic activity is limited. Here, we report the isolation and structure determination of components with α-glucosidase inhibitory activity, which were obtained from the hexane extract of red kidney beans. Triacylglycerols (TAGs) were identified as the major components exhibiting inhibitory activity against α-glucosidase. The chemical structure of TAGs was determined by a combination of GC-MS and UPLC-MS/MS. The primary TAGs identified were LnLnLn (trilinolenin) and LnLLn (1,3-dilinolenoyl-2-linoleoyl glycerol). The major fatty acids present in these TAGs were α-linolenic acid (ω-3) and linoleic acid (ω-6). These TAGs were also found to inhibit the α-glucosidase activity in a similar fashion as acarbose. These results suggest that TAGs have potency as antidiabetics and support the potential suitability of red kidney beans for diabetes treatment.
Graphical abstract
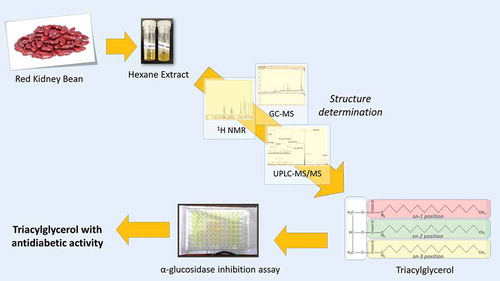
The dominant lipophilic compound of red kidney bean were identified as triacylglycerol and known to have α-glucosidase inhibition activity
Red kidney beans (Phaseolus vulgaris L) are one of the important legume plants cultivated in Indonesia and other parts of East Asia. This plant contains numerous bioactive compounds and some nutritional components, such as proteins, resistant starch, dietary fiber, and fat [Citation1–Citation3]. The seed coat of red kidney beans is red, which indicates that it may be a good source of polyphenols as colored beans are often found to contain polyphenols. Several previous studies have shown that these beans contain polyphenol compounds [Citation4–Citation9] and saponins [Citation10,Citation11], which exhibit antidiabetic activity.
Diabetes refers to a disorder where the uptake of glucose in blood by insulin is insufficient. When not well managed, this disease can lead to complications in several parts of the body [Citation12]. It is known that diabetes can be managed and prevented through diet, especially when plants containing antidiabetic components are included in the daily diet.
Polyphenols are known to have antidiabetic activity, although their content has been reported to be only 27.1 ± 3.0 mg/g. In contrast, the lipid content in this beans is approximately 1%–1.5% [Citation13,Citation14], and there are a few reports on the bioactivity of active compounds contained. In our preliminary research, a lipophilic fraction was extracted using n-hexane, which was subjected to antioxidant activity and invitro α-glucosidase inhibition activity assays. The results showed that this fraction had moderate α-glucosidase inhibitory activity, but had no antioxidant activity. This result suggests that the n-hexane extract contains antidiabetic components different from polyphenols, which has already been reported in this beans [Citation15,Citation16]. Hence, the purpose of this study was to isolate the antidiabetic compounds from the hexane extract of red kidney beans and identify their structure.
Materials and methods
Red kidney beans were purchased from a local farmer in Malang, East Java, Indonesia. The damaged beans were sorted and removed in the farm. The good beans were dried in the farm, and then shipped to the laboratory. In the laboratory, the beans were further peeled, ground, and sifted using 100 mesh sieves to obtain flour. The flour was then stored in a refrigerator at -30°C prior to analysis.
Reagents and standards
For extraction and isolation, n-hexane and diethyl ether were purchased from Kanto Chemicals (Osaka, Japan) and acetic acid was purchased from Nakalai Tesque (Kyoto, Japan). Deuterochloroform (CDCl3, containing 0.03 wt% TMS) was purchased from Kanto Chemical (Osaka, Japan). Lipid standard, mono-, di-, and triglyceride mix (Sigma Aldrich, Tokyo, Japan) was used as the reference to isolate the triacylglycerol fraction. Sodium hydroxide (Nakalai Tesque), methanol (Kanto Chemicals) and n-hexane (FUJIFILM Wako Pure Chemical, Osaka, Japan) were used for preparing fatty acid methyl ester (FAME). The mobile phase used for HPLC and UPLC was the mixture of 2-prophanol (Nakalai Tesque), formic acid (FUJIFILM Wako Pure Chemical), and acetonitrile (Kishida Chemical, Osaka, Japan). For the α-glucosidase inhibitory activity assay, α-glucosidase from Saccharomyces cerevisiae was purchased from Sigma Aldrich (Tokyo, Japan). Acarbose hydrate, as the positive control, was obtained from Tokyo Chemical Industry (Tokyo, Japan). Phosphate buffer was prepared by mixing dipotassium hydrogen phosphate and potassium dihydrogen phosphate purchased from FUJIFILM Wako Pure Chemical. Sodium chloride and sodium carbonate were purchased from Nacalai Tesque.
Extraction of beans
Fifty grams of flour was extracted with 250 mL of n-hexane for 24 h at 25°C using Multi Shaker MMS (Tokyo Rikakikai Co., Ltd) at 150 rpm. The mixture was then filtered and evaporated using a rotary evaporator (Iwaki REN-1000). The n-hexane extract was stored at 0°C prior to analysis. This extraction process was carried out six times, and the average extraction yield was 0.84% ± 0.07%.
Silica gel open column chromatography
The hexane extract (0.4 g) was separated by silica gel open column chromatography (M-300H (Nagara Science, Gifu, Japan), 17 mm I.D. × 200 mm). The sample was eluted using n-hexane:diethyl ether:acetic acid at a ratio of 70:35:1 and fractionated into six fractions (Fig. S1).
Fraction 2 was dissolved in CDCl3 and analyzed by 1H-NMR. The 1H-NMR spectra were recorded on the JEOL ECA 600 spectrometer (JEOL, Tokyo, Japan) at 600 MHz and tetramethylsilane (TMS) was used as the internal standard. Thin layer chromatography (TLC) was performed using a standard mixture for validation by detecting identical retention factors (Rfs).
High pressure liquid chromatography (HPLC) conditions
Further separation of fraction 2 was performed by preparative HPLC using the JASCO PU2089 intelligent pump equipped with a JASCO UV-2075 detector (Tokyo, Japan) and Shimadzu CTO-10ACVP column oven (Kyoto, Japan). The COSMOSIL 5C18-MS-II column (10 mm I.D. × 250 mm, particle size of 5 μm) was used and the column temperature was maintained at 40°C. The sample injection volume was 150 µL and detection was carried out at 210 nm using a UV-vis detector. Separation was performed under isocratic conditions. The mobile phase consisted of 30% isopropanol and 0.1% formic acid in acetonitrile, at a flow rate of 5.0 mL/min. Five fractions (fr.) were obtained, namely, fr. A (22.13% ± 0.56%), fr. B (21.55% ± 0.30%), fr. C (27.02% ± 0.55%), fr. D (13.4% ± 0.16%), and fr. E (5.70% ± 0.18%).
Fatty acid methyl ester preparation
Each TAG fraction (fr. A-E, 2–4 mg) was diluted in n-hexane (200 μL), and then 2 M methanolic KOH (20 μL) was added. The mixture was vortexed for 2 min at 25°C. The FAME in the upper layer was analyzed by GC/MS.
Gas chromatography mass spectrometry (GC/MS) conditions
GC/MS analysis was performed using the Agilent 6890N gas chromatograph (Agilent Technologies, Santa Clara, CA, USA) fitted with a DB-5 column (30 m, 250 µm I.D., film thickness 0.25 µm; J&W Scientific, Santa Clara, California,, USA) coupled with a JMS-AMSUN200/GI UltraQuad GC-MS (JEOL Ltd., Tokyo, Japan). The carrier gas was helium at a flow rate of 1 mL/min. The injection sample volume was 1 μL under splitless mode. The GC temperature program was set as follows: initial temperature 50°C held for 3 min, and then increased to 150°C at 5°C/min and held for 3 min, a second increase at 2.5°C/min to 220°C held for 3 min, and a final increase at 25°C/min to 300 °C held for 2 min. The mass spectrometer was operated in the electron impact ionization (EI) mode at 70 eV with a detector voltage of 1.2 kV. The MS detector scanned from m/z 50 to 1000 at a scan rate of 2 scans/s. Both transfer line and ion source were held at 280°C. The MS spectrum of each peak (corresponding to a FAME) was identified using the library match software from the National Institute of Standards and Technology (NIST) library.
Ultra-high-performance liquid chromatography–time-of-flight mass spectrometry (UPLC–TOF-MS) conditions
The analysis was performed on an UPLC system coupled with a QTOF-MS (Waters Xevo G2 QTof, Waters, USA). The QTOF-MS was operated in the electrospray ionization (ESI) mode at the mass resolution of 20 000 and controlled using MassLynx 4.1 software. The collision energy (CE) was 6 V. High-CE in the range of 30–42 eV for MS/MS scans generated fragment ion information. The source parameters were set as follows: capillary voltage 2.5 kV, sampling cone 30 V, extraction cone 4 V, source temperature 150°C, desolvation temperature 500°C, desolvation gas flow 1000 L/h, and cone gas flow 50 L/h. The mass spectrometer was calibrated with 0.5 mM sodium formate. Leucine enkephalin (2 µg/mL, m/z 556.2771 in the positive mode) was used as lock spray at a flow rate of 10 µL/min. The chromatographic separation was performed at 35°C on the Acquity UPLC BEH C18 column (2.1 mm I.D. × 100 mm, 1.7 μm; Waters). The analysis was performed under isocratic condition. The mobile phase consisted of 30% isopropanol/0.1% formic acid in acetonitrile at a flow rate of 0.3 mL/min. The sample (1 μL) was injected using an auto sampler.
In vitro α-glucosidase inhibition activity
The α-glucosidase inhibitory activity of triacylglycerol (TAG) was assayed in vitro using a 96-well plate according to Watanabe et al. [Citation17] with some modifications. All reagents used were dissolved in phosphate buffer (50 mM, pH 6.9). The mixture, containing 20 μL of α-glucosidase (0.5 U/mL) and 50 μL of varying concentrations of each sample and the acarbose standard (0.1, 0.5, 1, 5, 10, 50, 100, 500, and 1000 ppm) were preincubated at 37°C for 15 min. Then, 50 μL of 4-nitrophenyl-α-D- glucopyranoside (PNPG) (9 mg/mL) was added as a substrate and the mixture was incubated further at 37°C for 15 min. The reaction was stopped by adding 100 μL of Na2CO3 (100 mM). The absorbance of the released p-nitrophenol was measured at 405 nm using Multiplate Reader Spark 10M (Tecan Group Ltd., Switzerland). Phosphate buffer was used as the control and each experiment was performed in triplicate. The results are expressed as percent inhibition and calculated using the formula: inhibitory activity (%) = (1 – (As/Ac) × 100; where, “As” is the absorbance in the presence of test substance and “Ac” is the absorbance of the control. The inhibitory activity was expressed by IC50, which is the concentration at which 50% of the α-glucosidase enzyme activity is inhibited. Statistical analysis was performed using the analysis of variance (ANOVA) and the least significant difference (LSD) test carried out at significance level of p < 0.05 to determine differences between the fractions.
Results and discussion
Red kidney beans are generally consumed in the form of flour in Indonesia; the seed coat is usually removed during the milling process. This bean has red seed coat, indicating the presence of polyphenols, as pigments. In fact, the polyphenols quercetin glycosides and kaempferol glycosides are reported to be present in red kidney beans. In general, polyphenols have been known to have various bioactivities including antidiabetic activity. Hence, to focus on the functional components, except polyphenol, peeled bean samples were used in this study.
In our previous study, peeled beans were extracted using methanol, ethanol, and hexane. The percentage of hexane extract yield was relatively low (0.84% ± 0.07%); the yield of other extracts was also similarly low, below 1% each (data not shown). Red kidney beans contain a high percentage of carbohydrates and proteins [Citation1] which have a low solubility in these organic solvents. The low extract yields were presumed to be due to a relatively low content of other components. The yield of methanol extract was higher than that of hexane extract, and probably contained some polyphenols [Citation6]. On the contrary, hexane extract exhibited moderate α-glucosidase inhibitory activity although it had no DPPH scavenging activity (data not shown). Generally, it is known that phenolic compounds show a high radical-scavenging activity [Citation18,Citation19]; this result suggested that hexane extract might contain antidiabetic component, besides polyphenols. These data showed an important and interesting aspect of hexane extract for further investigation. Hence, we explored the functional components in hexane extract.
Separation of hexane extract
The hexane extract was separated by open column chromatography, which produced six fractions (Fig. S1). The largest fraction was fr. 2, accounting for 76.69% of the extract and was examined further. The 1H NMR analysis of fr. 2 was performed (Fig. S2). The 1H NMR spectra showed the presence of signals attributable to the methyl groups at δH 0.9 ppm, methylene signals at δH 1.3 ppm, methine proton signals at δH 1.70 ppm, and acyl protons at δH 2.0–2.8 ppm. These data and the polarity of the sample suggested that a fatty acid molecule was present in this fraction. In addition, the characteristic geminal proton signals at δH 4.1–4.2 ppm suggested the presence of a glycerol group attached to an ester group in this molecule. Based on these results, the major component of the hexane extract was assumed to be TAG. The chemical shift at δH 5.3–5.4 ppm indicates characteristic peaks of vinyl protons and shows that unsaturated fatty acids probably constitute the fatty acid component. Thin layer chromatography using the lipid standard mixture for comparison also revealed the presence of TAG in this fraction.
This fraction was then separated by preparative HPLC and the five largest peaks (A to E) were obtained (Fig. S1). Based on the peak shape, peaks A and B were presumed to be attributable to one compound each, whereas peaks C, D, and E were attributable to multiple components (Fig. S3).
Fatty acid composition of the TAG fraction
As previously stated, the main component in the hexane extract was presumed to be TAG. The fatty acid composition of fr. A to E was identified by GC-MS. Each fraction was derivatized into FAME prior to the analysis. lists the fatty acids found in fr. A-E. α-Linolenic acid (ω-3) and linoleic acid (ω-6) were found to be the major components. This was similar to the results obtained by other researchers who determined the fatty acid composition of this beans [Citation20–Citation23]. α-Linolenic acid and linoleic acid content in kidney beans has been reported to be approximately 46.0% and 21.0%, respectively [Citation24].
Table 1. Fatty acid composition and unsaturation ratio in each fraction from GC/MS analysis.
Although fatty acids in each fraction were identified, this information was not sufficient to determine the structure of TAGs, as the binding position of each fatty acid should also be determined. To elucidate the structure of TAGs in these fractions, further analysis by UPLC-MS was performed to determine the binding position of fatty acids in each TAG.
Identification of triacylglycerol structures
To identify TAGs, determination of the regioisomeric position of fatty acids in TAG is important. Hence, fr. A-E were subjected to UPLC-ESI-MS and MS/MS analyzes. Pseudo molecular ions of [TAG+H]+, [TAG+H2O+H]+, [TAG+Na]+, and [TAG+K]+ were observed as shown in . The number of pseudo molecular ions in each fraction indicated the number of TAGs in each fraction, namely, fr. A and B had one compound each (TAGs 1 and 2), fr. C and D had two compounds each (TAGs 3, 4 and 5, 6), and fr. E had four components (TAGs 7–10).
Table 2. Mass number of parent peak and fragment peaks (diacylglycerol and acylium ion) of triacylglycerols (TAGs).
Diacylglycerol (DAG) ion fragments ([DAG+H]+) and acylium ion fragments ([RCO]+, [RCO+74]+, [RCO+74-H2O]+, and [RCO-H2O]+) were obtained by MS/MS. DAG fragment ions with enough intensity were observed. The proposed fragmentation mechanism of TAG under ESI-MS-MS condition is shown in . The TAG fragmentation process generating DAG fragments produces a neutral loss corresponding to the fatty acid chain. shows the presumed structures of DAG fragments and fatty acid loss according to mass number obtained by confirming the fatty acids identified by GC-MS and matching with that in the database of fatty acid and triacylglycerol [Citation25,Citation26] . The binding positions of each fatty acid on glycerol were determined based on the combination of the presumed chemical structures of DAG and fatty acid.
Table 3. Neutral loss and fatty acid loss determined for each diacylglycerol detected.
In TAG 1 of fr. A, [DAG+H]+ ion at m/z 595.4493 was observed and no appreciable other corresponding fragment peak was present. This result suggested that fragment ion peaks for DAG1,2(2,3) and DAG1,3 had the same mass number. The neutral loss of 278.2218 corresponded to linolenic acid (Ln). Acylium ion fragments corresponding to Ln were also observed. Thus, TAG 1 was identified as LnLnLn (,), ). This result was in good agreement with the GC-MS results that only observed Ln ().
Figure 2. Positive ion mass spectra of fractions A and B. Fraction A contained TAG 1 with spectrum of MS (a) and MS-MS of m/z 873 (b), and fraction B contained TAG 2 with spectrum of MS (c) and MS-MS of m/z 875 (d).
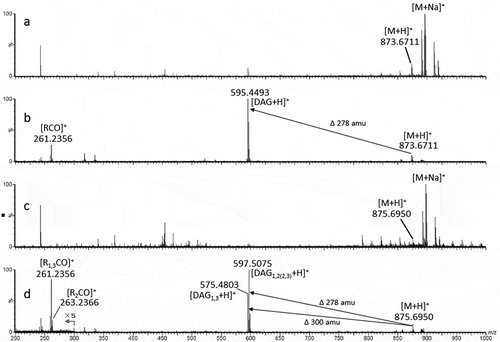
In TAG 2 of fr. B, [DAG+H]+ ion peaks at m/z 597.5075 (loss of 278.1875) and 595.4803 (loss of 280.2147) were observed (). The neutral losses corresponded to linoleic acid (L) and Ln. TAG fragmentation into DAG occurred more easily in the sn-1 and sn-3 positions; therefore, the fragment ion reflecting the sn-1 and sn-3 positions may be more abundant than the ion reflecting the sn-2 position [Citation27,Citation28]. Because the relative abundance of peak m/z 597.5075 was higher than that of m/z 595.4803, the former ion peak is assumed to be [DAG1,2(2,3)+H]+ and the latter to be [DAG1,3 + H]+ (). This result suggested that Ln was located at the sn-1 or sn-3 position and L at the sn-2 position. Thus, the TAG 2 structure was identified as LnLLn (,), ). This was also supported by the same fatty acid data in fr. B from GC-MS (), confirming our identification of the fatty acid constituents of TAG 2.
In TAG 5 of fr. D, [DAG+H]+ with m/z 599.5227 allowed either a combination of Ln and oleic acid (O) or Ln and elaidic acid (El) or two units of L as possible constituent fatty acids. However, El is a fatty acid with a trans structure that is typically found in bovine milk and meat, but not in legumes, such as red kidney beans. Furthermore, it was known that fatty acids identified as neutral loss from the three DAG fragments were L, Ln and O. Therefore, the TAG 5 structure was identified as LLnO.
Other TAGs were determined in the same way and all of them validated that GC-MS and LC-MS provide mutually supportive data to be used in determining the composition of TAGs ().
Table 4. Summary of TAG molecular species in the triacylglycerol fraction and IC50 of α-glucosidase inhibition activity.
The main TAGs were LnLnLn (trilinolenin) and LnLLn (1,3-dilinolenoyl-2-linoleoyl glycerol), considered as peaks A and B in the chromatogram with areas of 22.13% and 21.55%, respectively, and both composed of only one TAG. These results were slightly different from those obtained in previous studies, where LLLn was identified as the main TAG [Citation20] besides the two TAGs identified in our study (LnLnLn and LnLLn). This could occur because of the different cultivars used or other external factors, such as planting location, temperature, and soil nutrition.
In vitro α-glucosidase inhibition activity
The potency of fr. A-E as antidiabetic compounds was analyzed by studying their ability to inhibit α-glucosidase activity in vitro. The IC50 values of each fraction are shown in . Although all the fractions had inhibitory activity, fr. A showed the highest activity compared with that of the others, while fr. E had the lowest activity.
Inhibition of α-glucosidase activity was related to the type and number of TAGs contained within each fraction. The inhibitory activities of TAGs were strongly influenced by fatty acid composition, where the inhibitor activity decreased with decreasing number of double bonds (). Fr. A and B each contained just one type of TAG, namely LnLnLn and LnLLn, and both are polyunsaturated fatty acids (), leading to high inhibitory activities compared with those of other fractions. Previous study that explored the ability of fatty acids to act as antidiabetics using experimental animal models and rat insulinoma cell lines suggested better inhibitory activity with polyunsaturated fatty acids than saturated fatty acids, suggesting they could prevent diabetes and attenuate oxidant stress that may occur in diabetes mellitus [Citation29,Citation30]. Our results support these studies from earlier groups, suggesting that TAGs containing polyunsaturated fatty acids have stronger antidiabetic potential due to their ability to inhibit α-glucosidase activity.
The highest inhibition of α-glucosidase activity was observed with fr. A and B, whose TAG compositions differed in the fatty acids occupying the second position. The presence of L in the sn-2 position of fr. B, instead of Ln, caused a slight decrease in the inhibitory activity. Although both are unsaturated fatty acids, the number of double bonds in the structure of the TAGs was thought to contribute to their ability to inhibit α-glucosidase activity. Four types of TAGs, namely LOL, LPL, LnOP, and PLnP contained in fr. E, among the constituent fatty acids, L, Ln, and O are unsaturated fatty acids, while P is a saturated fatty acid. The presence of saturated fatty acids explained the decrease in α-glucosidase inhibition by the TAGs in certain fractions. The ratio of unsaturated fatty acids decreased from fr. C through E (). Although unsaturation ratio at fr. D of 3.73 only decreased to 2.84 in fr. E, the IC50 values were considerably different (). This may suggest that the positions of the fatty acids in the TAG structures are also thought to affect the ability of TAGs to inhibit the α-glucosidase activity.
Conclusively, we found that the inhibitory activities of fr. A through D were higher than those of the positive control acarbose, suggesting that the TAGs in red kidney beans, which contain Ln and L as the major fatty acids, could act as strong potential antidiabetic agents.
Conclusions
TAGs were the dominant lipid components found in red kidney beans. The main TAGs found were LnLnLn (trilinolenin) and LnLLn, while the dominant fatty acids were α-linolenic acid (ω-3) and linoleic acid (ω-6). The combination of GC-MS and UPLC-MS is well suited for determining TAG structures, while the relative intensity between the DAG peaks enables the determination of the isomer. The antidiabetic activity, measured via in vitro inhibition of α-glucosidase activity, was comparable to that of the positive control acarbose. Although the amount of each TAG in these beans is relatively low, daily consumption may provide an antidiabetic benefit, potentially via synergistic effects between the TAGs and polyphenols contained in red kidney beans, which are already known to possess antidiabetic activity. Thus, these beans may become one of the functional daily foods appropriate for diabetes therapy and can support the community’s efforts toward diabetes prevention. In addition, our results suggested that positions of the fatty acids in the TAG structure affects its activity in inhibiting α-glucosidase. On the contrary, the presence of TAG in the small intestine has opportunity to be hydrolyzed by lipase into fatty acids. Therefore, in vivo evaluation of this antidiabetic activity is required to ascertain the potential of red kidney beans as an antidiabetic agent. Interactions between TAGs and other food components should also be studied further to determine if there are any synergistic effects.
Author contribution
A.M.S., E.Y., I.B., D.F. and H.N.L. conceived and designed the experiments. A.M.S. performed the experiments. E.Y. supervised the experimental techniques. A.M.S. and E.Y. wrote the paper. I.B., D.F. and H.N.L. reviewed manuscript. All authors have read and approved the submitted manuscript.
supporting_material.docx
Download MS Word (456.9 KB)Acknowledgments
We thank Dr. Tohru Mitsunaga (Professor at Gifu University) and Dr. Shingo Kawai (Professor at Shizuoka University) for useful discussions.
Disclosure statement
No potential conflict of interest was reported by the authors.
Supplementary material
Supplemental data for this article can be accessed here.
References
- Wang N, Hatcher DW, Tyler RT, et al. Effect of cooking on the composition of beans (Phaseolus vulgaris L.) and chickpeas (Cicer arietinum L.). Food Res Int. 2010;43(2):589–594.
- Ibeabuchi JC, Okafor DC, Peter–Ikechukwu A, et al. Comparative study on the proximate composition, functional and sensory properties of three varieties of beans Phaseolus lunatus, Phaseolus vulgaris and Vigna umbellata. Int J Adv Eng Technol Manag Appl Sci. 2017;05(01):1–23.
- Chaudhary R, Sharma S. Conventional nutrients and antioxidants in red kidney beans (Phaseolus vulgaris L.): an explorative and product development endeavour. Ann Food Sci Technol. 2013;14(2):275–285.
- Dueñas M, Martínez-Villaluenga C, Limón RI, et al. Effect of germination and elicitation on phenolic composition and bioactivity of kidney beans. Food Res Int. 2015;70:55–63.
- Parmar N, Singh N, Kaur A, et al. Effect of canning on color, protein and phenolic profile of grains from kidney bean, field pea and chickpea. Food Res Int. 2016;89:504–513.
- Lin L-Z, Harnly JM, Pastor-Corrales MS, et al. The polyphenolic profiles of common bean (Phaseolus vulgaris L.). Food Chem. 2008;107(1):399–410.
- Oomah BD, Cardador-Martínez A, Loarca-Piña G. Phenolics and antioxidative activities in common beans (Phaseolus vulgaris L). J Sci Food Agric. 2005;85(6):935–942.
- Ganesan K, Xu B. Polyphenol-rich dry common beans (Phaseolus vulgaris L.) and their health benefits. Int J Mol Sci. 2017;18:2331.
- Atchibri -ALO-A, Brou KD, Kouakou TH, et al. Screening for antidiabetic activity and phytochemical constituents of common bean (Phaseolus vulgaris L.) seeds. J Med Plants Res. 2010;4(17):1757–1761.
- Pascale R, Bianco G, Cataldi TRI, et al. Mass spectrometry-based phytochemical screening for hypoglycemic activity of Fagioli di Sarconi beans (Phaseolus vulgaris L.). Food Chem. 2018;242:497–504.
- Bianco G, Pascale R, Carbone CF, et al. Determination of soyasaponins in Fagioli di Sarconi beans (Phaseolus vulgaris L.) by LC-ESI-FTICR-MS and evaluation of their hypoglycemic activity. Anal Bioanal Chem. 2018;410(5):1561–1569.
- International Diabetes Federation. IDF-diabetic atlas. 8th ed. Karuranga S, Fernandes J, Huang R, et al., editors. International Diabetes Federation, Brussel, Belgium; 2017. p. 16–17. Available from https://www.idf.org/e-library/epidemiology-research/diabetes-atlas.html
- Zhao Y, Du SK, Wang H, et al. In vitro antioxidant activity of extracts from common legumes. Food Chem. 2014;152:462–466.
- Marathe SA, Deshpande R, Khamesra A, et al. Effect of radiation processing on nutritional, functional, sensory and antioxidant properties of red kidney beans. Radiat Phys Chem. 2016;125:1–8.
- Mojica L, Berhow M, de Mejia EG. Black bean anthocyanin-rich extracts as food colorants: physicochemical stability and antidiabetes potential. Food Chem. 2017;229:628–639.
- Mojica L, Meyer A, Berhow MA, et al. Bean cultivars (Phaseolus vulgaris L.) have similar high antioxidant capacity, in vitro inhibition of α-amylase and α-glucosidase while diverse phenolic composition and concentration. Food Res Int. 2015;69:38–48.
- Watanabe J, Kawabata J, Kurihara H, et al. Isolation and identification of α -glucosidase inhibitors from tochu-cha (Eucommia ulmoides). Biosci Biotechnol Biochem. 1997;61(1):177–178.
- Wang YK, Zhang X, Chen GL, et al. Antioxidant property and their free, soluble conjugate and insoluble-bound phenolic contents in selected beans. J Funct Foods. 2016;24:359–372.
- Mastura YH, Hasnah H, Dang TN. Total phenolic content and antioxidant capacity of beans: organic vs inorganic. Int Food Res J. 2017;24(2):510–517.
- Caprioli G, Giusti F, Ballini R, et al. Lipid nutritional value of legumes: evaluation of different extraction methods and determination of fatty acid composition. Food Chem. 2016;192:965–971.
- Grela ER, Günter KD. Fatty acid composition and tocopherol content of some legume seeds. Anim Feed Sci Technol. 1995;52(3–4):325–331.
- Sasaki S, Ohnishi M, Mano Y, et al. Characterization of triacylglycerol species in oil-poor beans. Agric Biol Chem. 1989;53(7):2021–2023.
- Yoshida H, Tomiyama Y, Kita S, et al. Lipid classes, fatty acid composition and triacylglycerol molecular species of kidney beans (Phaseolus vulgaris L.). Eur J Lipid Sci Technol. 2005;107(5):307–315.
- Sutivisedsak N, Moser BR, Sharma BK, et al. Physical properties and fatty acid profiles of oils from black, kidney, great northern, and pinto beans. JAOCS, J Am Oil Chem Soc. 2011;88(2):193–200.
- Byrdwell WC. Fatty acids and related masses . [Internet]. 2018 cited 2018Dec18. p. 1–2. Available from: http://www.byrdwell.com/Triacylglycerols/FattyAcids.htm
- Byrdwell WC. Triacylglycerols by Mass. [Internet]. 2005. cited 2018 Dec 18. p. 50–53. Available from: http://www.byrdwell.com/Triacylglycerols/TAGbyMass2.htm
- Hsu F, Turk J. Structural characterization of triacylglycerols as lithiated adducts by electrospray energy collisionally activated dissociation on a triple stage quadrupole instrument. J Am Soc Mass Spectrom. 1999;10(7):587–599.
- Hvattum E. Analysis of triacylglycerols with non-aqueous reversed-phase liquid chromatography and positive ion electrospray tandem mass spectrometry. Rapid Commun Mass Spectrom. 2001 Feb 15;15(3):187–190.
- Suresh Y, Das UN. Long-chain polyunsaturated fatty acids and chemically induced diabetes mellitus: effect of ω-3 fatty acids. Nutrition. 2003;19(2):213–228.
- Mohan IK, Das UN. Prevention of chemically induced diabetes mellitus in experimental animals by polyunsaturated fatty acids. Nutrition. 2001;17(2):126–151.